DOI:
10.1039/D2SC03156F
(Edge Article)
Chem. Sci., 2022,
13, 10048-10056
Subcomponent self-assembly of circular helical Dy6(L)6 and bipyramid Dy12(L)8 architectures directed via second-order template effects†
Received
3rd June 2022
, Accepted 20th July 2022
First published on 22nd July 2022
Abstract
In situ metal-templated (hydrazone) condensation also called subcomponent self-assembly of 4,6-dihydrazino-pyrimidine, o-vanillin and dysprosium ions resulted in the formation of discrete hexa- or dodecanuclear metallosupramolecular Dy6(L)6 or Dy12(L)8 aggregates resulting from second-order template effects of the base and the lanthanide counterions used in these processes. XRD analysis revealed unique circular helical or tetragonal bipyramid architectures in which the bis(hydrazone) ligand L adopts different conformations and shows remarkable differences in its mode of metal coordination. While a molecule of trimethylamine acts as a secondary template that fills the void of the Dy6(L)6 assembly, sodium ions take on this role for the formation of heterobimetallic Dy12(L)8 by occupying vacant coordination sites, thus demonstrating that these processes can be steered in different directions upon subtle changes of reaction conditions. Furthermore, Dy6(L)6 shows an interesting spin-relaxation energy barrier of 435 K, which is amongst the largest values within multinuclear lanthanide single-molecular magnets.
Introduction
Oligonuclear metallosupramolecular complexes of lanthanides have attracted quite some interest over the last two decades mainly due to their astonishing photophysical and magnetic properties.1 Nevertheless, their supramolecular chemistry is much less developed than that of d-block metals1a,b,2,3 as their coordination chemistry is more challenging to control – their generally large coordination numbers and geometries are much less defined, and since their coordination chemistry is mainly governed by electrostatic interactions they neither show very distinct preferences for donor atoms such as nitrogen or oxygen; other than that, they should be rather hard Lewis-basic sites nor do classical supramolecular design principles such as the maximum site occupancy rule4 apply to them.1,5 Therefore, the use of ligand motifs that offer a high density of potential donor atoms has proven to be a successful approach to access metallosupramolecular aggregates of lanthanides via coordination-driven self-assembly processes although their outcome is still hard to predict.1,6
The subcomponent self-assembly approach7 that involves reversible in situ metal-templated condensation reactions to form imine or hydrazone bonds has been established as a particularly powerful tool for generating metallosupramolecular architectures of increasing structural complexity with various properties and features,8 like for example, astonishing host–guest behavior,9 complex-to-complex transformations10 or switchable systems.10a,11 Moreover, subcomponent self-assembly also provides space for serendipity to form new structures, and thereby, discover new interesting general structural motifs and/or metal binding motifs which might not even necessarily make use of all subcomponents in the initially expected relative ratio. Thus, we regard it as an especially versatile approach to prepare oligonuclear metallosupramolecular aggregates with densely functionalized multifunctional metal binding motifs essential to address the specific needs of lanthanide coordination.
Following our interest in the magnetic properties of oligonuclear lanthanide complexes,12,13 we decided to also apply this approach. Herein, we report on the use of o-vanillin and 4,6-dihydrazinopyrimidine as subcomponents that undergo twofold metal-templated hydrazone condensation and metal coordination upon mixing with lanthanide salts and a suitable base giving rise to rather surprising cyclic helical hexanuclear complexes or a dodecanuclear tetragonal bipyramid complex as a result of secondary template effects. With this study we extend our previous work that demonstrated the validity of the flexible dianionic ligand L (Scheme 1), that results from the metal-templated twofold hydrazone formation of the organic subcomponents, in the construction of polynuclear lanthanide SMMs with desired topology and magnetic properties.14
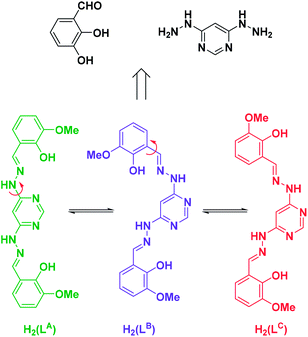 |
| Scheme 1 Ligand L derived from o-vanillin and 4,6-dihydrazinopyrimidine as its neutral form H2(L) in three different conformations H2(LA), H2(LB) and H2(LC) relevant for this study. Red arrows indicate successive rotations around single bonds to convert the different conformers into each other. The different conformations give rise to different coordination modes as shown in Fig. 1 and 2. | |
Experimental
General synthetic considerations
All starting materials were of A.R. Grade and were used as commercially obtained without further purification. 4,6-Dihydrazinopyrimidine was prepared according to a previously published method.15 Elemental analyses for C, H, and N were carried out on a PerkinElmer 2400 analyzer. Fourier transform IR (FTIR) spectra were recorded with a PerkinElmer FTIR spectrophotometer using the reflectance technique (4000–300 cm−1). The samples were prepared as KBr pellets. High-resolution mass spectra were acquired using a Thermo Scientific X series.
Synthesis of [Dy6LA2LC4(CH3OH)7(H2O)5(NO3)4]·NEt3·10CH3OH·11H2O·2NO3 (Dy6L6)
4,6-Dihydrazinopyrimidine (0.1 mmol) was dissolved in 15 mL methanol, and then o-vanillin (0.2 mmol) and NEt3 (0.2 mmol) were added successively. Finally, Dy(NO3)3·6H2O (0.1 mmol) was added to the mixture. The reaction mixture was stirred at room temperature for 4 h and the resultant solution was filtered and left unperturbed to allow for slow evaporation of the solvent. Dark yellow single crystals of complex Dy6L6 were obtained after one week. Yield: 25 mg, (31.95%, based on the metal salt). Elemental analysis (%) calcd for C143Dy6N43O75H198: C, 36.59, H, 4.25, N, 12.83; found C, 36.52, H, 4.29, N, 12.78.
Synthesis of [Dy12Na4LA4LB4(CH3COO)12(C8H7O)4(CO3)4 (CH3CN)2(H2O)4]·6H2O (Dy12L8)
4,6-Dihydrazinopyrimidine (0.1 mmol) was dissolved in methanol/acetonitrile (5 mL
:
10 mL), and then o-vanillin (0.2 mmol) and NaHCO3 (0.1 mmol) were added successively. Finally, Dy(OAc)3·2H2O (0.1 mmol) was added to the mixture. The reaction mixture was stirred at room temperature for 5 h and the resultant solution was filtered and left unperturbed to allow for slow evaporation of the solvent. Dark yellow single crystals of complex Dy12L8 were obtained after one week. Yield: 25 mg, (41.89%, based on the DyIII salt). Elemental analysis (%) calcd for C224H222Dy12N50Na4O94: C, 20.46, H, 3.12, N, 9.78; found C, 20.39, H, 3.18, N, 9.84.
