DOI:
10.1039/D2SC03375E
(Edge Article)
Chem. Sci., 2022,
13, 11360-11367
A platinum–ruthenium hybrid prodrug with multi-enzymatic activities for chemo-catalytic therapy of hypoxic tumors†
Received
16th June 2022
, Accepted 14th August 2022
First published on 9th September 2022
Abstract
Regulation of tumor hypoxia and redox homeostasis is a promising strategy for cancer therapy. Nanocatalytic medicine has played more and more important roles in this field because it can cleverly convert the efficiency and selectivity of catalysis into high therapeutic efficiency. Herein, we developed a platinum(IV)–ruthenium hybrid prodrug, named as Pt–Ru, for efficient chemo-catalytic synergistic therapy of hypoxic tumors. The ruthenium hybridization endowed the Pt(IV) prodrug with multi-enzyme catalytic activity, that is, mimicking catalase (CAT) to generate O2in situ, mimicking peroxidase (POD) to produce reactive oxygen species, and mimicking glutathione peroxidase (GPx) to deplete GSH, thus effectively overcoming tumor hypoxia and cisplatin resistance. As a result, Pt–Ru treatment led to a superior anticancer efficacy to cisplatin both in vitro and in vivo. This work suggested redox homeostasis regulation as a tantalizing angle for developing the next generation of platinum drugs.
Introduction
In recent years, the concept of “nanocatalytic medicine” has attracted extensive attention and played more and more important roles in the field of cancer therapy.1–3 This emerging strategy cleverly converts the efficiency and selectivity of catalysis into high therapeutic efficiency with reduced side effects.4 Compared with normal tissue cells, tumor cells metabolize in different ways to form a characteristic tumor microenvironment and redox homeostasis, thus making it possible to trigger catalytic therapy selectively in tumor cells and tissues.5,6
Hypoxia, characterized by a low oxygen concentration, is a prominent feature of a tumor microenvironment playing important roles in tumor recurrence, angiogenesis and metastasis.7–9 Extensive evidence has demonstrated that hypoxia can seriously reduce the efficacy of chemotherapeutic drugs and induce multi-drug resistance in the treatment of solid tumors.10–12 Therefore, chemotherapeutic drugs which can overcome tumor hypoxia are highly required. Cisplatin is one of the most widely used antitumor drugs in clinics, and continuous efforts are still being made to address these serious problems of cisplatin resistance and side effects.13–16 Recently, the nanocatalytic medicine strategy has been used to improve the efficacy of platinum drugs, especially against hypoxic tumors,17–19 for example, co-delivery of catalase and Pt(II) drugs by poly(D,L-lactic-co-glycolic acid) nanoparticles, and encapsulation of catalase inside liposomes consisting of Pt(IV) prodrug-conjugated phospholipids, both realizing hypoxia relief by catalyzing endogenous hydrogen peroxide (H2O2) to generate oxygen.20,21 Inorganic nanomaterials such as MnO2 capable of mimicking catalase were also reported to enhance the efficacy of platinum drugs against hypoxic tumors via incorporation inside human serum protein (HSA) modified Pt(IV) prodrugs.22 However, co-loading of platinum drugs and exogenous oxygen production catalysts may bring inevitable catalyst leakage and nanomaterial safety problems.23,24 For this reason, we have recently designed a novel platinum(IV) prodrug, CAIXplatin, which can endogenously regulate oxygen contents by targeting and inhibiting carbonic anhydrase IX.17
Besides hypoxia, a high concentration of glutathione (GSH) in cancer cells (∼10 mM) is a well-known cause of cisplatin resistance and detoxification, due to the extensive Pt-GSH conjugation and rapid export from cells via the glutathione S-conjugate pump.25–27 Due to redox homeostasis, cancer cells also possess higher reactive oxygen species (ROS) levels than normal cells, meaning that cancer cells are more vulnerable to oxidative stress.28–30 Inspired by these, development of multifunctional platinum drugs capable of alleviating hypoxia, consuming GSH, and promoting oxidative stress simultaneously should have profound significance in the clinical treatment of cancer. Considering that ruthenium complexes are the most likely substitutes for platinum drugs, some of which have entered clinical trials (NAMI-A, TLD1433, etc.),31–33 and ultrasmall ruthenium oxide nanoenzymes have recently been reported with catalase activity,34–37 design of ruthenium hybrid platinum drugs may provide a tantalizing angle for efficient treatment of hypoxic tumors.
Herein, we developed a platinum(IV)–ruthenium hybrid prodrug, named as Pt–Ru, through a simple one-pot mixing step in water at room temperature. As shown in Scheme 1, ruthenium elements present mixed-valence states (Ru3+ and Ru4+) and act as glues to bind Pt(IV) complexes together, and most importantly, endow this newly developed Pt(IV) prodrug with multi-enzymatic activities, that is, catalase (CAT) activity to generate O2in situ, peroxidase (POD) activity to produce ROS, and glutathione peroxidase (GPx) to deplete GSH. Therefore, Pt–Ru treatment can effectively alleviate tumor hypoxia and show much higher antitumor efficacy than cisplatin both in vitro and in vivo. This study suggests that endowing drugs with multi-enzyme activities to regulate tumor redox homeostasis will be a novel strategy for developing the next generation of platinum drugs.
