DOI:
10.1039/D2SC04168E
(Edge Article)
Chem. Sci., 2022,
13, 13147-13152
Cyclic arrays of five pyrenes on one rim of a planar chiral pillar[5]arene†
Received
26th July 2022
, Accepted 24th October 2022
First published on 24th October 2022
Abstract
Spatial arrangement of multiple planar chromophores is an emerging strategy for molecule-based chiroptical materials via easy and systematic synthesis. We attached five pyrene planes to a chiral macrocycle, pillar[5]arene, producing a set of chiroptical molecules in which pyrene-derived absorption and emission were endowed with dissymmetry by effective transfer of chiral information. The chiroptical response was dependent on linker structures and substituted patterns because of variable interactions between pyrene units. One of these hybrids showed larger dissymmetry factor and response wavelength (glum = 7.0 × 10−3 at ca. 547 nm) than reported pillar[5]arene-based molecules using the pillar[5]arene cores as parts of photo-responsive π-conjugated units.
Introduction
Chirality occurs widely in nature, enabling sophisticated folding or assembling structures and recognition systems that are essential for biological activities. Chirality is also a key for advanced materials because of characteristic functions caused by symmetry breaking. Among them, interaction between chiral compounds and circularly polarized light is of prime importance. Much attention is directed to circular dichroism (CD) and circularly polarized luminescence (CPL) of newly synthesized compounds with diverse structures.1 Organic π-conjugated molecules are attractive in terms of strong π–π* electronic transitions as well as lightweight, flexible and soluble nature.2 However, they are mostly achiral due to planar structures and need to be endowed with chirality by structural modification.
Contorting planar π-conjugated skeletons into non-planar ones is a major protocol to attain strong chiroptical response. Helicenes3 are one of the most representative classes of compounds and have been extensively studied (Fig. 1a), including derivatives with controlled symmetry4,5 and electric and magnetic transition dipole moments (μ and m).6 Recently, macrocyclic compounds have also attracted intensive research,7 one of which improved the record of CPL dissymmetry factors (glum)‡ in organic molecules.8 Cyclic structures were indicated to be effective for the development of chiroptical molecules because they had appropriate symmetry and an increased ratio of |m| to |μ|, which is typically small in organic compounds without d- and f-electrons. Although these platforms offer appealing electronic systems with smooth π-conjugation, the synthesis requires construction of distorted structures and in some cases fused structures via multiple bond formation.
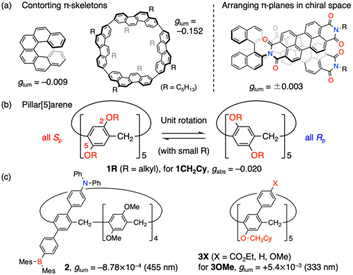 |
| Fig. 1 (a) Two key strategies for chiroptical molecules. (b) Chemical structure of a pillar[5]arene and (c) its modification toward CPL-active derivatives. | |
Spatial arrangement of π-conjugated planes is another strategy for chiroptical molecules.2,9–12 In these systems, π-conjugated units are simply connected to chiral centres with single bonds or aggregate into chiral assembly using non-covalent interactions. These characteristics are suitable for systematic structural alteration, enabling easy tuning of response wavelength and solubility. The less rigid structures also allow them to switch the chiroptical properties in response to external stimuli.9b,d,12 On the other hand, it is relatively difficult to obtain high g values with this approach as compared with contorted π-conjugated systems because strong π-conjugation was unavailable for inducing chirality. To transmit chiral information effectively to photo-responsive π-conjugated units, π-stacked dimers in chiral arrangements were extensively used.9a,c,11 π-Stacked pyrene dimers with excimer emission is one of the most investigated systems9a for elaborate stimuli-responsive systems. Meanwhile, molecular designs for strong chiroptical response were rather unexplored. Chiral π-stacked dimers of pyrene were piled up by integrating them with chiral oligo-naphthyl backbones and helical polymers13 or forming one-dimensional supramolecular assemblies.14 However, ideas applied in the designs of contorted π-conjugated molecules, such as forming chiral cyclic structures, have not been fully exploited in these π-stacked systems, except the latest example using cyclodextrins.15 The report attained highly efficient CD and CPL induction, while both left- and right-handed responses were attained by tuning linker units as only one chirality is available for cyclodextrins. Hence, we envisage arranging multiple pyrene units on a rim of pillar[5]arene, a cylindrical macrocyclic molecule.