X-ray crystal structure determinations
Crystallographic data and refinement details are given in Table S2.† Single crystals of suitable dimensions of Dy6(L)6 and Dy12(L)8 were selected for single-crystal X-ray diffraction analysis. Crystallographic data were collected at 100(5) K on a Bruker Apex II CCD diffractometer with graphite monochromated Mo-Kα radiation (λ = 0.71073 Å). The data were integrated using the Siemens SAINT program. Absorption corrections were applied. The structures were solved by direct methods and refined by the full-matrix least-squares method based on F2 with anisotropic thermal parameters for all non-hydrogen atoms by using the SHELXS (direct methods) and refined by SHELXL (full matrix least-squares techniques) in the Olex2 package.16 The locations of Dy and Na atoms were easily determined, and O, N, and C atoms were subsequently determined from the different Fourier maps. Anisotropic thermal parameters were assigned to all non-hydrogen atoms. The H atoms were introduced in the calculated positions and refined with a fixed geometry with respect to their carrier atoms. CCDC 1507720 (Dy6(L)6) and 1536333 (Dy12(L)8) contain the supplementary crystallographic data for this paper.†
Magnetic measurements
Magnetic susceptibility measurements were recorded on a Quantum Design MPMS-XL7 SQUID magnetometer equipped with a 7 T magnet. The variable-temperature magnetizations were measured in the temperature range of 1.9–300 K with an external magnetic field of 1000 Oe. The dynamics of magnetization were investigated from the ac susceptibility measurements in the zero static fields and a 3.0 Oe ac oscillating field. Diamagnetic corrections were made with Pascal's constants17 for all the constituent atoms as well as the contributions of the sample holder.
Results and discussion
Structural analysis
Here, we dissolved 4,6-dihydrazinopyrimidine, o-vanillin and NEt3 (ref. 18) in methanol successively, and then added one equivalent of Dy(NO3)3 to the mixture.19 The initially light yellow solution slowly turned dark yellow and dark yellow crystals were obtained after one week. The crystals were macroscopically examined by optical microscopy and scanning electron microscopy (SEM), revealing their hexagonal shape (see the ESI†). ESI mass spectrometric investigations of the reaction mixtures as well as of the samples obtained upon dissolving the crystalline product revealed a Dy6(L)6 stoichiometry of the resulting products (see the ESI†). This assumption could finally be proven by X-ray diffraction analysis of the single crystals of the hexanuclear dysprosium(III) complex. The result of the analysis is shown in Fig. 1 and it reveals the rather complicated and unique structure of the NEt3@[Dy6(L)6(CH3OH)7 (H2O)5(NO3)4](NO3)2 unit of this metallosupramolecular architecture. The six DyIII ions adopt a distorted hexagonal arrangement with an average distance of 8.3 Å between them (the corresponding angles in this Dy6 hexagon and the coordination geometries of the DyIII ions are listed in the ESI†).
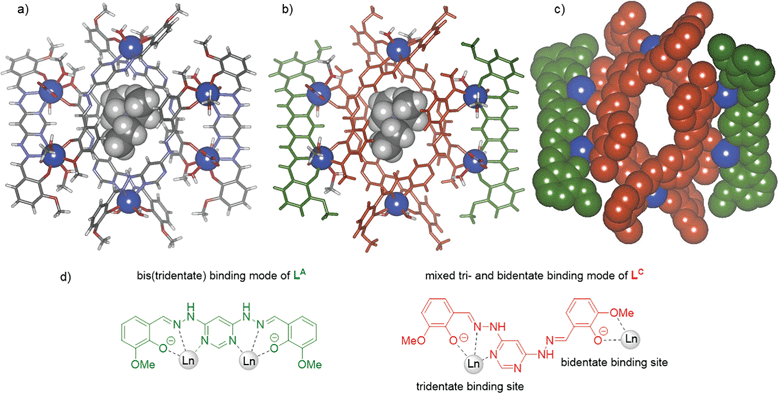 |
| Fig. 1 (a) Structure of the cationic unit of [Dy6(LA)2(LC)4(CH3OH)7(H2O)5(NO3)4](NO3)2 as determined by X-ray diffraction analysis: (a) wire-and-sphere representation of the supramolecular aggregate where the NEt3 molecule occupying the central cavity is shown as a space-filling model (color code: C gray, H white, N light blue, O red, and Dy dark blue); (b) the same representation where ligands LA and LC have been colored in green and red as in Scheme 1, respectively; (c) space filling representation (coordinating nitrate ions and water and methanol molecules as well as the encapsulated molecule of trimethylamine are omitted, colour code as in (b)); (d) representation of the different binding modes of ligands LA and LC in this assembly. | |
Each DyIII center is coordinated by two metal binding motifs from two dianionic ligands L (Fig. 1d and the ESI†) and the remaining coordination sites are occupied by counter ions or solvent molecules. The ligands L, however, do not all have the same conformation, and therefore, do not bind in the same binding mode to all of the DyIII centers. While two of these ligands adopt conformation A (LA) acting as a bis(tridentate) ligand bridging two adjacent metal centers in the periphery of the cyclic hexanuclear aggregate (see the green ligands in Fig. 1b), the other four adopt conformation C (LC) which is why the structure could more precisely be described as a [Dy6(LA)2(LC)4(CH3OH)7(H2O)5(NO3)4]2+ unit (see the red ligands in Fig. 1b). As such, ligands LC bridge the metal centers in a mixed tri- and bidentate binding mode in a grid like fashion leading to an overall helically interwoven architecture as illustrated in Fig. 1c (see the ESI† for other representations). Thus, two opposite DyIII centers are coordinated by the tridentate binding sites of two ligands LC whereas the other four are coordinated by one tridentate binding site of a ligand LA and the bidentate binding motif of a ligand LC.
It is noteworthy that many lanthanide-based double-,5 triple-20 and quadruple-stranded21 helicates assembled using flexible ligands have been reported, which generally display ordinary linear topology, while for lanthanide-based circular helicates, only very few examples have been reported to date, including triangular,22 square23 and hexagonal21,24 diverse topologies.1q Therefore, the circular helicate Dy6(L)6 indeed belongs to the rather rare species considering that the bridging ligand L adopts different conformations (LA and LC, Fig. 1 and the ESI†).
Due to this arrangement, the aggregate contains a rather hydrophobic cavity which is occupied by a molecule of triethylamine which perfectly fills this void. Such (secondary) template effects are rather common in the formation of cyclic or cage-like self-assembled structures whose components can also assemble into other spatial arrangements in the absence of a fitting template.2g,8a–c,25 Hence, we assume that triethylamine and basic molecules of similar shape26 act as secondary templates for the formation of this aesthetically appealing supramolecular aggregate.