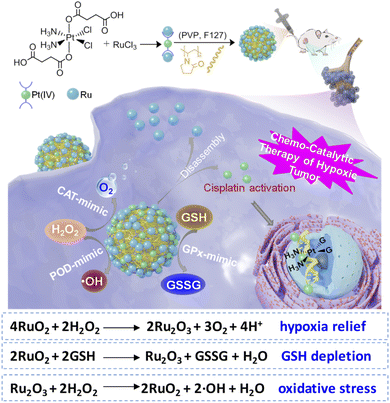 |
| Scheme 1 Self-assembled Pt–Ru hybrid prodrug with multi-enzymatic activities (CAT, POD, and GPx) for manipulating redox homeostasis and chemo-catalytic synergistic therapy of hypoxic tumors. | |
Results and discussion
Fabrication of Pt–Ru hybrids
The detailed synthesis process of Pt–Ru hybrids is illustrated in Scheme 1. Initially, a Pt(IV) prodrug functionalized with succinic anhydride as the axial ligand was synthesized, named as Pt(IV)–COOH and characterized by 1H NMR (Fig. S1, ESI†). Then Pt–Ru hybrids were fabricated via a simple and robust synthesis pathway under ambient conditions, where Pt(IV)–COOH, triethylamine and RuCl3 were simply mixed in water and then stirred with FDA approved water-soluble polymers (PVP and F127) at room temperature. Herein, ruthenium acted as an adhesive to assemble Pt(IV) prodrugs into nanoparticles by reacting with carboxyl groups, meanwhile, the mixed-valence properties of Ru endow nanoparticles with multi-enzyme activity. Transmission electron microscopy (TEM) and dynamic laser scattering (DLS) experiments showed that Pt–Ru hybrids were well distributed in aqueous solution with uniform spherical morphology, and the average particle sizes were 160–180 nm (Fig. 1A and B). The X-ray photoelectron spectroscopy (XPS) survey spectrum showed that Pt–Ru hybrids contained Pt, Ru, N, O and Cl elements (Fig. 1C), and the molar ratio of Pt
:
Ru = 1
:
2 was also determined by ICP-MS (Table S1, ESI†). The high-resolution XPS spectrum (Fig. 1D) further confirmed the presence of mixed-valence states of the Ru element: the peak at 281.6 eV and 284.9 eV could be attributed to Ru3+ and Ru4+, respectively,38 while the presence of Pt4+ was also verified by the XPS peak at 75.33 eV (Fig. S2, ESI†).39Pt–Ru hybrids exhibited excellent stability in water, PBS, 10% FBS for at least 5 days as indicated by the relatively stable hydrodynamic diameters (Fig. S3, ESI†). However, GSH (10 mM) could effectively promote the decomposition of Pt–Ru hybrids, as indicated by the gradually decreased DLS diameters from ca. 210 nm to 90 nm in 12 h (Fig. S4 and S5†, ESI). The drug release file of Pt–Ru in aqueous solution in the presence of GSH (1, 5 and 10 mM) was also monitored by ICP-MS. It is clear that GSH could promote the decomposition of Pt–Ru, and ca. 80% of Pt content could be released in 12 h, most likely in the form of cisplatin while only ca. 10% of Ru content was released under the same conditions (Fig. S6, ESI†). These data suggested that a high concentration of GSH in cancer cells could act as a reducing agent of Pt–Ru hybrids to release cisplatin.
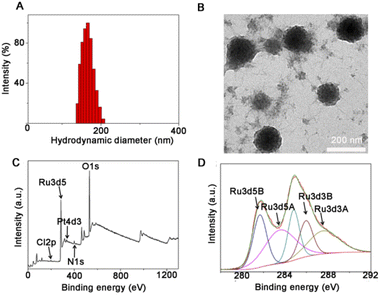 |
| Fig. 1 The morphology and composition of as-prepared Pt–Ru hybrids measured by (A) DLS; (B) TEM; (C and D) XPS of Pt–Ru. | |
Multi-enzymatic activities of Pt–Ru hybrids
First, the CAT mimic activity of Pt–Ru hybrids was evaluated by monitoring the decomposition of H2O2 to produce oxygen. As shown in Fig. 2A, the UV-visible absorbance of H2O2 (10 mM) was rapidly decreased in 5 min in the presence of Pt–Ru hybrids (20 μg), accompanied by bubble generation in the cuvette, indicating the rapid decomposition of H2O2. Meanwhile, the electron spin resonance (ESR) technique was employed to detect oxygen generation by using CTPO as an O2 trapping agent. As shown in Fig. 2B, CTPO had a characteristic triplet signal which was not affected by H2O2 alone.
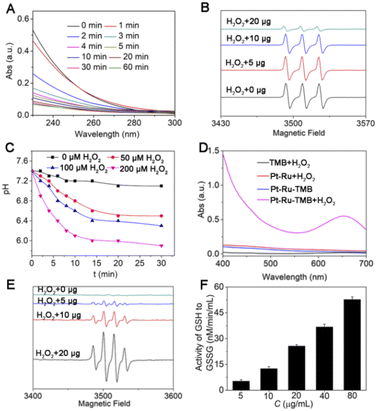 |
| Fig. 2 (A) Consumption of H2O2 over time in the presence of Pt–Ru (20 μg); effect of Pt–Ru at indicated doses on the (B) production of O2; (C) decrease of pH values; (D and E) generation of ˙OH, respectively, in the presence of H2O2; (F) effect of Pt–Ru on the production of GSSG from GSH. | |
However, such a signal was gradually quenched by Pt–Ru hybrids in a dose-dependent manner (5–20 μg) in the presence of the same concentration of H2O2, indicating oxygen production mediated by Pt–Ru hybrids. Moreover, the pH value of the Pt–Ru/H2O2 mixture solution gradually decreased with the progress of catalytic reaction, the more H2O2, the lower the pH value of the solution. For example, when the concentration of H2O2 was 200 μM, the pH value of the mixture solution decreased from 7.4 to 6.0 within 10 min, but only decreased to 6.8 when the H2O2 concentration was 50 μM (Fig. 2C). This further proved that Pt–Ru hybrids could simulate CAT activity by catalyzing H2O2 to produce oxygen and protons. Second, the POD mimic activity of Pt–Ru hybrids was evaluated by monitoring the ˙OH production from H2O2. Due to the high chemical activity and short lifetime of ˙OH, a colorimetric indicator 3,3′,5,5′-tetramethyl-benzidine (TMB) was employed, which could be oxidized by ˙OH accompanied by a colorless-to-blue color change. As shown in Fig. 2D, the oxidation of TMB only occurred when Pt–Ru and H2O2 were both present, as indicated by the emerging characteristic peak at 650 nm. The more H2O2, the more TMB oxidized, which verified the ˙OH production from H2O2 (Fig. S7, ESI†). Meanwhile, ESR spectroscopy was also employed using DMPO as an ˙OH trapping agent. With increasing amount of Pt–Ru hybrids (5–20 μg) in aqueous solution containing H2O2, a quadruplet ESR signal gradually emerged and increased, which was the characteristic peak of ˙OH (Fig. 2E). These findings demonstrated the POD-mimic activity of Pt–Ru hybrids that could catalyze H2O2 to produce ˙OH.