Pillar[5]arenes 1R (Fig. 1b)16,17 are constructed from paraphenylene units linked with methylene groups. Each phenylene unit is usually substituted with alkoxy groups at the 2,5-positions and exhibits planar chirality. With small and flexible alkoxy groups, the π-units can rotate with inversion of the chirality, which affords equilibrium state between energetically preferred all-Sp or all-Rp conformations.18 On the other hand, two enantiomers are successfully separated when bulky substituents are attached onto both rims.19 Although enantiopure pillar[5]arenes themselves have good chiroptical properties (gabs: 10−2 order), they are not suitable for CPL materials because of poorly fluorescent nature and short response wavelength (<320 nm). To circumvent these drawbacks, several functionalized pillar[5]arenes were developed (Fig. 1c).20–22 In 2020, Chen and co-workers introduced amine and borane substituents into one unit of a pillar[5]arene and attained CPL at around 455 nm (2).20a However, the emission largely relied on the functionalized terphenyl unit and hence limited chiral transfer from the chiral macrocycle core resulted in 10−4 order of glum value. We reported a set of rim-differentiated pillar[5]arenes 3X with improved dissymmetry based on retained C5-symmetry, but the twisted biphenyl units gave chiroptical response at around 400 nm or less.21 A rational solution to these drawbacks is hybrids with planar π-conjugated units such as pyrene,23 in which a pillar[5]arene served solely as a chiral C5-symmetric platform. Indeed, a hybrid molecule in the present work exhibited glum of −7.1 × 10−3 at around 550 nm, derived from a chiral pyrene cluster. The luminescence properties were also dependent on the linker segments and substituents on pyrene rings.
Results and discussion
Synthesis and characterization
Synthesis.
Pillar[5]arene scaffold 9 was prepared according to the reported procedure.21,24 As an initial attempt, the SN2 reaction with 1-(bromomethyl)pyrene was tested with Cs2CO3 in a mixture of N,N-dimethylformamide (DMF) and toluene at 110 °C. Although the target compound was observed in the 1H NMR spectrum, the crude mixture contained large amounts of side products because of the incomplete 5-fold reaction. After several experiments, NaH turned out to be effective but still the target product could not be isolated by gel permeation chromatography (GPC) and repeated recrystallization. The unfunctionalized pyrene segments led to poor solubility in n-hexane and low polarity, which made silica gel chromatography useless for purification.
With this partial success in mind, we envisioned incorporation of ester groups on pyrene periphery (Scheme 1). Butyl ester was selected due to its adequate polarity, solubilizing ability and π-stacking character derived from small bulkiness and weakly electron-withdrawing nature. After ester formation with 1-bromobutane,25 a combination of dichloromethyl methyl ether and TiCl4 enabled to append a formyl group.26 After reduction of formyl groups into hydroxymethyl groups with NaBH4,27 three isomers were separated using a high-performance liquid chromatography (HPLC) system with a chiral column. Assignment of isomers 7a–c was conducted based on their NMR spectra including two-dimensional correlation measurement (for details, see Tables S3-1 and S3-2, Fig. S3-3 to S3-5, S3-12 to S3-16 in the ESI†). Final bromination with PBr3 furnished ester-appended (bromomethyl)pyrenes 8a–c. The SN2 reaction of pillar[5]arene 9 with 8a–c was conducted under the conditions optimized with simple (bromomethyl)pyrene. While 1,3-substituted pyrene did not provide the corresponding product at all probably because of large steric hindrance, use of 1,6- and 1,8-derivatives led to smooth reaction due to improved solubility of multi-pyrene products. Since remaining 8b and 8c overlapped on silica gel columns, products 10b and 10c were obtained as pairs of pure enantiomers in 28–29% total yields after chiral HPLC separation (Fig. S6-1†). To examine effects of spacer length between pillar[5]arene and pyrene moieties, 12 was synthesized with 1-(bromoacetyl)pyrene in 10% yield. The solubility of 12 was not very high and hence purification was performed by GPC and recrystallization from CH2Cl2/n-hexane. Because of the inserted carbonyl groups with moderate polarity, 12 was successfully separated into enantiomers using a chiral column.
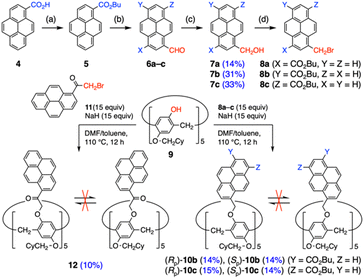 |
| Scheme 1 Introduction of pyrene units on one rim of a pillar[5]arene with cyclohexylmethoxy stoppers. Reagents and conditions: (a) 1-bromobutane (1.5 equiv.), K2CO3 (2.0 equiv.), DMF, 80 °C, 26 h, quant.; (b) MeOCHCl2 (1.25 equiv.), TiCl4 (1.25 equiv.), CH2Cl2, 0 °C to RT, 1 h, 46% as mixture; (c) NaBH4 (0.26 equiv.), CH2Cl2/MeOH, −20 °C, 1 h, total 78%; (d) PBr3 (1.2 equiv.), toluene, 0 °C to RT, 1 h, 44–47%. | |
NMR spectroscopy.