In order to test this and the influence of the counter ion further, we also investigated a subcomponent self-assembly approach using Dy(OAc)3 and NaHCO3 (ref. 18) as the base. Again, we were able to obtain the product of this approach in the form of yellow circular bipyramid-shaped single crystals (see the ESI†) already indicating that another metallosupramolecular aggregate has been formed. Fortunately, the quality of the crystals was good enough to perform an XRD analysis that revealed yet another unique complex hexadecanuclear heterobimetallic architecture ([Dy12Na4(L)8(CH3COO)12(C8H7O)4(CO3)4(CH3CN)2(H2O)4]·6H2O) (Fig. 2). The asymmetric unit of the structure contains three DyIII ions and one NaI ion with one of the DyIII centers being eight-coordinated whereas the other two are nine-coordinated (see the ESI†). The whole aggregate can be divided into three parts: two identical Dy4(L)2 units featuring a dense arrangement of four DyIII centers that are bridged by carbonates, acetates and solvate molecules where the DyIII centers also bind in pairs to two ligands in conformation B (LB, shown in purple in Fig. 2b) in a mixed tri- and bidentate fashion, as depicted in Fig. 2c (the other coordination sites of the DyIII ions of these units are occupied by acetates). The third unit is a grid-like Dy4(L)4 unit where each DyIII center coordinates to tridentate binding sites of two ligands in conformation A (LA, shown in green in Fig. 2b). Interestingly, not all organic subcomponents assemble to ligand L here but the remaining coordination sites of each DyIII ion are occupied by one molecule of o-vanillin (light blue in Fig. 2b) and a water molecule. These three units are held together by four sodium ions that coordinate as secondary templates to the remaining binding sites of the ligands LA and LB each in a chelating manner, as depicted in Fig. 2c. This results in an overall tetragonal bipyramid ([Dy12Na4(LA)4(LB)4(CH3COO)12(C8H7O)4(CO3)4(CH3CN)2 (H2O)4]·6H2O) architecture in which the two Dy4(LB)2 units form a Na4Dy8(LB)4 cage that embeds the grid-like Dy4(LA)4 unit in its center (for additional representations of this unique assembly see the ESI†).
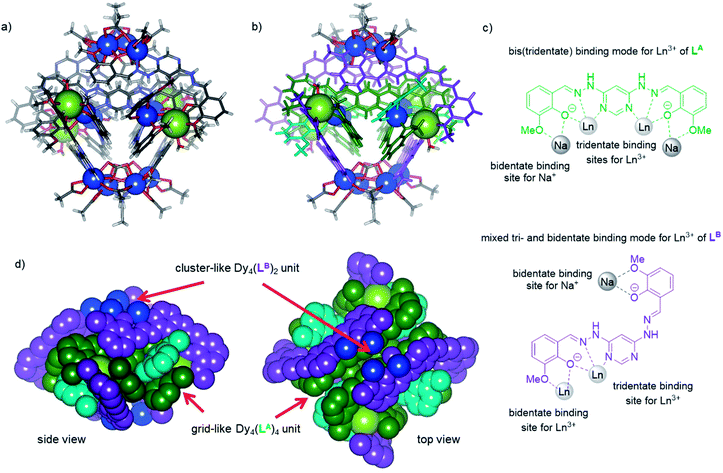 |
| Fig. 2 (a–d) Structure of the ([Dy12Na4(LA)4(LB)4(CH3COO)12(C8H7O)4(CO3)4(CH3CN)2 (H2O)4] unit as determined by X-ray diffraction analysis: (a) wire-and- sphere representation of the supramolecular aggregate (color code: C gray, H white, N light blue, O red, Dy dark blue, and Na yellow-green); (b) the same representation where ligands LA and LB and the o-vanillin molecules have been colored in green, purple and light blue, respectively; (c) representation of the different binding modes of ligands LA and LB in this assembly; (d) space filling representation from two perspectives (coordinating acetate and carbonate ions and acetonitrile and water are omitted, colour code as in (b)). | |
Both discrete metallosupramolecular aggregates belong to the rather rare species in which a bridging ligand adopts more than one conformation.25h,27 However, in these two new examples the ligands also differ significantly in their coordination behavior with regard to the binding sites for the metal ions and their denticity. This is special and a feature of metallosupramolecular assemblies of lanthanide ions because they tend to fill vacant coordination sites with counter ions and solvate molecules as capping or bridging ligands much more easily than transition metal ions due to the by far predominant electrostatic nature of the lanthanides' coordinative interactions with their ligands. That way, both assemblies achieve a favorable filling of space as well as a mutual orientation of ligands LA and LB to ensure π–π-interactions in Dy12(L)8 which most likely provide the driving forces for their formation.
Magnetic properties
Having elucidated the unexpected new structural motifs of the hexa- and dodecanuclear metallosupramolecular Dy6(L)6 and Dy12(L)8 assemblies, we wanted to see how this reflects in the complex properties. Therefore, we turned to explore their magnetic behavior and their ability to act as a lanthanide single-molecule magnet.1c,e,g,k,m,n,28 The variable-temperature χMT products (Fig. 3 and the ESI†) for Dy6(L)6 and Dy12(L)8 show similar trends with room-temperature χMT values of 83.98 and 168.98 cm3 K mol−1 corresponding to the expected values for uncoupled DyIII6 and DyIII12 moieties (6H15/2, S = 5/2, L = 5, J = 15/2, g = 4/3). Upon lowering the temperature, the χMT values are weakly temperature dependent in both cases until below 70 K where they start to gradually fall, reaching values of 77.90 and 154.88 cm3 K mol−1 at 50 K, after which they precipitously decrease to reach 68.17 and 130.75 cm3 K mol−1 at 2.0 K.
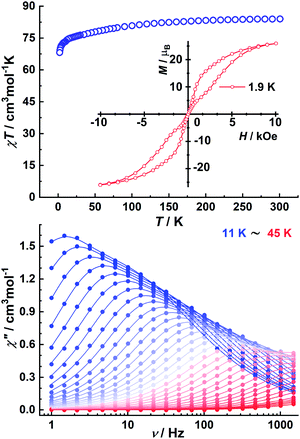 |
| Fig. 3
χT vs. T plot recorded on Dy6(L)6 in an applied field of 1 kOe. Inset: molar magnetization at 1.9 K (top) and frequency dependence of the χ′′ of Dy6(L)6 between 11 and 45 K (bottom). The solid lines represent the fitting of the experimental data using the sum of two modified Debye functions. | |
The decrease observed in the χMT values suggests the magnetic anisotropy/ligand field and thermal depopulation of the excited MJ states, but we cannot preclude the presence of weak antiferromagnetic interactions in view of these poly-nuclear systems. Furthermore, the field dependence of the magnetization measurements shows a rapid increase at low fields and eventually reaches values of about 29.31 μB for Dy6(L)6 and 62.83 μB for Dy12(L)8 without clear saturation up to 70 kOe below 1.9 K, respectively (Fig. 3 and the ESI†). Additionally, in contrast to the non-superposition of the M vs. H/T data on to a single master-curve for Dy12(L)8, the nearly overlapping M versus H/T curves at different temperatures (see the ESI†) imply that there are well separated high excited-energy levels for Dy6(L)6 (see the ESI†). In addition, a butterfly-shaped magnetic hysteresis loop can be detected at 1.9 K, indicative of the presence of fast quantum tunnelling of the magnetization process in Dy6(L)6.