In addition, the GPx mimic activity of Pt–Ru hybrids was evaluated using a GPx assay kit, and we found that the GSSG/GSH ratio gradually increased in Pt–Ru treated cells in a dose-dependent manner (Fig. 2F). This was consistent with the intrinsic activity of GPx that catalyzed the oxidation of GSH to oxidized glutathione (GSSG). These findings demonstrated the multi-enzymatic activities (CAT, POD, and GPx) of Pt–Ru hybrids in the presence of H2O2, which would be highly beneficial for regulating redox homeostasis.
Redox homeostasis regulation and chemo-catalytic synergistic therapy
To verify the great potential of Pt–Ru hybrids in redox homeostasis regulation, e.g. alleviating hypoxia, enhancing oxidative stress and depleting GSH, we further investigated the catalytic behavior of Pt–Ru hybrids in living cells. Initially, the changes of intracellular O2 content were monitored by CLMS using RDDP ([Ru(dpp)3]Cl2) as the oxygen indicator, the fluorescence of which could be quenched by O2.40 As shown in Fig. 3A, the red fluorescence of RDDP in hypoxic cells was more intensive than that in normoxic cells, moreover, the fluorescence was remarkably quenched after Pt–Ru treatment (5 μM, 24 h) while not affected by cisplatin, indicating an increased O2 content in Pt–Ru treated cells. Once the hypoxic cells were pretreated with NAC (a ROS scavenger) to remove intracellular H2O2, Pt–Ru treatment could not affect the fluorescence of RDDP either, further confirming that intracellular H2O2 was the source of O2 in this case. Meanwhile, the immunofluorescence assay showed that the expression level of the hypoxia inducible factor (HIF-1α) in hypoxic MCF-7 cells was greatly reduced after Pt–Ru treatment (5 μM, 24 h), as indicated by the quenched red fluorescence, but not affected by cisplatin at the same concentration (Fig. 3B). This demonstrated that Pt–Ru could alleviate tumor hypoxia by catalyzing endogenous H2O2 to produce oxygen.
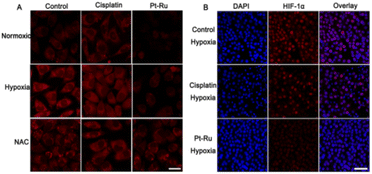 |
| Fig. 3 CLSM images of (A) O2 content and (B) HIF-1α expression in MCF-7 cells after 24 h treatment with cisplatin or Pt–Ru hybrids (5 μM). Scale bar = 20 μm (A); 100 μm (B). | |
Then we monitored the changes of intracellular ROS and GSH levels induced by Pt–Ru hybrids. Confocal microscopy showed that Pt–Ru treatment (5 μM, 24 h) could induce substantial ˙OH generation, as indicated by the emerging fluorescence from the ˙OH specific probe, which was not observed in cisplatin treated cells under the same conditions (Fig. 4A and B). However, the generation of hydroxyl radicals was negligible once the cells were pre-treated with NAC (Fig. S8, ESI†). Meanwhile, flow cytometry showed that Pt–Ru treatment could induce intracellular ROS elevation in a dose-dependent manner (Fig. S9, ESI†). We also monitored the capability of Pt–Ru hybrids to produce O2˙− as cisplatin was known to activate nicotinamide adenine dinucleotide phosphate oxidases (NOXs) that could catalyze O2 into O2˙−. Confocal microscopy using dihydroethidium as an O2˙− specific probe showed that both cisplatin and Pt–Ru hybrids could induce O2˙− generation in hypoxic cells, the latter of which induced more, further confirming the hypoxia relief in Pt–Ru treated cells which provided more O2 as a substrate for NOX catalysis (Fig. S10, ESI†). Furthermore, the GSH assay showed that the GSH levels in normoxic and hypoxic MCF-7 cells after Pt–Ru treatment were both significantly lower than that in the untreated or cisplatin treated cells (C and D). This would be beneficial to minimize GSH-medicated cisplatin detoxification. These results demonstrated the intracellular catalytic activity of Pt–Ru hybrids capable of simultaneously alleviating hypoxia, enhancing oxidative stress and depleting GSH.
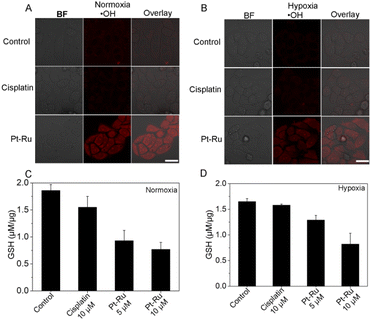 |
| Fig. 4 (A and B) CLSM images of ˙OH production and (C and D) GSH depletion in MCF-7 cells after incubation with cisplatin or Pt–Ru hybrids for 36 h under normoxia and hypoxia, respectively. Scale bar = 20 μm. | |
Inspired by these redox homeostasis regulation capabilities, the anticancer activity of Pt–Ru hybrids was evaluated under both normoxia (21% O2) and hypoxia (1% O2) using a 48 h MTT assay.