Because of bulky substituents on both rims of pillar[5]arenes, pyrene-arrayed pillar[5]arenes 10b, 10c, and 12 displayed sets of C5-symmetric signals in the 1H NMR spectra (Fig. S3-9 to S3-11†). Clear peak splitting was observed for methylene protons in the pillar[5]arene cores and pendent OCH2-moieties on both rims, indicating restricted unit rotation.18b Pyrenyl protons appeared at 8.80–6.87 and 9.00–7.55 ppm in 10b and 10c respectively, which were largely up-field shifted compared with those in 8b (9.23–8.03 ppm) and 8c (9.38–8.00 ppm). The peak shifts were mainly ascribed to aromatic shielding by a pyrene ring in the next unit, suggesting the proximity of pyrene planes suitable for effective interactions.§ Two protons in 12 were much more shielded (6.00 and 5.89 ppm), which implied the existence of CH/π interactions.
Optical properties
UV/vis absorption and CD spectra.
Fig. 2a presents the UV/vis absorption and CD spectra of 10b, 10c and 12 in CHCl3. Because of five pyrene segments, the UV/vis absorption spectra of 10b and 10c were composed of two major bands at 286 and 358 nm with a side peak at 389 nm. In common per-alkoxy-substituted pillar[5]arenes, broad UV absorption was observed at around 300 nm, which was not apparent in 10b and 10c by overlapping with the shoulder region of the intense 286 nm peak. In contrast, CD signals due to pillar[5]arene cores were clearly observed at 310 nm with |Δε| ∼ 120 M−1 cm−1 and |gabs| ∼ 4 × 10−3 (Table 1). The |gabs| values were smaller than that of the per-cyclohexylmethoxy-substituted derivative (|gabs| = 20 × 10−3)19 because of dilution by the weakly dissymmetric pyrene-based absorption. From the positive Cotton effect of this peak, the 1st fractions of 10b and 10c were assigned as Rp-forms and the 2nd fractions as Sp-forms. CD intensities in the long-wavelength range were also high for 10b (|Δε| = 83 M−1 cm−1 and |gabs| = 1.0 × 10−3 at 372 nm) and moderate for 10c with some broadening (|Δε| = 40 M−1 cm−1 and |gabs| = 0.3 × 10−3 at 361 nm). These values indicated that chiral information was transferred effectively from the pillar[5]arene to pyrene moieties in these hybrid molecules.
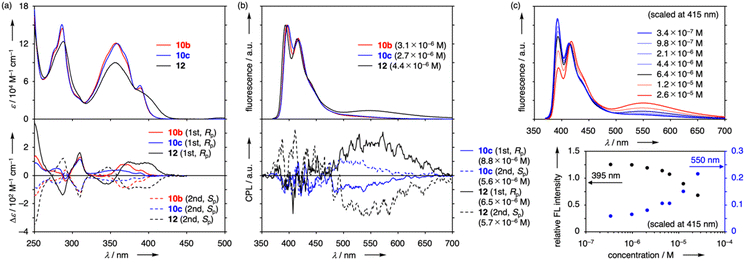 |
| Fig. 2 (a) UV/vis absorption (top) and CD (bottom) spectra of 10b, 10c and 12 in CHCl3. (b) Fluorescence (top, λex = 350 nm) and CPL (bottom, λex = 280 nm) spectra of 10b, 10c and 12 in CHCl3. (c) Concentration-dependent fluorescence spectra of 12 in CHCl3 (top) and relative intensity plot (bottom) of peaks at 395 nm (black, left axis) and 550 nm (blue, right axis) scaled at 415 nm. | |
Table 1 Summary of chiroptical properties in CHCl3
|
λ
abs [nm] (|gabs| × 103) |
Φ
lum
|
λ
lum
[nm] (|glum| × 103) |
λ
ex = 350 nm.
λ
ex = 280 nm.
4.4 × 10−6 M.