To verify SMM behavior, the frequency- and temperature-dependent AC susceptibilities of Dy6(L)6 and Dy12(L)8 were measured in a zero DC field (see the ESI†). For Dy12(L)8, no obvious χ′′ signal was observed in the temperature-dependent AC measurements, suggesting the presence of fast quantum tunneling relaxation of magnetization. By contrast, significant slow relaxation of magnetization is observed in the AC susceptibility measurements for Dy6(L)6 (Fig. 3). The well-defined frequency-dependent maxima up to 35 K and strong frequency dependence of the AC susceptibilities both demonstrate SMM behavior with a large thermal energy barrier (see the ESI†). Two closely spaced relaxation processes operate in the high-temperature regime as seen from the broad χ′′ peak and significantly broadened Cole–Cole plots (see the ESI†). The observation of two relaxation processes is common in multi-nuclear lanthanide systems and results from the two groups of distinct anisotropic centers in Dy6(L)6 (see the ESI†).29
The relaxation times (τ) for these two processes can be extracted from the excellent fit of the sum of two modified Debye functions (see the ESI†). The fitting of the data is in good agreement with the data for Dy6(L)6 over the entire temperature range with effective energy barriers of Ueff1 = 435 K (pre-exponential factor τ01 = 8.98 × 10−10 s) and Ueff2 = 254 K (τ02 = 7.4 × 10−9 s) for the slow relaxation (SR) and fast relaxation (FR) phases, respectively (see the ESI†). We also obtain parameters of C1 = 2.0295 × 10−5 s−1 K−5.3, n1 = 5.3 and C2 = 3.4718 × 10−4 s−1 K−5.09, and n2 = 5.09 for SR and FR processes, respectively (Fig. 4).
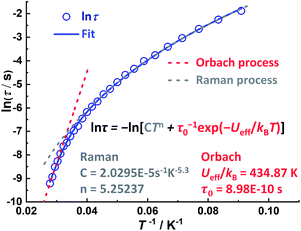 |
| Fig. 4 A plot of ln(τ/s) versus T−1 for SR of Dy6(L)6 under a zero dc-field; the relaxation times were obtained by simultaneous fitting of the Cole–Cole plots (Fig. S25†). The blue line represents the fit to multiple relaxation processes using eqn (S1).† Red and grey dashed lines represent individual Orbach and Raman fits, respectively. | |
In addition, the α values extracted from the sum of two modified Debye functions are less than 0.16 for SR, (see the ESI†) suggesting a relatively narrow distribution of the relaxation times. In contrast, the large α values (as large as 0.38) for FR indicate a wide distribution of relaxation times. Thus, Dy6(L)6 represents an interesting example of an oligonuclear DyIII SMM due to its unique closed helical structure and its effective energy barrier for the spin relaxation process of 435 K which is amongst the largest values within multinuclear lanthanide SMMs (see the ESI†).30
Conclusion
In conclusion, we have shown how subcomponent self-assembly can give rise to facile ligand synthesis and spontaneous formation of two very different hexa- and dodecanuclear lanthanide metallosupramolecular architectures by employing the different second-order template effects arising from the base and the dysprosium's counterions. While the base trimethylamine acts as an efficient secondary template to favor the formation of a hexanuclear Dy6(L)6 assembly by perfect space filling of the assembly's central void, the sodium ions from sodium bicarbonate act as secondary templates by being capable of occupying vacant coordination sites, and hence, lead to the formation of the Dy12(L)8 aggregate. This highlights the possibilities for in situ formation of flexible ligand structures furnished with multiple donor centers that offer a plethora of different bi- or tridentate metal binding sites that can adopt different conformations to access different complicated metallosupramolecular architectures by rather subtle changes in the subcomponent self-assembly process.
Dy6(L)6 not only has an extraordinary structure but it also exhibits clear butterfly-shaped magnetic hysteresis at 1.9 K and one of the largest energy barriers of 435 K within multinuclear lanthanide SMMs.
Thus, we envision this strategy to be very promising to address the formation of new (supra-)molecular structures with complex topologies and to learn more about how to understand, and ultimately predict, the self-assembly of lanthanide-based systems in a similarly reliable way as is already possible for transition metal systems.
Data availability
All data used to reach the results presented in this manuscript can be found in the ESI.†
Author contributions
J. T. conceived and designed the project. X.-L. L. carried out the synthesis and characterization studies, with the help of L. Z., J. W., W. S. and P. C. J. T. planned and executed the magnetic measurements and analysed the data. N. S. and A. L. analysed the ESI mass spectra. X.-L. L., J. T., A. L. and A. K. P. wrote the manuscript, with contributions from all the co-authors.
Conflicts of interest
The authors declare no conflict of interest.
Acknowledgements
We thank the National Natural Science Foundation of China (21871247, 21801237 and 21971123), the Key Research Program of Frontier Sciences, CAS (ZDBS-LY-SLH023), the Key Research Program of the Chinese Academy of Sciences (ZDRW-CN-2021-3-3), the National Science and Technology Major Project (2020YFE0204500), the Natural Science Foundation of Jilin Province of China (20200201244JC), the China Postdoctoral Science Foundation (2021M693397) and the POF STN of the Helmholtz Foundation for financial support. X.-L. L. is thankful to the Chinese Academy of Sciences for a Special Research Assistant Grant (2021000162). J. T. gratefully acknowledges support of the Royal Society-Newton Advanced Fellowship (NA160075). N. S. is thankful to the Evonik Foundation for a doctoral grant.
References
-
(a) M. Albrecht, Chem. Rev., 2001, 101, 3457 CrossRef CAS PubMed;
(b) J.-C. G. Bünzli and C. Piguet, Chem. Rev., 2002, 102, 1897 CrossRef PubMed;
(c) G. Benelli and D. Gateschi, Chem. Rev., 2002, 102, 2369 CrossRef PubMed;
(d) J.-C. G. Bünzli and C. Piguet, Chem. Soc. Rev., 2005, 34, 1048 RSC;
(e) R. Sessoli and A. K. Powell, Coord. Chem. Rev., 2009, 253, 2328 CrossRef CAS;
(f) S. V. Eliseeva and J.-C. G. Bünzli, Chem. Soc. Rev., 2010, 39, 189 RSC;
(g) S. V. Eliseeva and J.-C. G. Bünzli, New J. Chem., 2011, 35, 1165 RSC;
(h) P. Zhang, Y.-N. Guo and J. Tang, Coord. Chem. Rev., 2013, 257, 1728 CrossRef CAS;
(i) S. J. Bradberry, A. J. Savyasachi, M. Martinez-Calvo and T. Gunnlaugsson, Coord. Chem. Rev., 2014, 273–274, 226 CrossRef CAS;
(j) J.-C. G. Bünzli, J. Coord. Chem., 2014, 67, 3706 CrossRef;
(k) K. Liu, X. Zhang, X. Meng, W. Shi, P. Cheng and A. K. Powell, Chem. Soc. Rev., 2016, 45, 2423 RSC;
(l) D. E. Barry, D. F. Caffrey and T. Gunnlaugsson, Chem. Soc. Rev., 2016, 45, 3244 RSC;
(m) Z. Zhu, M. Guo, X.-L. Li and J. Tang, Coord. Chem. Rev., 2019, 378, 350 CrossRef CAS;
(n) X.-L. Li and J. Tang, Dalton Trans., 2019, 48, 15358 RSC;
(o) X. Liu and J.-R. Hamon, Coord. Chem. Rev., 2019, 389, 94 CrossRef CAS;
(p) H.-Y. Wong, W.-S. Lo, K.-H. Yim and G.-L. Law, Chem, 2019, 5, 3058 CrossRef CAS;
(q) X.-Z. Li, C.-B. Tian and Q.-F. Sun, Chem. Rev., 2022, 122, 6374 CrossRef CAS PubMed.