Cisplatin-sensitive breast (MCF-7) and cisplatin-resistant human lung (A549R) carcinoma cell lines were selected and cisplatin was adopted as a positive control. As shown in Table 1 and Fig. S11† (ESI), Pt–Ru hybrids always exhibited higher anticancer activity than cisplatin whether under normoxic or hypoxic conditions, suggesting an effective chemo-catalytic synergistic therapy. Moreover, the toxicity of Pt–Ru against cancer cells under hypoxia was similar to that under normoxia, while that of cisplatin was substantially reduced under hypoxia. Take the A549R cell line as an example, when the O2 concentration was decreased from 21% to 1%, the IC50 value of Pt–Ru remained ca. 19.3 ± 2.4 μM while that of cisplatin increased from 49.7 ± 3.7 μM to 65.2 ± 3.1 μM, indicating the capability of Pt–Ru to overcome hypoxia and cisplatin resistance. It was worth mentioning that the cytotoxicity of Pt–Ru was substantially decreased in NAC-pretreated cells while the cytotoxicity of cisplatin was not affected by NAC at all. This was reasonable as NAC performed as an H2O2 scavenger to diminish the substrate of catalysis reaction, thus inhibiting the catalytic activity of Pt–Ru.
Table 1 IC50 (μM) values of tested compounds towards different cell lines under normoxia and hypoxia, respectivelya
Drug |
MCF-7 |
NAC- pretreated MCF-7 |
A549R |
Nor. |
Hyp. |
Nor. |
Hyp. |
Nor. |
Hyp. |
Data are presented as the means ± standard deviations (SD) of three repeated measurements.
|
Pt–Ru
|
2.80 ± 0.38 |
4.83 ± 0.42 |
5.40 ± 0.62 |
10.04 ± 0.76 |
11.63 ± 0.85 |
19.30 ± 2.40 |
Cisplatin |
6.74 ± 0.88 |
14.28 ± 1.25 |
8.83 ± 0.54 |
13.98 ± 1.21 |
49.70 ± 3.70 |
65.20 ± 3.10 |
Furthermore, the immunofluorescence assay using phosphorylated histone γ-H2AX as a DNA break marker also showed that Pt–Ru hybrids could trigger DNA damage effectively in both normoxic and hypoxic cells, and the effect was better than that of cisplatin under the same conditions (Fig. 5A). We also detected the distribution of Pt–Ru in living MCF-7 cells by ICP-MS (Fig. 5B). We found that the Pt content mainly distributes in the cytosol and nucleus while the Ru content mainly distributes in the cytosol and mitochondria. It was worth mentioning that after 36 h incubation the uptakes of Pt–Ru and cisplatin by MCF-7 cells were basically the same, but the cytotoxicity of Pt–Ru was ca. 3-fold higher than that of cisplatin, further verifying the chemo-catalytic synergistic therapy.
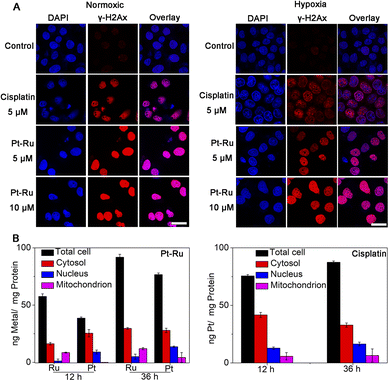 |
| Fig. 5 (A) Immunofluorescence of γ-H2AX (DNA breaks foci) in MCF-7 cells, scale bar = 20 μm; (B) distribution of Ru and Pt elements in MCF-7 cells after different incubation times with Pt–Ru and cisplatin (5 μM), respectively. | |
In vivo antitumor efficacy
Finally, we evaluated the antitumor efficacy of Pt–Ru hybrids in nude mice bearing MCF-7 tumors, the initial volumes of which were ca. 200 mm3. These mice were randomly divided into three groups (n = 3) and intravenously injected (i.v. 100 μL) with PBS (control), cisplatin (5 mg kg−1), and Pt–Ru (5 mg kg−1), respectively, only on the 1st day. Then the tumor volumes were measured every 3 days and all mice were sacrificed after 18 days for tumor collection. As shown in Fig. 6A and B, the tumor sizes in the control and cisplatin groups increased from 200 to 3280 and 1807 mm3, respectively, while Pt–Ru displayed much higher inhibitory activity against tumor growth where the tumor size only increased to 681 mm3. Meanwhile, Pt–Ru treated mice did not show obvious body weight loss while cisplatin caused 16% of weight loss during treatment, indicating minimum side effects (Fig. S12, ESI†). The immunofluorescence assay showed that Pt–Ru hybrids induced substantial DNA damage as indicated by the up-regulated γ-H2AX, as well as significant inhibition of HIF-1α expression in tumors tissues, the effect of which was much more apparent than that in the cisplatin group (Fig. 6C). These results demonstrated that Pt–Ru hybrids had excellent in vivo anti-tumor efficacy with hypoxia relieving capability.