5.7–6.5 × 10−6 M.
|
10b
|
310 (4.0) |
372 (1.0) |
0.13 |
(Not detected) |
10c
|
310 (3.6) |
361 (0.3) |
0.15 |
ca. 500 (3.2) |
12
|
310 (4.6) |
373 (1.5) |
0.01c |
ca. 547 (7.0)d |
In 12, unfunctionalized pyrene rings were separated by longer spacer segments with additional carbonyl groups, but the UV/vis and CD spectra of 12 displayed roughly the same shapes as those of 10b and 10c. As minor differences, UV/vis absorption of 12 were broader to extend the tail over 430 nm probably due to the existence of several conformations with different interactions between pyrene units (vide infra). Additional CD peaks with the opposite sign were also evident at both high- and low-energy sides of the 310 nm signal. The pyrene-based CD band of 12 was the most intense (|Δε| = 97 M−1 cm−1 at 373 nm) among the three and reached almost the same dissymmetry as the pillar[5]arene-derived band in the long-wavelength shoulder region (|gabs| = 2.7 × 10−3 at 406 nm).
Fluorescence and CPL spectra.
Fluorescence spectra of 10b and 10c in CHCl3 were composed of single bands with clear vibronic structures at around 400 nm, which can be assigned to emission from locally excited states (Fig. 2b). On the other hand, that of 12 additionally had a weak and broad signal at 500–700 nm. Such bands were almost negligible in the cases of 10b and 10c, which reflected the structural differences of spacer lengths. When the excitation wavelength was altered from 350 nm to 370 and 390 nm, 10b and 10c exhibited almost identical spectra to that with 350 nm excitation except for changes in ratios of two peak top intensities (Fig. S5-2†). The spectral shape of 12 varied with small red shifts upon excitation at longer wavelengths, while the intensity at around 547 nm was roughly retained. These results suggested that 10b and 10c took nearly single stable conformation, changed between multiple conformations fast or showed similar fluorescence regardless of conformational differences. In contrast, it was assumed that two or more conformers contributed to fluorescence behaviour of 12, indicating again the significance of spacer structures.
To characterize fluorescent species, excitation spectra were measured for all the peak top wavelengths of 10b, 10c and 12 (Fig. S5-3 and S5-4†). While each spectrum of 10b and 10c displayed shape like its UV/vis absorption spectrum, that of 12 did not overlap well. Upon detection at 395 and 415 nm, the spectral shape of 12 appeared sharp like those of 10b and 10c and it was highly broadened with 550 nm detection. These results implied that the UV/vis absorption spectrum was understood as the sum of two types of excitation spectra and short- and long-wavelength emission were mainly derived from different ground-state conformations. Excitation spectra were also acquired at 500 nm, which corresponded to the tail region of single-pyrene emission. The spectrum of 12 was similar to that at 550 nm, reflecting large contribution of the conformer with broad UV/vis absorption. The spectra of 10b and 10c were not as broad as that of 12 but were significantly deviated from excitation spectra at 397 and 417 nm. The broadening indicated the presence of minor conformations in 10b and 10c with broad absorption though their populations were too small to be detected in usual UV/vis and fluorescence spectra. Further, two types of species were characterized by emission lifetime measurement (Fig. S5-5 and S5-6, Table S5-1†). At wavelengths of single-pyrene emission, all the hybrids showed lifetimes of 4.3–4.6 ns along with minor contributions with shorter decay constants (0.30–2.3 ns). At 500 nm, 10b and 10c had additional components of 22 and 19 ns respectively, which indicated the presence of excimer emission in the tail regions. In the case of 12, fitting for decay at 500 and 550 nm was rather difficult owing to multiple conformations but included components with longer lifetime (>7.0 ns) assignable to excimer emission. These results demonstrated that conformations with broad absorption led to excimer emission in tail regions more efficiently than those with sharp absorption.
Then, concentration effects on emission properties were investigated. While both UV/vis and fluorescence spectra of 10b and 10c were not concentration-dependent, the fluorescence spectrum of 12 changed its shape in response to concentration with its UV/vis, CD, and 1H NMR spectral shape unchanged (Fig. S3-18, S5-7 and S6-4†). These results showed the absence of ground-state aggregates. As the concentration increased over 10−6 M, relative intensity at 395 nm dropped as compared with that of 415 nm and that at 550 nm gradually increased (Fig. 2c). Therefore, long-wavelength emission at around 550 nm was accounted for mainly by intermolecularly formed pyrene excimers. On the other hand, nearly constant intensity (relative intensity of ca. 5%) at 3.4 × 10−7 M was due to intramolecular excimers. Large contribution of intermolecular excimers implied that most conformations of 12 did not easily take conformations suitable for intramolecular excimer states but were able to form intermolecular π-stacked dimers. On the other hand, absence of concentration-dependence in the fluorescence spectra of 10b and 10c indicated that intermolecular excimers were not accessible from 10b and 10c probably because of appended ester groups and long-lifetime components in the tail region could be accounted for solely by intramolecular excimers.