- For some reviews on structural aspects see:
(a) K. Suzuki, M. Tominaga, M. Kawano and M. Fujita, Chem. Commun., 2009, 45, 1638 RSC;
(b) R. Chakrabaty, P. S. Mukherjee and P. J. Stang, Chem. Rev., 2011, 111, 5810 Search PubMed;
(c) T. R. Cook, Y. R. Zheng and P. J. Stang, Chem. Rev., 2013, 113, 734 CrossRef CAS PubMed;
(d) M. M. J. Smulders, I. A. Riddell, C. Browne and J. R. Nitschke, Chem. Soc. Rev., 2013, 42, 1728 RSC;
(e) K. Harris, D. Fujita and M. Fujita, Chem. Commun., 2013, 49, 6703 RSC;
(f) S. Mukherjee and P. S. Mukherjee, Chem. Commun., 2014, 50, 2239 RSC;
(g) M. Han, D. M. Engelhard and G. H. Clever, Chem. Soc. Rev., 2014, 43, 1848 RSC;
(h) L. Chen, Q. Chen, M. Wu, F. Jiang and M. Hong, Acc. Chem. Res., 2015, 48, 201 CrossRef CAS PubMed;
(i) H. Li, Z.-J. Yao, D. Liu and G.-X. Jin, Coord. Chem. Rev., 2015, 293–294, 139 CrossRef CAS;
(j) T. R. Cook and P. J. Stang, Chem. Rev., 2015, 115, 7001 CrossRef CAS PubMed;
(k) L.-J. Chen, H.-B. Yang and M. Shinoya, Chem. Soc. Rev., 2017, 46, 2555 RSC;
(l) Y.-Y. Zhang, W.-X. Gao, L. Lin and G.-X. Jin, Coord. Chem.
Rev., 2017, 344, 323 CrossRef CAS;
(m) Q. Yang and J. Tang, Dalton Trans., 2019, 48, 769 RSC.
- For some reviews on functional aspects see:
(a) D. Fielder, D. H. Leung, R. G. Bergman and K. N. Raymond, Acc. Chem. Res., 2005, 38, 349 CrossRef PubMed;
(b) M. Fujita, M. Tominaga, A. Hori and B. Therrien, Acc. Chem. Res., 2005, 38, 369 CrossRef CAS PubMed;
(c) M. J. Hannon, Chem. Soc. Rev., 2007, 36, 280 RSC;
(d) H. Amouri, C. Desmarets, A. Bettoschi, M. N. Rager, K. Boubekeur, P. Rabu and M. Drillon, Chem.–Eur. J., 2007, 13, 5401 CrossRef CAS PubMed;
(e) A. Bousseksou, G. Molnár, J. A. Real and K. Tanaka, Coord. Chem. Rev., 2007, 251, 1822 CrossRef CAS;
(f) J. A. Kitchen and S. Brooker, Coord. Chem. Rev., 2008, 252, 2072 CrossRef CAS;
(g) M. Yoshizawa, J. K. Klosterman and M. Fujita, Angew. Chem., Int. Ed., 2009, 48, 3418 CrossRef CAS PubMed;
(h) J. Olguín and S. Brooker, Coord. Chem. Rev., 2011, 255, 203 CrossRef;
(i) T. R. Cook, V. Vajpayee, M. H. Lee, P. J. Stang and K.-W. Chi, Acc. Chem. Res., 2013, 46, 2464 CrossRef CAS PubMed;
(j) M. D. Ward and P. R. Raithby, Chem. Soc. Rev., 2013, 42, 1619 RSC;
(k) M. L. Saha, S. Neogi and M. Schmittel, Dalton Trans., 2014, 43, 3815 RSC;
(l) X. Yan, T. R. Cook, P. Wang, F. Huang and P. J. Stang, Nat. Chem., 2015, 7, 342 CrossRef CAS PubMed;
(m) S. Zarra, D. M. Wood, D. A. Roberts and J. R. Nitschke, Chem. Soc. Rev., 2015, 44, 419 RSC;
(n) S. H. A. M. Leenders, R. Gramaga-Doria, B. de Bruin and J. N. H. Reek, Chem. Soc. Rev., 2015, 44, 433 RSC;
(o) C. J. Brown, F. D. Toste, R. G. Bergman and K. N. Raymond, Chem. Rev., 2015, 115, 3012 CrossRef CAS PubMed;
(p) J. E. M. Lewis, P. D. Beer, S. J. Loeb and S. M. Goldup, Chem. Soc. Rev., 2017, 46, 2577 RSC;
(q) R. W. Hogue, S. Singh and S. Brooker, Chem. Soc. Rev., 2018, 47, 7303 RSC;
(r) M. Hardy and A. Lützen, Chem.–Eur. J., 2020, 26, 13332 CrossRef CAS PubMed.
-
(a) R. Krämer, J.-M. Lehn and A. Marquis-Rigault, Proc. Natl. Acad. Sci. U. S. A., 1993, 90, 5394 CrossRef PubMed;
(b) S. De, K. Mahata and M. Schmittel, Chem. Soc. Rev., 2010, 39, 1555 RSC.
- J.-F. Lemomnnier, L. Guénée, G. Bernardinelli, J. F. Vigier, B. Bocquet and C. Piguet, Inorg. Chem., 2010, 49, 1252 CrossRef PubMed.
- A. Adhikary, H. S. Jena, S. Khatua and S. Konar, Chem.–Asian J., 2014, 9, 1083 CrossRef CAS PubMed.
- A. M. Castilla, W. J. Ramsay and J. R. Nitschke, Acc. Chem. Res., 2014, 47, 2063 CrossRef CAS PubMed.