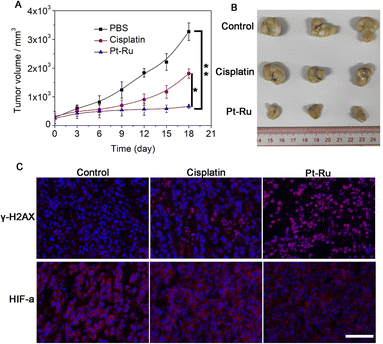 |
| Fig. 6
In vivo anti-tumor efficacy of PBS, cisplatin and Pt–Ru hybrids investigated following intravenous (i.v.) injection at a dose of 5 mg kg−1 or cisplatin-equivalent dose of 5 mg kg−1. (A) Changes in MCF-7 tumor volumes; (B) image of resected tumors; (C) expression of DNA damage marker (γ-H2AX) and HIF-1α in tumor tissues after 96 h of Pt–Ru hybrid treatment (5 mg kg−1, i.v). Scale bar = 100 μm. | |
Conclusions
In summary, we developed a Pt–Ru hybrid prodrug with multi-enzymatic activities (CAT, POD, and GPx) for efficient chemo-catalytic synergistic therapy of hypoxic tumors. The catalytic properties were mainly attributed to the ruthenium hybridization which endowed the novel Pt(IV) prodrug with redox homeostasis regulation capability, that is, alleviating hypoxia, producing excess ROS and depleting GSH simultaneously, which led to a superior therapeutic efficacy to cisplatin both in vitro and in vivo. This work suggested that endowing the drugs with multi-enzymatic activities would be a promising strategy for developing the next generation of platinum drugs.
Experimental section
Materials
Cisplatin and RuCl3 were both purchased from Bidepharm. Pluronic F-127 (F127, molecular weight = 10
000 g mol−1) was bought from Macklin reagent company. Succinic anhydride, 3,3′,5,5′-tetramethylbenzidine (TMB) and triethylamine were both purchased from Aladdin reagent company. 3-(4,5-Dimethylthiazol-2-yl)-2,5-diphenyltetrazolium bromide (MTT) was supplied by Sigma USA. Distilled water was purified by passing through a Millipore Milli-Q Biocel purification system (18.2 MΩ) with a 0.22 μm filter.
General instruments
UV-Vis spectra were monitored with a Varian Cary 100 UV/vis spectrophotometer (USA). Ru and Pt contents were measured on a Thermo X Series 2 Inductively Coupled Plasma Mass Spectrometer (Thermo Fisher Corp., USA). Transmission Electron Microscopy (T12, FEI Tecnai G2 Spirit, Holland) was performed at room temperature. Dynamic light scattering (DLS) studies were performed using a Zetasizer Nano instrument (EliteSizer, Bruker, Germany). The ruthenium and platinum contents were determined using an iCAP RQ. X-ray photoelectron spectra (XPS) were recorded on an S-Probe XPS spectrometer with Al Kα radiation as the X-ray source (1486 eV). Cell imaging experiments were carried out on a confocal microscope (Zeiss LSM-710/800, ZEISS, Germany). Flow cytometric analysis was done using a BD FACS Calibur™ flow cytometer (Becton Dickinson, USA).
Synthesis of [Pt(NH3)2Cl2(O2CCH2CH2CO2H)2]
The complex c,c,t-[Pt(NH3)2Cl2(OH)2] was synthesized by literature methods.41 Synthesis of [Pt(NH3)2Cl2(O2CCH2CH2CO2H)2] was carried out according to a previous report.42 Succinic anhydride (0.18 g, 1.8 mM) was added to a solution of c,c,t-[Pt(NH3)2Cl2(OH)2] (0.2 g, 0.6 mM) in dimethyl sulfoxide (DMSO, 5 mL). Then this solution was stirred at room temperature for 24 h under dark conditions. After the reaction, the solution was filtered and the precipitate was discarded, and then ultrapure water was added to the filtrate, followed by freeze-drying. A small amount of acetone was added to the lyophilized product for washing, and then the product was washed with ether and a small amount of methanol, obtaining the white product.
Fabrication of Pt–Ru nanoparticles
Pt–Ru nanoparticles were prepared by aqueous synthesis at room temperature. First, [Pt(NH3)2Cl2(O2CCH2CH2CO2H)2] (50 mg, 0.093 mM) was added to a 50 mL eggplant bottle and then 20 mL of water was added. This solution was stirred for 30 min at room temperature. Next 20 μL triethylamine was added slowly to the aqueous solution and stirred for 24 h. 20 mg RuCl3 was added to the above solution, and the solution was continuously stirred for 12 h, and then 10 mL of 50 mg F127 and 20 mg PVP solution was added, followed by continuous stirring for 12 h. The final product was obtained by centrifuging and washing with water. The contents of Ru and Pt in the product were determined by ICP-MS.
Effect of glutathione on the stability of Pt–Ru nanoparticles
20 μg mL−1 of Pt–Ru was dispersed in glutathione solution (5, 10 mM) and then incubated at 37 °C for 0, 1, 4, 12, 24 and 48 h respectively. The hydrodynamic diameter of Pt–Ru nanoparticles was measured at the above time. The stability of the nanoparticles was measured with 3 mL of water, PBS and 10% FBS water solution, and then, the particle size change of nanoparticles was measured every day for a total of 5 days.
Drug release study
0.5 mL of the Pt–Ru solution was transferred to a dialysis device (MWCO 8000–14000, Spectrum) that was immersed in pH 7.4 and 5.0 buffer solution and the GSH groups contained different concentrations of GSH (1, 5, 10 mM). After placing at 37 °C for 48 h, the Pt and Ru elements were examined by ICP-MS. The cumulative release of the Pt and Ru elements was calculated as follows: M release (%)= (Mc/M0) ×100%, where Mc is the amount of Ru or Pt released from Pt–Ru at time t and M0 is the amount of Ru or Pt elements in the Pt–Ru.
Decomposition of H2O2 by Pt–Ru and production of O2
H2O2 has UV-vis absorption at 240 nm, so the UV absorption was measured at 240 nm after the reaction to calibrate the concentration of H2O2. First, Pt–Ru (20 μg mL−1) was added to water solutions containing H2O2 (10 mM) at 37 °C. After incubation for different time intervals, the UV/Vis spectra of the residual H2O2 were recorded. The production of O2 from the reaction of H2O2 (1 mM) with different concentrations of Pt–Ru (20 μg) at 37 °C was detected by EPR measurements using the oxygen-sensitive spin-label probe CTPO (0.1 mM). The EPR spectra were recorded on a Bruker A300 X-band EPR spectrometer. The change of pH in the reaction process was measured with a handheld pH meter for a total of 30 minutes.