CPL spectra were also recorded for enantiopure fractions of 10b, 10c and 12 in CHCl3 (Fig. 2b). Notably, single pyrene-based emission at around 400 nm was CPL-silent for all the compounds despite intense CD response and only excimer-based emission showed evident CPL signals. Along this line, 12 displayed a pair of mirror-image CPL at 480–680 nm. The observed |glum| value at around 547 nm peak top (7.0 × 10−3 at 5.7–6.5 × 10−6 M) was the highest among pillar[n]arene single molecules in solution, though the luminescence quantum yield was low (Φlum = 0.01).¶ The |glum| value was also concentration-dependent and increased from 2.5 × 10−3 at 2.7 × 10−7 M to 8.4 × 10−3 at 2.4 × 10−5 M, which indicated that CPL signals were composed of both intramolecular and intermolecular excimer emission and the latter possessed larger dissymmetry factor. Curiously, CPL was not detected for 10b at 350–800 nm but 10c displayed CPL signals at 470–600 nm (|glum| = 3.2 × 10−3 at around 500 nm), which corresponded to the tail region with small contribution of intramolecular excimers in the fluorescence spectrum.
Conclusions
Multiple π-conjugated planes were arranged on a chiral macrocycle by using functionalized pyrenes and a pillar[5]arene with cyclohexylmethoxy groups on the other rim, via 5-fold SN2 reactions of a phenolic pillar[5]arene. In these hybrids, fixed chiral information of the pillar[5]arene was effectively transmitted to the assemblies of pyrene units, leading to as intense CD signals at 350–400 nm as that at 310 nm due to the per-alkoxy-substituted pillar[5]arene. Pyrene-derived emission bands were composed of single-pyrene fluorescence at around 400 nm with vibronic structures and excimer emission at 500–550 nm in the tail region. The former was silent in CPL response while the latter displayed 10−3 order of CPL signals. The excimer formation was effective with a three-atom linker as compared with two-atom ones and was sterically hampered when substituents on pyrene units were located inside the macrocycle. Without additional substituents on pyrenes, intramolecular excimer formation competed with the intermolecular process, offering concentration-dependent fluorescence properties.
In this work, a high |glum| value of 7.0 × 10−3 at around 550 nm was attained based on the hybrid of a chiral macrocycle and π-conjugated planes. Although the luminescence quantum yield was low, the obtained CPL response demonstrated a useful design principle for CPL-active pillar[5]arene molecules. Further studies on chiroptical pillar[n]arenes are underway in our group.
Data availability
The datasets supporting this article have been uploaded as the ESI.†
Author contributions
K. T. and T. O. supervised and administered this project. K. K. performed conceptualization. K. K., S. O. and M. G. conducted the experiments and theoretical calculations. K. K. prepared the draft and initial version of the ESI,† which were reviewed and edited by all authors.
Conflicts of interest
There are no conflicts to declare.
Acknowledgements
This work was supported by JSPS KAKENHI Grant Numbers JP20K22528 (Research Activity Start-up, K. K.), JP21K14611 (Early-Career Scientists, K. K.), JP21K20533 (Research Activity Start-up, S. O.), JP18H04510 and JP20H04670 (Scientific Research on Innovative Areas, T. O.) and JP19H00909 and JP22H00334 (Scientific Research (A), T. O.), JST CREST Grant Number JPMJCR18R3 (T. O.) and the MEXT World Premier International Research Center Initiative (WPI), Japan.
Notes and references
- Reviews on CD, ORD, and CPL:
(a) J. A. Schellman, Chem. Rev., 1975, 75, 323 CrossRef CAS;
(b) F. S. Richardson and J. P. Riehl, Chem. Rev., 1977, 77, 773 CrossRef CAS;
(c) J. P. Riehl and F. S. Richardson, Chem. Rev., 1986, 86, 1 CrossRef CAS.
- Reviews on organic molecules and their assembly with strong chiroptical response:
(a) E. M. Sanchez-Carnerero, A. R. Agarrabeitia, F. Moreno, B. L. Maroto, G. Muller, M. J. Ortiz and S. de la Moya, Chem.–Eur. J., 2015, 21, 13488 CrossRef CAS PubMed;
(b) J. Kumar, T. Nakashima and T. Kawai, J. Phys. Chem. Lett., 2015, 6, 3445 CrossRef CAS PubMed;
(c) E. Yashima, N. Ousaka, D. Taura, K. Shimomur, T. Ikai and K. Maeda, Chem. Rev., 2016, 116, 13752 CrossRef CAS PubMed.