-
(a) J.-F. Ayme, J. E. Beves, D. A. Leigh, R. T. McBurney, K. Rissanen and D. Schultz, Nat. Chem., 2012, 4, 15 CrossRef CAS PubMed;
(b) J.-F. Ayme, J. E. Beves, D. A. Leigh, R. T. McBurney, K. Rissanen and D. Schultz, J. Am. Chem. Soc., 2012, 134, 9488 CrossRef CAS PubMed;
(c) J.-F. Ayme, J. E. Beves, C. J. Campbell and D. A. Leigh, Angew. Chem., Int. Ed., 2014, 53, 7823 CrossRef CAS PubMed;
(d) X.-P. Zhou, Y. Wu and D. Li, J. Am. Chem. Soc., 2013, 135, 16062 CrossRef CAS PubMed;
(e) S. Hong, M. R. Rohman, J. Jia, Y. Kim, D. Moon, Y. Kim, Y. H. Ko, E. Lee and K. Kim, Angew. Chem., Int. Ed., 2015, 54, 13241 CrossRef CAS PubMed;
(f) P. D. Frischmann, V. Kunz, V. Stepaneko and F. Würthner, Chem.–Eur. J., 2015, 21, 2766–2769 CrossRef CAS PubMed;
(g) P. D. Frischmann, V. Kunz and F. Würthner, Angew. Chem., Int. Ed., 2015, 54, 7285 CrossRef CAS PubMed;
(h) C. J. E. Haynes, J. Zhu, C. Chimerel, S. Hernández-Ainsa, I. A. Riddell, T. K. Ronson, U. F. Keyser and J. R. Nitschke, Angew. Chem., Int. Ed., 2017, 56, 15388 CrossRef CAS PubMed;
(i) R. Saha, D. Samanta, A. J. Bhattacharyya and P. S. Mukherjee, Chem.–Eur. J., 2017, 23, 8980 CrossRef CAS PubMed;
(j) M. Hardy, N. Struch, F. Topić, G. Schnakenburg, K. L. Rissanen and A. Lützen, Inorg. Chem., 2018, 57, 3507 CrossRef CAS PubMed;
(k) I. Sinha and P. S. Mukherjee, Inorg. Chem., 2018, 57, 4205 CrossRef CAS PubMed;
(l) D. Zhang, T. K. Ronson, J. Mosquera, A. Martinez and J. R. Nitschke, Angew. Chem., Int. Ed., 2018, 57, 3717 CrossRef CAS PubMed;
(m) J. Anhäuser, R. Puttreddy, L. Glanz, A. Schneider, M. Engeser, K. Rissanen and A. Lützen, Chem.–Eur. J., 2019, 25, 12294 CrossRef PubMed.
-
(a) M. C. Young, L. R. Holloway, A. M. Johnson and R. J. Hooley, Angew. Chem., Int. Ed., 2014, 53, 9832 CrossRef CAS PubMed;
(b) W. J. Ramsay, F. J. Rizzuto, T. K. Ronson, K. Caprice and J. R. Nitschke, J. Am. Chem. Soc., 2016, 138, 7264 CrossRef CAS PubMed;
(c) T. K. Ronson, B. S. Pilgrim and J. R. Nitschke, J. Am. Chem. Soc., 2016, 138, 10417 CrossRef CAS PubMed;
(d) S.-J. Hu, X.-Q. Guo, L.-P. Zhou, D.-N. Yan, P.-M. Cheng, L.-X. Cai, X.-Z. Li and Q.-F. Sun, J. Am. Chem. Soc., 2022, 144, 4244 CrossRef CAS PubMed.
-
(a) P. Mal, D. Schultz, K. Beyeh, K. Rissanen and J. R. Nitschke, Angew. Chem., Int. Ed., 2008, 47, 8297 CrossRef CAS PubMed;
(b) W. Meng, T. K. Ronson, J. K. Clegg and J. R. Nitschke, Angew. Chem., Int. Ed., 2013, 52, 1017 CrossRef CAS PubMed;
(c) X.-P. Zhou, Y. Wu and D. Li, J. Am. Chem. Soc., 2013, 135, 16062 CrossRef CAS PubMed;
(d) D. Samanta and P. S. Mukherjee, Chem.–Eur. J., 2014, 20, 12483 CrossRef CAS PubMed;
(e) A. J. McConnell, C. M. Aitchison, A. B. Grommet and J. R. Nitschke, J. Am. Chem. Soc., 2017, 139, 6294 CrossRef CAS PubMed;
(f) N. Struch, F. Topić, K. Rissanen and A. Lützen, Dalton Trans., 2017, 46, 10809 RSC;
(g) N. Struch, F. Topić, G. Schnakenburg, K. Rissanen and A. Lützen, Inorg. Chem., 2018, 57, 241 CrossRef CAS PubMed;
(h) M. Hardy, N. Struch, J. J. Holstein, G. Schnakenburg, N. Wagner, M. Engeser, J. Beck, G. H. Clever and A. Lützen, Angew. Chem., Int. Ed., 2020, 59, 3195 CrossRef CAS PubMed.
-
(a) D.-H. Ren, D. Qiu, C.-Y. Pang, Z. Li and Z.-G. Gu, Chem. Commun., 2015, 51, 788–791 RSC;
(b) N. Struch, G. Schnakenburg, R. Weisbarth, S. Klos, J. Beck and A. Lützen, Dalton Trans., 2016, 45, 14023–14029 RSC;
(c) N. Struch, C. Bannwarth, T. K. Ronson, Y. Lorenz, B. Mienert, N. Wagner, M. Engeser, E. Bill, R. Puttreddy, K. Rissanen, J. Beck, S. Grimme, J. R. Nitschke and A. Lützen, Angew. Chem., Int. Ed., 2017, 56, 4930 CrossRef CAS PubMed;
(d) A. J. McConnell, Supramol. Chem., 2018, 30, 858 CrossRef CAS;
(e) M. Hardy, J. Tessarolo, J. J. Holstein, N. Struch, N. Wagner, R. Weisbarth, M. Engeser, J. Beck, S. Horiuchi, G. H. Clever and A. Lützen, Angew. Chem., Int. Ed., 2021, 60, 22562 CrossRef CAS PubMed.