POD mimic activity of Pt–Ru
To investigate the POD mimic activity, different concentrations of TMB and H2O2 with Pt–Ru (20 μg mL−1) were combined at room temperature for 10 min in water solutions. The absorbance of TMB (650 nm) was recorded after 30 min of reaction time. The ˙OH was also monitored using the DMPO adduct in the EPR spectrometer.
Cell culture conditions
MCF-7 cells were obtained from the Experimental Animal Center of Sun Yat-Sen University (Guangzhou, China), and then maintained in DMEM medium containing 10% FBS, streptomycin (100 μg mL−1) and penicillin (100 U mL−1). A549R cells were purchased from Shanghai Zhong Qiao Xin Zhou Biotechnology Co. Ltd, and then maintained in 1640 medium containing 10% FBS, 20 μM Cisplatin streptomycin (100 μg mL−1) and penicillin (100 U mL−1). These cells were cultured in a humidified incubator in an atmosphere of 5% CO2 and 95% air at 37 °C. Hypoxic culture: cells were maintained at 37 °C in a humidified atmosphere containing 1% oxygen, 5% carbon dioxide and 94% nitrogen. Simulated hypoxic culture: 100 μM CoCl2 solution was used to simulate the hypoxia conditions according to ref. 17.
Cell uptake experiment and distribution
MCF-7 cells were seeded in a 10 cm dish and incubated for 24 h, and then treated with cisplatin and Pt–Ru (5 μM) for 12 h and 36 h. Then, the cells were washed three times with PBS and then the cells were collected and divided into three groups. The mitochondria and nucleus were extracted using a mitochondria extraction kit (Mitochondria Isolation Kit for Cell and Tissue, Yeasen Biotech) and nucleus extraction kit (Sangon Biotech (Shanghai) Co., Ltd) for the two groups of cells. The mitochondria, nucleus and another cell were added to a cell lysis buffer respectively to extract proteins. Then, a BCA protein quantification kit was used to quantify the protein. The Ru and Pt elements in the proteins were determined by ICP-MS.
Generation of O2 in MCF-7 cells
MCF-7 cells (1 × 105) were seeded into culture dishes and incubated at 37 °C for 12 h. Then, all those cells were incubated with [Ru(dpp)3]2Cl2 (0.1 μM in DMEM medium) for another 4 h, and then each dish was rinsed with PBS three times and further incubated with Pt–Ru solutions for 24 h. The cells with https://www.chembk.com/en/chem/N-Acetyl-L-cysteine (NAC, hydrogen peroxide scavenging reagent) treatment were used for study and the cells without treatment served as the control. Finally, all those cells were washed with DMEM medium three times and then captured using a CLSM under excitation at 488 nm; the emission was collected between 600 and 640 nm.
HIF-1α expression measured by immunofluorescence
After subculture, MCF-7 cells were laid in 12 well plates, and cell climbing sheets were placed in each hole of the plates in advance. Firstly, CoCl2 solutions were added into each well, and then Pt–Ru and cisplatin were added and the cells were incubated for 24 h. The cells were fixed with a 4% paraformaldehyde solution and permeabilized using 1% Triton X-100. After incubation with a blocking buffer (1 × PBS, 0.1% goat serum, 0.075% glycin) for 1 h at room temperature, the primary rabbit polyclonal antibody for HIF-1α was added and the cells were incubated at 4 °C for 24 h. The cells were then incubated with the secondary Alexa Fluor 488 goat anti-rabbit antibody for 4 h at 37 °C. After washing three times with PBS, the cells were captured using a confocal microscope (LSM 800, Carl Zeiss, Germany). Emission was collected at 520 nm on excitation at 488 nm.
MTT assay
MCF-7 and A549R cells were plated in 96 well plates. After 24 h of culture, different concentrations of cisplatin and Pt–Ru were added for 44 h incubation, and then MTT was added for another 4 h of incubation. For cytotoxicity under hypoxia, the cells should better be adapted to hypoxia 1 week in advance. Other experimental methods are the same as those under normal oxygen. Then the medium was removed and 150 μL of DMSO was added to each well. The cell viability was evaluated by measuring the absorbance at 595 nm (Infinite F200, Tecan, Switzerland).
Determination of intracellular ROS levels
MCF-7 cells were treated with different concentrations of Pt–Ru for 24 h. For the cells in the hypoxia group, a cobalt chloride solution was added at the same time, and other operations were the same as those in the normoxia group. Then, the cells were stained with 10 μM DCFH-DA for 20 min at 37 °C. After washing with PBS three times, the green fluorescence intensity of DCFH-DA was detected by flow cytometry.
Detection of hydroxyl radicals (˙OH) and superoxide anions (O2˙−) in cells
MCF-7 cells were seeded in a 35 mm dish and incubated for 24 h, and then treated with different concentrations of cisplatin and Pt–Ru for 36 h. The cells in the hypoxia group were treated with a cobalt chloride solution at the same time, and other operation methods were the same. Then, the cells were washed three times with PBS and then treated with a hydroxyl radical probe (hydroxyphenyl fluorescein, HPF) and superoxide anion probe (dihydroethidium, DHE) for 20 min. For the NAC group, 0.1 mM of NAC solution was added 2 h before dosing. After washing three times with PBS, the cells were captured using a confocal microscope (LSM 800, Carl Zeiss, Germany). Emission was collected at 520 nm on excitation at 488 nm.