-
(a) R. H. Martin, Angew. Chem., Int. Ed., 1974, 13, 649 CrossRef;
(b) Y. Shen and C.-F. Chen, Chem. Rev., 2012, 112, 1463 CrossRef CAS PubMed;
(c) K. Dhbaibi, L. Favereau and J. Crassous, Chem. Rev., 2019, 119, 8846 CrossRef CAS PubMed.
- T. Mori, Chem. Rev., 2021, 121, 2373 CrossRef CAS PubMed.
-
(a) H. Tanaka, M. Ikenosako, Y. Kato, M. Fujiki, Y. Inoue and T. Mori, Commun. Chem., 2018, 1, 38 CrossRef;
(b) H. Tanaka, Y. Kato, M. Fujiki, Y. Inoue and T. Mori, J. Phys. Chem. A, 2018, 122, 7378 CrossRef CAS PubMed.
- Donor–acceptor-type helicenes:
(a) H. Kubo, T. Hirose and K. Matsuda, Org. Lett., 2017, 19, 1776 CrossRef CAS PubMed;
(b) H. Kubo, T. Hirose, T. Nakashima, T. Kawai, J.-y. Hasegawa and K. Matsuda, J. Phys. Chem. Lett., 2021, 12, 686 CrossRef CAS PubMed;
(c) H. Kubo, T. Hirose, D. Shimizu and K. Matsuda, Chem. Lett., 2021, 50, 804 CrossRef CAS.
- M. Hasegawa, Y. Nojima and Y. Mazaki, ChemPhotoChem, 2021, 5, 1042 CrossRef CAS.
- S. Sato, A. Yoshii, S. Takahashi, S. Furumi, M. Takeuchi and H. Isobe, Proc. Natl. Acad. Sci. U. S. A., 2017, 114, 13097 CrossRef CAS PubMed.
-
(a) Y. Ohishi and M. Inouye, Tetrahedron Lett., 2019, 60, 151232 CrossRef CAS;
(b) J.-L. Ma, Q. Peng and C.-H. Zhao, Chem.–Eur. J., 2019, 25, 15441 CrossRef CAS PubMed;
(c) Y. Imai, Chem. Lett., 2021, 50, 1131 CrossRef CAS;
(d) J. Zhao and P. Xing, ChemPhotoChem, 2021, 6, e202100124 Search PubMed.
-
(a) N. Harada and K. Nakanishi, J. Am. Chem. Soc., 1969, 91, 3989 CrossRef CAS;
(b) N. Harada, Y. Takuma and H. Uda, J. Am. Chem. Soc., 1976, 98, 5408 CrossRef CAS.
-
(a) T. Kawai, K. Kawamura, H. Tsumatori, M. Ishikawa, M. Naito, M. Fujiki and T. Nakashima, ChemPhysChem, 2007, 8, 1465 CrossRef CAS PubMed;
(b) K. Nakabayashi, T. Amako, N. Tajima, M. Fujiki and Y. Imai, Chem. Commun., 2014, 50, 13228 RSC;
(c) H. Ito, H. Sakai, Y. Okayasu, J. Yuasa, T. Mori and T. Hasobe, Chem.–Eur. J., 2018, 24, 16889 CrossRef CAS PubMed.
-
(a) P. Reiné, J. Justicia, S. P. Morcillo, S. Abbate, B. Vaz, M. Ribagorda, Á. Orte, L. Álvarez de Cienfuegos, G. Longhi, A. G. Campaña, D. Miguel and J. M. Cuerva, J. Org. Chem., 2018, 83, 4455 CrossRef PubMed;
(b) Y. Hashimoto, T. Nakashima, J. Kuno, M. Yamada and T. Kawai, ChemNanoMat, 2018, 4, 815 CrossRef CAS;
(c) A. Homberg, E. Brun, F. Zinna, S. Pascal, M. Gorecki, L. Monnier, C. Besnard, G. Pescitelli, L. D. Bari and J. Lacour, Chem. Sci., 2018, 9, 7043 RSC;
(d) K. Takaishi, K. Iwachido and T. Ema, J. Am. Chem. Soc., 2020, 142, 1774 CrossRef CAS PubMed;
(e) S. Ito, R. Sekine, M. Munakata, M. Asami, T. Tachikawa, D. Kaji, K. Mishima and Y. Imai, ChemPhotoChem, 2021, 5, 920 CrossRef CAS;
(f) H. Nian, L. Cheng, L. Wang, H. Zhang, P. Wang, Y. Li and L. Cao, Angew. Chem., Int. Ed., 2011, 60, 15354 CrossRef PubMed.
