Porphyrin framework-derived N-doped porous carbon-confined Ru for NH3BH3 methanolysis: the more pyridinic-N, the better†
Received
10th August 2021
, Accepted 1st December 2021
First published on 1st December 2021
Abstract
Encapsulating metal nanoparticles (MNPs) inside specific porous materials is considered as a feasible strategy to prepare ultrafine MNPs with high stability. Herein, ultrafine Ru nanoparticles (NPs) with a mean size of 3.2 nm encapsulated in an N-doped porous carbon (NC) derived from the pyrolysis of a new porphyrin framework (POF) were fabricated via a simple wet chemical method, in which the POF was synthesized using 4,4′-biphenyldicarboxaldehyde and pyrrole as raw materials. The tomographic characterization of reconstructed slices confirmed the successful encapsulation of Ru NPs in POF-NC600 (pyrolysis temperature T = 600 °C). Due to the confinement effects and anchoring effects of POF-NC600, Ru@POF-NC600 shows the smallest particle size of Ru NPs and the highest hydrogen production performance toward the methanolysis of ammonia borane (AB) among the investigated catalysts, providing a record initial turnover frequency (TOF) value of 727 min−1 at 25 °C as compared to those heterogeneous catalysts ever reported. Systematic investigations reveal that pyridinic-N plays a pivotal role in the particle size distribution of Ru NPs and catalytic activity of the catalyst. Extended studies confirm that pyridinic-N, as an efficient anchoring site, determines the dispersity and size distribution of Ru NPs, thus affecting the catalytic activity of the catalyst. The more pyridinic-N, the better the catalytic activity. A series of isotopic experiments were performed, and it is found for the first time that the cleavage of the O–H bond in methanol is involved in the rate-determining step (RDS) of AB methanolysis. This work offers insights into rationally designing stable and ultrafine metal NPs confined in pyridinic-N-rich composites for various applications.
1. Introduction
Hydrogen, with high energy density and near-zero emission, has been extensively considered as an ideal clean energy carrier to solve the increasingly serious environment contamination and energy crises.1–3 Secure and efficient hydrogen storage is crucial to make hydrogen a viable energy carrier. Chemical hydrogen storage provides an optimum option for the particular applications of hydrogen in on-board vehicles and portable power sources. Among all the reported chemical hydrogen storage materials, ammonia borane (AB) has become a prominent candidate, due to its nontoxicity, long-term stability in solvents, and high gravimetric hydrogen capacity (19.6 wt%).4,5 AB can generate H2 through thermal decomposition; however, high dehydrogenation temperature and unwanted byproducts severely limited its practical applications.6,7 Another way to release H2 from AB is solvolysis in protic solvents, such as in water (hydrolysis, eqn (1)) and in methanol (methanolysis, eqn (2)).8–11 Compared with AB hydrolysis, AB methanolysis has some advantages, such as higher dissolvability in methanol, higher hydrogen purity, efficient regeneration of byproducts, and possible application at low temperatures (≤0 °C).12–14 | NH3BH3 + 2H2O → NH4BO2 + 3H2 | (1) |
| NH3BH3 + 4CH3OH → NH4B(CH3O)4 + 3H2 | (2) |
To date, tremendous attempts have been made to fabricate highly active and highly stable metal nanoparticle (MNP) catalysts for AB methanolysis.10,11,15–17 Encapsulating MNPs inside specific porous materials has been considered as a feasible strategy to prepare ultrafine and highly dispersed MNPs with high stability and activity. The confinement effect of the supports can effectively control the growth of MNPs, diminish nanoparticle aggregation, and enhance nanoparticle stability. To this end, numerous porous materials, including porous organic polymers (POPs),18 metal–organic frameworks (MOFs),19–21 covalent organic frameworks (COFs),22,23 organic molecular cages,24–26 and porous carbon,27–31 have been successfully constructed to encapsulate MNPs. Among all these porous materials, porous carbon, as an outstanding candidate, has attracted much attention in encapsulating MNPs because of their nontoxicity, inherent porous structure, and good physical and chemical durability.