Detection of intracellular GSH levels
The intracellular GSH level was measured using a GSH Assay Kit (Beyotime, China). MCF-7 cells were seeded in a 10 cm dish and incubated for 24 h, and then treated with different concentrations of cisplatin and Pt–Ru for 36 h. The cells were washed three times with PBS and centrifuged with a cell lysate to collect the supernatant. The absorption was recorded at 414 nm on an Infinite M200 pro microplate reader.
γ-H2AX expression measured by immunofluorescence
After subculture, MCF-7 cells were placed in 12 well plates. After incubation for 24 h, cisplatin and the drug were added respectively, and then the cells were incubated for 36 h. For the hypoxic group, a cobalt chloride solution was added while adding drugs. These cells were then fixed with 4% paraformaldehyde solution and permeabilized using 1% Triton X-100. After incubation with a blocking buffer (1 × PBS, 0.1% goat serum, 0.075% glycin) for 1 h at room temperature. The primary rabbit polyclonal antibody for γ-H2AX (2.5 μg mL−1 in blocking buffer) was added and the cells were incubated at 4 °C for 12 h. The cells were then incubated with the secondary Alexa Fluor 488 goat anti-rabbit antibody for 1 h at 37 °C. The cells were incubated with DAPI for 20 min at 37 °C. After washing three times with PBST (PBS with 1% Triton X-100), the cells were visualized using a confocal microscope (LSM 800, Carl Zeiss, Germany). For γ-H2AX, emission was collected at 520 nm upon excitation at 488 nm, and for DAPI, emission was collected at 470 nm on excitation at 405 nm.
In vivo anti-tumor therapy
All of the animal experiments were approved by the university animal care and use committee of Sun Yat-Sen University.
The female BALB/c-(nu/nu) nude mice aged 4–5 weeks were bought from Beijing Vitalriver Experimental Animal Technology Co. Ltd, under approval by Sun Yat-sen university laboratory animal center. All procedures used in this experiment were compliant with the local animal ethics committee. MCF-7 cells (2 × 106) were subcutaneously injected into the female mice. When the tumors grew to 200 mm3, those mice were divided into three groups and administered with an intravenous injection of PBS, cisplatin and Pt–Ru, respectively, on the 1st day. The tumor size was obtained using a Vernier caliper and the mice weight was measured every three days. The tumor volume was calculated by the formula: V = a2 × b/2, where a and b are the shortest and longest diameter of tumors. After 18 days of treatment, the mice were sacrificed and the major organs were collected for the enzyme linked immunosorbent assay (ELISA, Servicebio technology, Wuhan, China).
Statistical analysis
The significance of several experimental results was analyzed by using the analysis of variance (ANOVA) test. Probabilities p < 0.05 (*) and p < 0.002 (**), ***P < 0.001 were marked in figures and 0.05 was chosen as the significance level.
Author contributions
G. G. Y. synthesized and fully characterized all materials, and also did the animal experiment. B. B. L. performed the ICP-MS for the revised manuscript. Experimental data collection and analysis by X. X. S. and Z. Y. P. Q. C. designed the work and drafted the manuscript. Z. W. M. gave revision suggestions and writing guidance for the paper.
Conflicts of interest
There are no conflicts to declare.
Acknowledgements
This work was financially supported by the National Natural Science Foundation of China [21837006, 91953117, and 22177141], Natural Science Foundation of Guangdong Province [2021A1515010342], Science and Technology Program of Guangzhou [202201011718], and Fundamental Research Funds for Central Universities.
Notes and references
- B. Yang, Y. Chen and J. Shi, Adv. Mater., 2019, 31, 1901778 CrossRef PubMed.
- W. Wu, Y. Pu and J. Shi, Adv. Sci., 2021, 8, 2002816 CrossRef CAS PubMed.
- B. Yang, L. Ding, H. Yao, Y. Chen and J. Shi, Adv. Mater., 2020, 32, 1907152 CrossRef CAS.
- W. Wu, Y. Pu, X. Lu, H. Lin and J. Shi, Adv. Healthcare Mater., 2021, 10, 2001819 CrossRef CAS PubMed.
- X. Wang, X. Wang, S. Jin, N. Muhammad and Z. Guo, Chem. Rev., 2019, 119, 1138–1192 CrossRef CAS PubMed.
- M. Huo, L. Wang, Y. Chen and J. Shi, Nat. Commun., 2017, 8, 1–12 CrossRef CAS PubMed.
- M. L. Drakes and P. J. Stiff, Cancers, 2018, 10, 302–322 CrossRef PubMed.
- J. Chen, Y. Zhu, C. Wu and J. Shi, Chem. Soc. Rev., 2020, 49, 9057–9094 RSC.
- Y. Da I, C. Xu, X. Sun and X. Chen, Chem. Soc. Rev., 2017, 46, 3830–3852 RSC.
- C. C. Huang, W. T. Chia, M. F. Chung, K. J. Lin, C. W. Hsiao, C. Jin, W. H. Lim, C. C. Chen and H. W. Sung, J. Am. Chem. Soc., 2016, 5222–5225 CrossRef CAS.
- Y. You, Z. Zhao, L. He, Z. Sun, D. Zhang, C. Shi, Q. Cheng, Y. Liu, L. Luo and T. Chen, Adv. Funct. Mater., 2020, 30, 2002369 CrossRef CAS.
- X. Zhu, Y. Gong, Y. Liu, C. Yang and X. Qin, Biomaterials, 2020, 242, 119923 CrossRef CAS PubMed.
- C. Imberti, F. Lermyte, E. P. Friar, P. B. O'Connor and P. J. Sadler, Chem. Commun., 2021, 57, 7645–7648 RSC.
- H. Shi, C. Imberti, G. J. Clarkson and P. J. Sadler, Inorg. Chem. Front., 2020, 7, 3533–3540 RSC.