-
(a) K. Takaishi, R. Takehana and T. Ema, Chem. Commun., 2018, 54, 1449 RSC;
(b) K. Takaishi, K. Iwachido, R. Takehana, M. Uchiyama and T. Ema, J. Am. Chem. Soc., 2019, 141, 6185 CrossRef CAS PubMed;
(c) K. Takaishi, S. Murakami, K. Iwachido and T. Ema, Chem. Sci., 2021, 12, 14570 RSC;
(d) Y. Nagata, T. Nishikawa and M. Suginome, Chem. Commun., 2012, 48, 11193 RSC.
-
(a) J. Xiao, J. Xu, S. Cui, H. Liu, S. Wang and Y. Li, Org. Lett., 2008, 10, 645 CrossRef CAS PubMed;
(b) A. L. Nussbaumer, D. Studer, V. L. Malinovskii and R. Häner, Angew. Chem., Int. Ed., 2011, 50, 5490 CrossRef CAS PubMed;
(c) M.-C. Li, H.-F. Wang, C.-H. Chiang, Y.-D. Lee and R.-M. Ho, Angew. Chem., Int. Ed., 2014, 53, 4450 CrossRef CAS PubMed;
(d) Z. Wang, A. Hao and P. Xing, Angew. Chem., Int. Ed., 2020, 59, 11556 CrossRef CAS PubMed.
- H. Shigemitsu, K. Kawakami, Y. Nagata, R. Kajiwara, S. Yamada, T. Mori and T. Kida, Angew. Chem., Int. Ed., 2022, 61, e202114700 CrossRef CAS PubMed.
- T. Ogoshi, S. Kanai, S. Fujinami, T.-a. Yamagishi and Y. Nakamoto, J. Am. Chem. Soc., 2008, 130, 5022 CrossRef CAS PubMed.
- Reviews and a book on pillar[n]arenes:
(a) P. J. Cragg and K. Sharma, Chem. Soc. Rev., 2012, 41, 597 RSC;
(b) M. Xue, Y. Yang, X. Chi, Z. Zhang and F. Huang, Acc. Chem. Res., 2012, 45, 1294 CrossRef CAS PubMed;
(c) N. L. Stutt, H. Huacheng, S. T. Schneebeli and J. F. Stoddart, Acc. Chem. Res., 2014, 47, 2631 CrossRef PubMed;
(d) K. Yang, Y. Pei, J. Wen and Z. Pei, Chem. Commun., 2016, 52, 9316 RSC;
(e) T. Ogoshi, T.-a. Yamagishi and Y. Nakamoto, Chem. Rev., 2016, 116, 7937 CrossRef CAS PubMed;
(f) S. Fa, T. Kakuta, T.-a. Yamagishi and T. Ogoshi, Chem. Lett., 2019, 48, 1278 CrossRef CAS;
(g) J.-F. Chen, J.-D. Ding and T.-B. Wei, Chem. Commun., 2021, 57, 9029 RSC;
(h)
Pillararene, ed. T. Ogoshi, The Royal Society of Chemistry, Cambridge, U.K., 2016 Search PubMed.
-
(a) T. Ogoshi, K. Kitajima, T. Aoki, S. Fujinami, T.-a. Yamagishi and Y. Nakamoto, J. Org. Chem., 2010, 75, 3268 CrossRef CAS PubMed;
(b) K. Du, P. Demay-Drouhard, K. Samanta, S. Li, T. U. Thikekar, H. Wang, M. Guo, B. v. Lagen, H. Zuilhof and A. C.-H. Sue, J. Org. Chem., 2020, 85, 11368 CrossRef CAS PubMed.
- T. Ogoshi, K. Masaki, R. Shiga, K. Kitajima and T.-a. Yamagishi, Org. Lett., 2011, 13, 1264 CrossRef CAS PubMed.
-
(a) J.-F. Chen, X. Yin, B. Wang, K. Zhang, G. Meng, S. Zhang, Y. Shi, N. Wang, S. Wang and P. Chen, Angew. Chem., Int. Ed., 2020, 59, 11267 CrossRef CAS PubMed;
(b) H. Zhu, Q. Li, B. Shi, H. Xing, Y. Sun, S. Lu, L. Shangguan, X. Li, F. Huang and P. J. Stang, J. Am. Chem. Soc., 2020, 142, 17340 CrossRef CAS PubMed;
(c) J.-F. Chen, X. Yin, K. Zhang, Z. Zhao, S. Zhang, N. Zhang, N. Wang and P. Chen, J. Org. Chem., 2021, 86, 12654 CrossRef CAS PubMed.
- K. Kato, Y. Kurakake, S. Ohtani, S. Fa, M. Gon, K. Tanaka and T. Ogoshi, Angew. Chem., Int. Ed., 2022, 61, e202209222 CrossRef CAS PubMed.