- For some recent examples see:
(a) S.-Y. Lin, W. Wernsdorfer, L. Ungur, A. K. Powell, Y.-N. Guo, J. Tang, L. Zhao, L. F. Chibotaru and H.-J. Zhang, Angew. Chem., Int. Ed., 2016, 55, 15574 CrossRef PubMed;
(b) X. Zhang, S. Liu, V. Vieru, N. Xu, C. Gao, B.-W. Wang, W. Shi, L. F. Chibotaru, S. Gao, P. Cheng and A. K. Powell, Chem.–Eur. J., 2018, 24, 6079 CrossRef CAS PubMed;
(c) V. Vieru, L. Ungur, V. Cemortan, A. Sukhanov, A. Baniodeh, C. E. Anson, A. K. Powell, V. Voronkova and L. F. Chibotaru, Chem.–Eur. J., 2018, 24, 16652 CrossRef CAS PubMed;
(d) L. Chertan, S. F. M. Schmidt, H. Aueerbach, T. Hochdörffer, J. A. Wollny, W. Bi, J. Zhao, M. Y. Hu, T. Toellner, E. E. Alp, D. E. Brown, C. E. Anson, A. K. Powell and V. Schünemann, Angew. Chem., Int. Ed., 2019, 58, 3444 CrossRef PubMed;
(e) K. Griffiths, I. A. Kuhne, G. J. Tizzard, S. J. Coles, G. E. Kostakis and A. K. Powell, Inorg. Chem., 2019, 58, 2483 CrossRef CAS PubMed;
(f) T. Bereta, A. Mondal, K. Slepokura, Y. Peng, A. K. Powell and J. Lisowski, Inorg. Chem., 2019, 58, 4201 CrossRef CAS PubMed;
(g) Y. Peng, M. K. Singh, V. Mereacre, C. E. Anson, G. Rajamaran and A. K. Powell, Chem. Sci., 2019, 10, 5528 RSC;
(h) M. Perfetti, J. Rinck, G. Cucinotta, C. E. Anson, Y. Gong, L. Ungur, L. Chibotura, M.-E. Boulon, A. K. Powell and R. Sessoli, Front. Chem., 2019, 7, 6 CrossRef CAS PubMed;
(i) A. Khan, O. Fuhr, M. N. Akhtar, Y. Lan, M. Thomas and A. K. Powell, J. Coord. Chem., 2020, 73, 1045 CrossRef CAS;
(j) P. Hahn, S. Ullmann, J. Klose, Y. Peng, A. K. Powell and B. Kersting, Dalton Trans., 2020, 49, 10901 RSC;
(k) H. Kaemmerer, A. Baniodeh, Y. Peng, E. Moreno-Pineda, M. Schulze, C. E. Anson, W. Wernsdorfer, J. Schnack and A. K. Powell, J. Am. Chem. Soc., 2020, 142, 14838 CrossRef CAS PubMed.
- For some recent examples see:
(a) J. Wu, J. Jung, P. Zhang, H. Zhang, J. Tang and B. Le Guennic, Chem. Sci., 2016, 7, 3632 RSC;
(b) X.-L. Li, J. Wu, L. Zhao, W. Shi, P. Cheng and J. Tang, Chem. Commun., 2017, 53, 3026 RSC;
(c) J. Wu, M. Guo, X.-L. Li, L. Zhao, Q.-F. Sun, R. A. Layfield and J. Tang, Chem. Commun., 2018, 54, 12097 RSC;
(d) Y. Zhang, B. Ali, J. Wu, M. Guo, Y. Yu, Z. Liu and J. Tang, Inorg. Chem., 2019, 58, 3167 CrossRef CAS PubMed;
(e) J. Lu, X.-L. Li, Z. Zhu, S. Liu, Q. Yang and J. Tang, Dalton Trans., 2019, 48, 14062 RSC;
(f) J. Lu, X.-L. Li, C. Jin, Y. Yu and J. Tang, New J. Chem., 2020, 44, 994 RSC;
(g) C. Jin, X.-L. Li, Z. Liu, A. Mansikkamäki and J. Tang, Dalton Trans., 2020, 49, 10477 RSC;
(h) Z. Zhu, Y.-Q. Zhang, X.-L. Li, M. Guo, J. Lu, S. Liu, A. Layfield Richard and J. Tang, CCS Chem., 2021, 3, 388 CrossRef CAS;
(i) Z. Zhu, C. Zhao, T. Feng, X. Liu, X. Ying, X.-L. Li, Y.-Q. Zhang and J. Tang, J. Am. Chem. Soc., 2021, 143, 10077 CrossRef CAS PubMed;
(j) Z. Zhu, C. Zhao, Q. Zhou, S. Liu, X.-L. Li, A. Mansikkamäki and J. Tang, CCS Chem., 2022 DOI:10.31635/ccschem.022.202101604.
- X. L. Li, H. Li, D. M. Chen, C. Wang, J. Wu, J. Tang, W. Shi and P. Cheng, Dalton Trans., 2015, 44, 20316 RSC.
- M. B. Bushuev, V. P. Krivopalov, N. V. Semikolenova, Y. G. Shvedenkov, L. A. Sheludyakova, G. G. Moskalenko, L. G. Lavrenova, V. A. Zakharov and S. V. Larionov, Russ. J. Coord. Chem., 2007, 33, 601 CrossRef CAS.
- O. V. Dolomanov, L. J. Bourhis, R. J. Gildea, J. A. K. Howard and H. Puschmann, J. Appl. Crystallogr., 2009, 42, 339 CrossRef CAS.
-
L. N. M. E. A. Boudreaux, Theory and Applications of Molecular Paramagnetism, John Wiley & Sons, New York, 1976 Search PubMed.
- Please note that the amount of base used in the two subcomponent self-assembly processes proved to be critical for the successful formation of the oligonuclear supramolecular architectures as single crystalline Dy6(L)6 and D12(L)8 could only be obtained using the relative ratios given in the experimental part.
- Please note that all attempts to assemble the oligonuclear complexes from the preformed protonated ligand H2(L) were not successful.
-
(a) B. Bocquet, G. Bernardinelli, N. Ouali, S. Floquet, F. Renaud, G. Hopfgartner and C. Piguet, Chem. Commun., 2002, 930 RSC;
(b) K. Zeckert, J. Hamacek, J.-P. Rivera, S. Floquet, A. Pinto, M. Borkovec and C. Piguet, J. Am. Chem. Soc., 2004, 126, 11589 CrossRef CAS PubMed;
(c) J. Hamacek, S. Blanc, M. Elhabiri, E. Leize, A. Van Dorsselaer, C. Piguet and A.-M. Albrecht-Gary, J. Am. Chem. Soc., 2003, 125, 1541 CrossRef CAS PubMed.
- B. Wang, Z. Zang, H. Wang, W. Dou, X. Tang, W. Liu, Y. Shao, J. Ma, Y. Li and J. Zhou, Angew. Chem., Int. Ed., 2013, 52, 3756 CrossRef CAS PubMed.
-
(a) J.-M. Senegas, S. Koeller, G. Bernardinelli and C. Piguet, Chem. Commun., 2005, 2235 RSC;
(b) S.-Y. Lin, L. Zhao, Y.-N. Guo, P. Zhang, Y. Guo and J. Tang, Inorg. Chem., 2012, 51, 10522 CrossRef CAS PubMed;
(c) L. Zhang, P. Zhang, L. Zhao, J. Wu, M. Guo and J. Tang, Inorg. Chem., 2015, 54, 5571 CrossRef CAS PubMed.
- T. Y. Bing, T. Kawai and J. Yuasa, J. Am. Chem. Soc., 2018, 140, 3683 CrossRef CAS PubMed.
- J. Lu, V. Montigaud, O. Cador, J. Wu, L. Zhao, X.-L. Li, M. Guo, B. Le Guennic and J. Tang, Inorg. Chem., 2019, 58, 11903 CrossRef CAS PubMed.