- J. Karges, T. Yempala, M. Tharaud, D. Gibson and G. Gasser, Angew. Chem., Int. Ed., 2020, 59, 7069–7075 CrossRef CAS.
- T. C. Johnstone, K. Suntharalingam and S. J. Lippard, Chem. Rev., 2016, 116, 3436–3486 CrossRef CAS PubMed.
- Q. Cao, D.-J. Zhou, Z.-Y. Pan, G.-G. Yang, H. Zhang, L.-N. Ji and Z.-W. Mao, Angew. Chem., Int. Ed., 2020, 59, 18556–18562 CrossRef CAS.
- Y. Yang, Y. Yu, H. Chen, X. Meng, W. Ma, M. Yu, Z. Li, C. Li, H. Liu, X. Zhang, H. Xiao and Z. Yu, ACS Nano, 2020, 14, 13536–13547 CrossRef CAS PubMed.
- P. A. Ma, H. Xiao, C. Yu, J. Liu, Z. Cheng, H. Song, X. Zhang, C. Li, J. Wang, Z. Gu and J. Lin, Nano Lett., 2017, 17, 928–937 CrossRef CAS PubMed.
- H. Chen, J. Tian, W. He and Z. Guo, J. Am. Chem. Soc., 2015, 137, 1539–1547 CrossRef CAS PubMed.
- J. Liu, Q. Chen, L. Feng and Z. Liu, Nano Today, 2018, 21, 55–73 CrossRef CAS.
- Q. Chen, L. Feng, J. Liu, W. Zhu, Z. Dong, Y. Wu and Z. Liu, Adv. Mater., 2016, 28, 7129–7136 CrossRef CAS PubMed.
- X. Fan, F. Yang, C. Nie, L. Ma, C. Cheng and R. Haag, Adv. Mater., 2021, 33, 2100637 CrossRef CAS PubMed.
- X. Xiao, S. Liang, Y. Zhao, M. Pang, P. a. Ma, Z. Cheng and J. Lin, Biomaterials, 2021, 277, 121120 CrossRef CAS PubMed.
- G.-G. Yang, Z.-Y. Pan, D.-Y. Zhang, Q. Cao, L.-N. Ji and Z.-W. Mao, ACS Appl. Mater. Interfaces, 2020, 12, 43444–43455 CrossRef CAS.
- H. Zhang, Y. Zhang, J. Cao, L. Ma and T. Chen, Chem. Commun., 2022, 58, 3759–3762 RSC.
- M. Xu, B. Xue, Y. Wang, D. Wang, D. Gao, S. Yang, Q. Zhao, C. Zhou, S. Ruan and Z. Yuan, Small, 2021, 17, 2101397 CrossRef CAS PubMed.
- L. Tong, C.-C. Chuang, S. Wu and L. Zuo, Cancer Lett., 2015, 367, 18–25 CrossRef CAS PubMed.
- C. Nathan and A. Cunningham-Bussel, Nat. Rev. Immunol., 2013, 13, 349–361 CrossRef CAS PubMed.
- S. Kwon, H. Ko, D. G. You, K. Kataoka and J. H. Park, Acc. Chem. Res., 2019, 52, 1771–1782 CrossRef CAS PubMed.
- S. Thota, D. A. Rodrigues, D. C. Crans and E. J. Barreiro, J. Med. Chem., 2018, 61, 5805–5821 CrossRef CAS PubMed.
- F. Heinemann, J. Karges and G. Gasser, Acc. Chem. Res., 2017, 50, 2727–2736 CrossRef CAS PubMed.
- M. Rausch, P. J. Dyson and P. Nowak-Sliwinska, Adv. Therap., 2019, 2, 1900042 CrossRef CAS.
- Z. Liu, L. Xie, K. Qiu, X. Liao, T. W. Rees, Z. Zhao, L. Ji and H. Chao, ACS Appl. Mater. Interfaces, 2020, 12, 31205–31216 CrossRef CAS PubMed.
- C. Wu, X. Han, W. Feng, Z. Liu, L. Chen, B. Zhou, Y. Chen and J. Shi, Chem. Eng. J., 2021, 411, 128543 CrossRef CAS.
- H. Deng, W. Shen, Y. Peng, X. Chen, G. Yi and Z. Gao, Chem.–Eur. J., 2012, 18, 8906–8911 CrossRef CAS PubMed.
- S.-B. He, P. Balasubramanian, Z.-W. Chen, Q. Zhang, Q.-Q. Zhuang, H.-P. Peng, H.-H. Deng, X.-H. Xia and W. Chen, ACS Appl. Mater. Interfaces, 2020, 12, 14876–14883 CrossRef CAS PubMed.
- X. Zhu, X. Chen, Z. Jia, D. Huo, Y. Liu and J. Liu, J. Colloid Interface Sci., 2021, 603, 615–632 CrossRef CAS PubMed.
- E. Ruggiero, J. Hernández-Gil, J. C. Mareque-Rivas and L. Salassa, Chem. Commun., 2015, 51, 2091–2094 RSC.
- W. Zhen, Y. Liu, L. Lin, J. Bai, X. Jia, H. Tian and X. Jiang, Angew. Chem., Int. Ed., 2018, 57, 10309–10313 CrossRef CAS PubMed.
- M. Hall, C. Dillon, M. Zhang, P. Beale, Z. Cai, B. Lai, A. J. Stampfl and T. Hambley, J. Biol. Inorg Chem., 2003, 8, 726–732 CrossRef CAS.
- K. R. Barnes, A. Kutikov and S. J. Lippard, Chem. Biol., 2004, 11, 557–564 CrossRef CAS PubMed.
|
This journal is © The Royal Society of Chemistry 2022 |
Click here to see how this site uses Cookies. View our privacy policy here.