- Chiroptical supramolecular systems using pillar[n]arenes:
(a) W.-J. Li, Q. Gu, X.-Q. Wang, D.-Y. Zhang, Y.-T. Wang, X. He, W. Wang and H.-B. Yang, Angew. Chem., Int. Ed., 2021, 60, 9507 CrossRef CAS PubMed;
(b) S. Fa, T. Tomita, K. Wada, K. Yasuhara, S. Ohtani, K. Kato, M. Gon, K. Tanaka, T. Kakuta, T.-a. Yamagishi and T. Ogoshi, Chem. Sci., 2022, 13, 5846 RSC.
- Pyrene units were incorporated into pillar[n]arene racemates:
(a) N. L. Strutt, R. S. Forgan, J. M. Spruell, Y. Y. Botros and J. F. Stoddart, J. Am. Chem. Soc., 2011, 133, 5668 CrossRef CAS PubMed;
(b) T. Ogoshi, R. Shiga, M. Hashizume and T.-a. Yamagishi, Chem. Commun., 2011, 47, 6927 RSC;
(c) T. Ogoshi, D. Yamafuji, D. Kotera, T. Aoki, S. Fujinami and T.-a. Yamagishi, J. Org. Chem., 2012, 77, 11146 CrossRef CAS PubMed;
(d) T. Ogoshi, D. Yamafuji, T.-a. Yamagishi and A. M. Brouwer, Chem. Commun., 2013, 49, 5468 RSC;
(e) C. Penga, W. Liangb, J. Jia, C. Fana, K. Kanagaraja, W. Wua, G. Chenga, D. Sua, Z. Zhonga and C. Yang, Chin. Chem. Lett., 2021, 32, 345 CrossRef.
-
(a) J. Ding, J. Chen, W. Mao, J. Huang and D. Ma, Org. Biomol. Chem., 2017, 15, 7894 RSC;
(b) M. Guo, X. Wang, C. Zhan, P. Demay-Drouhard, W. Li, K. Du, M. A. Olson, H. Zuilhof and A. C.-H. Sue, J. Am. Chem. Soc., 2018, 140, 74 CrossRef CAS PubMed;
(c) P. Demay-Drouhard, K. Du, K. Samanta, X. Wan, W. Yang, R. Srinivasan, A. C.-H. Sue and H. Zuilhof, Org. Lett., 2019, 21, 3976 CrossRef CAS PubMed;
(d) K. Du and A. C.-H. Sue, Synlett, 2019, 30, 2209 CrossRef CAS.
- Q. Kong, W. Zhuang, G. Li, Y. Xu, Q. Jiang and Y. Wang, New J. Chem., 2017, 41, 13784 RSC.
-
(a) T. Yamato, A. Miyazawa and M. Tashiro, J. Chem. Soc., Perkin Trans. 1, 1993, 3127 RSC;
(b) K. S. Unikela, B. L. Merner, P. G. Ghasemabadi, C. C. Warford, C. S. Qiu, L. N. Dawe, Y. Zhao and G. J. Bodwell, Eur. J. Org. Chem., 2019, 4546 CrossRef CAS.
- P. Skrinjar, M. Schwarz, S. Lexmüller, T. P. Mechtler, M. Zeyda, S. Greber-Platzer, J. Trometer, D. C. Kasper and H. Mikula, ACS Cent. Sci., 2018, 4, 1688 CrossRef CAS PubMed.
- X. Shu, S. Chen, J. Li, Z. Chen, L. Weng, X. Jia and C. Li, Chem. Commun., 2012, 48, 2967 RSC.
Footnotes |
† Electronic supplementary information (ESI) available. Experimental procedures, NMR and MS spectral data, HPLC charts and results of optical measurement. See DOI: https://doi.org/10.1039/d2sc04168e |
‡ The dissymmetry factors (g) of electronic transitions were described as follows, where μ and m are electric and magnetic transition dipole moments, respectively, and θ is the angle between them:
|
§ To gain further structural information, theoretical calculations of the hybrids were performed at the RωB97X-D/6-31G(d,p) and RB3LYP-D3/6-31G(d,p) levels, starting from C5-symmetric geometry without specific inter-unit interactions. However, TD-SCF calculations of the optimized structures failed to give consistent CD spectra for both levels. |
¶ To improve luminescence efficiency, optical measurement was also performed in the presence of 1 M adiponitrile.28 However, UV/vis absorption, CD, fluorescence and luminescence quantum yield measurement did not indicate any differences for 10b, 10c and 12. |
|
This journal is © The Royal Society of Chemistry 2022 |
Click here to see how this site uses Cookies. View our privacy policy here.