-
(a) B. Hasenknopf, J.-M. Lehn, B. O. Kneisel, G. Baum and D. Fenske, Angew. Chem., Int. Ed., 1996, 35, 1838 CrossRef CAS;
(b) P. L. Jones, K. J. Byrom, J. C. Jefferey, J. A. McCleverty and M. D. Ward, Chem. Commun., 1997, 1361 RSC;
(c) B. Hasenknopf, J.-M. Lehn, N. Boumediene, A. Dupont-Gervais, A. Van Dorsselaer, B. Kneisel and D. Fenske, J. Am. Chem. Soc., 1997, 119, 10956 CrossRef CAS;
(d) B. Hasenknopf, J.-M. Lehn, N. Boudediene, E. Leize and A. Van Dorsselaer, Angew. Chem., Int. Ed., 1998, 37, 3265 CrossRef CAS;
(e) C. S. Campos-Fernandes, B. L. Schottel, H. T. Chifotides, J. K. Bera, J. Bacsa, J. M. Koomen, D. H. Russell and K. R. Dunbar, J. Am. Chem. Soc., 2005, 127, 12909 CrossRef PubMed;
(f) S. P. Argent, H. Adams, T. Riis-Johannessen, J. C. Jefferey, L. P. Harding, O. Mamula and M. D. Ward, Inorg. Chem., 2006, 45, 3905 CrossRef CAS PubMed;
(g) H. B. Tanh Jeazet, K. Gloe, T. Doert, O. N. Kataeva, A. Jäger, G. Geipel, G. Bernhard, B. Büchner and K. Gloe, Chem. Commun., 2010, 46, 2373 RSC;
(h) C. Klein, C. Gütz, M. Bogner, F. Topić, K. Rissanen and A. Lützen, Angew. Chem., Int. Ed., 2014, 53, 3739 CrossRef CAS PubMed.
- Please note that the use of amino-2-propanol instead of NEt3 also gave rise to the same Dy6(L)6 architecture; however, its structure could not be completely satisfyingly resolved based on the single crystal XRD analysis (see the ESI†).
-
(a) M. D. Wise, J. J. Holstein, P. Pattison, C. Besnard, E. Solari, R. Scopelliti, G. Bricogne and K. Severin, Chem. Sci., 2015, 6, 1004 RSC;
(b) M. Käseborn, J. J. Holstein, G. H. Clever and A. Lützen, Angew. Chem., Int. Ed., 2018, 57, 12171 CrossRef PubMed.
- Some further reviews on lanthanide single-moelcule magnets not covered by ref. 1:
(a)
D. Gatteschi, R. Sessoli and R. Villain, Molecular Nanomagentes, Oxford University Press, Oxford, 2006 CrossRef;
(b) D. N. Woodruff, R. E. Winpenny and R. A. Layfield, Chem. Rev., 2013, 113, 5110 CrossRef CAS PubMed;
(c) H. L. C. Feltham and S. Brooker, Coord. Chem. Rev., 2014, 276, 1 CrossRef CAS;
(d) F. Pointillart, O. Cador, B. LeGuennic and L. Ouahab, Coord. Chem. Rev., 2017, 346, 150 CrossRef CAS;
(e) S. G. McAdams, A.-M. Ariciu, A. K. Kostopoulos, J. P. S. Walsh and F. Tuna, Coord. Chem. Rev., 2017, 346, 216 CrossRef CAS;
(f) J.-L. Liu, Y.-C. Chen and M.-L. Tong, Chem. Soc. Rev., 2018, 47, 2431 RSC;
(g) F.-S. Guo, A. K. Bar and R. A. Layfield, Chem. Rev., 2019, 119, 8479 CrossRef CAS PubMed;
(h) Z. Zhu, X.-L. Li, S. Liu and J. Tang, Inorg. Chem. Front., 2020, 7, 3315 RSC.
- Y.-N. Guo, G.-F. Xu, P. Gamez, L. Zhao, S.-Y. Lin, R. Deng, J. Tang and H.-J. Zhang, J. Am. Chem. Soc., 2010, 132, 8538 CrossRef CAS PubMed.
-
(a) I. J. Hewitt, J. Tang, N. T. Madhu, C. E. Anson, Y. Lan, J. Luzon, M. Etienne, R. Sessoli and A. K. Powell, Angew. Chem., Int. Ed., 2010, 49, 6352 CrossRef CAS PubMed;
(b) R. J. Blagg, C. A. Muryn, E. J. L. McInnes, F. Tuna and R. E. P. Winpenny, Angew. Chem., Int. Ed., 2011, 50, 6530 CrossRef CAS PubMed;
(c) R. J. Blagg, F. Tuna, E. J. L. McInnes and R. E. P. Winpenny, Chem. Commun., 2011, 47, 10587 RSC;
(d) P.-H. Guo, J. Liu, Z.-H. Wu, H. Yan, Y.-C. Chen, J.-H. Jia and M.-L. Tong, Inorg. Chem., 2015, 54, 8087 CrossRef CAS PubMed;
(e) T. Pugh, V. Vieru, L. F. Chibotaru and R. A. Layfield, Chem. Sci., 2016, 7, 2128 RSC;
(f) T. Pugh, N. F. Chilton and R. A. Layfield, Chem. Sci., 2017, 8, 2073 RSC;
(g) J. Xiong, H.-Y. Ding, Y.-S. Meng, C. Gao, X.-J. Zhang, Z.-S. Meng, Y.-Q. Zhang, W. Shi, B.-W. Wang and S. Gao, Chem. Sci., 2017, 8, 1288 RSC;
(h) J. D. Rinehart, M. Fang, W. J. Evans and J. R. Long, J. Am. Chem. Soc., 2011, 133, 14236 CrossRef CAS PubMed;
(i) R. J. Blagg, L. Ungur, F. Tuna, J. Speak, P. Comar, D. Collison, W. Wernsdorfer, E. J. L. McInnes, L. F. Chibotaru and R. E. P. Winpenny, Nat. Chem., 2013, 5, 673 CrossRef CAS PubMed;
(j) C. A. Gould, K. R. McClain, D. Reta, J. G. C. Kragskow, D. A. Marchiori, E. Lachman, E.-S. Choi, J. G. Analytis, R. D. Britt, N. F. Chilton, B. G. Harvey and J. R. Long, Science, 2022, 375, 198 CrossRef CAS PubMed.
Footnotes |
† Electronic supplementary information (ESI) available: Experimental details and additional figures (Tables S1–S8 and Fig. S1–S28. CCDC 1507720 and 1536333. For ESI and crystallographic data in CIF or other electronic format see https://doi.org/10.1039/d2sc03156f |
‡ Current address: Arlanxeo Deutschland GmbH, Alte Heerstraβe 2, 41540 Dormagen, Germany. |
|
This journal is © The Royal Society of Chemistry 2022 |
Click here to see how this site uses Cookies. View our privacy policy here.