Furthermore, when doping nitrogen atoms into carbon skeletons, nitrogen groups will modify the electronic properties of carbon materials and then change their physicochemical properties.32–36 Especially, for supported MNPs, the doping of nitrogen can not only enhance the electronic density of supported MNPs by coordinating with the empty orbit of the metal, but also provide more anchoring sites to obtain MNPs with a high dispersity and small size.37–42 A variety of nitrogen configurations are formed during the N doping process, such as pyridinic nitrogen (pyridinic-N), pyrrolic nitrogen (pyrrolic-N), and graphitic nitrogen (graphitic-N). Pyridinic-N and pyrrolic-N are the nitrogen species at the edge or in the vacancies of the graphitic matrix, which is more easily exposed and more likely coordinates with the empty orbit of the metal.43–45 The porphyrin framework (POF), one of the organic framework materials composed of porphyrin units connected by covalent bonds,46 is expected to be a good precursor to fabricate N-doped porous carbon due to its diversity in composition, inherent porous properties, high content of nitrogen, and ease of functionalization.47–49
Herein, a new POF was synthesized with 4,4′-biphenyldicarboxaldehyde (BPDA) and pyrrole as raw materials. Subsequently, the N-doped porous carbon derived from the pyrolysis of the POF was used as the support to encapsulate active Ru NPs toward the methanolysis of AB for hydrogen production. During the pyrolysis process, pyrrole rings in the POF open and rearrange into more stable pyridine rings, resulting in the generation of pyridine nitrogen (pyridinic-N). The effects of nitrogen species on the particle size of MNPs as well as catalytic activity are investigated in detail. The optimized catalyst Ru@POF-NC600 displays ultrahigh catalytic performance toward AB methanolysis at 25 °C.
2. Experimental
2.1 Fabrication of porphyrin framework-derived N-doped porous carbons
BPDA-POF was fabricated by condensation polymerization in acetic acid with BPDA and pyrrole as raw materials (see the ESI†). About 0.6 g of BPDA-POF was placed in a ceramic boat and then transferred into a pyrolyzing furnace. Subsequently, pyrolyzation was performed at a designed temperature (T = 500, 600, 700, and 800 °C) for 3 h under an argon atmosphere. Finally, porphyrin framework-derived N-doped porous carbons (POF-NCT) were obtained and denoted as POF-NC500, POF-NC600, POF-NC700, and POF-NC800.
2.2 Synthesis of Ru@POF-NC600 and hydrogen generation from AB
Ru@POF-NC600 was prepared by a facile ultrasonic impregnation reduction method. Specifically, 251 mg of POF-NC600 and 4 mL methanol were added into a three-necked flask and then ultrasonically dispersed evenly at 25 °C. After adding 1 mL of 0.0125 M RuCl3 methanol solution, the system was further ultrasonically impregnated for 2 h to ensure the complete diffusion of the Ru precursor inside the pores of POF-NC600. After the displacement with argon three times, 1 mmol AB (31.8 mg) was added and the Ru precursor was immediately reduced to produce 0.50 wt% Ru@POF-NC600. The released hydrogen can be monitored by reading the volume of displaced water in a gas burette at 25 °C. Using the above method, Ru@POF-NC600 catalysts with other loadings of Ru were prepared by changing the mass of POF-NC600.
2.3 Durability test of catalysts
The durability of Ru@POF-NC600 was tested under an argon atmosphere at 25 °C. After the first run of the hydrogen generation reaction, a new batch of 1 mmol AB was added to the reaction system, and then the hydrogen production reaction occurred. The test was repeated five times to examine the durability of the catalyst.
3. Results and discussion
3.1 Fabrication and characterization
For the first time, a new POF was synthesized using modified solution-processed condensation polymerization based on a previous report (Fig. 1a).45 Fourier transform infrared spectroscopy (FTIR) characterization was conducted to analyse the changes in functional groups during the synthesis of BPDA-POF (Fig. 1b). The disappearance of the aldehyde group stretching vibration (1694 cm−1) and aldehyde hydrogen stretching vibration (2742 and 2838 cm−1) of BPDA after the polymerization indicates the complete conversion of BPDA to a POF. The adsorption bands at 1604 and 1677 cm−1 belong to the stretching vibrations of C
C and C
N in the POF.50 The adsorption bands of the C–H backbone at about 806 and 1000 cm−1 can be assigned to the C–H vibrations of in-plane porphyrin deformation modes.51 The solid-state 13C nuclear magnetic resonance (NMR) spectrum of the POF is shown in Fig. 1c, in which the peaks at 128.2 and 135.6 ppm can be assigned to the porphyrin and benzene linkages, further confirming the successful construction of the porphyrin framework. Organic elemental analysis (OEA) of BPDA-POF was comprehensively performed by the combustion method (COM) (Fig. 1d). BPDA-POF displays a C content of 87.1 wt% and N content of 8.8 wt%, which is close to the theoretical value of 86.4 wt% and 9.0 wt%, respectively. According to the scanning electron microscopy (SEM) image of BPDA-POF in Fig. 1e, a typical spherical morphology was observed, distinguished from the two-dimensional sheet structures of porphyrin-based framework materials ever reported.52–55
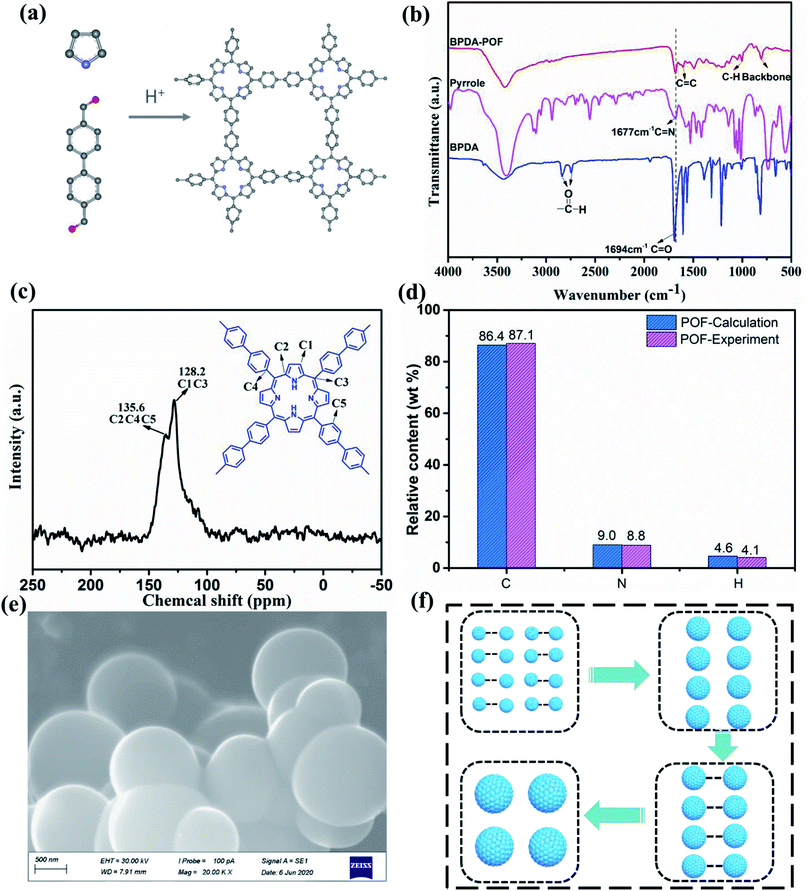 |
| Fig. 1 (a) Schematic illustration of the synthesis of BPDA-POF; the carbon, nitrogen, and oxygen atoms are marked in gray, blue, and red, respectively; (b) FTIR spectra of BPDA, pyrrole, and BPDA-POF; (c) solid-state 13C NMR spectrum of BPDA-POF; the inset of (c) shows the corresponding identified structure; (d) organic elemental analysis (OEA) of BPDA-POF by the experimental combustion method and theoretical calculation; (e) typical SEM image of BPDA-POF; (f) proposed formation mechanism for spherical BPDA-POF. | |
To explore the formation mechanism of the spherical POF, the morphology of BPDA-POF was examined by transmission electron microscopy (TEM) during the synthetic reaction, which was quenched after 1 min, 2 min, 5 min, 30 min, and 2 h at room temperature and 12 h at 110 °C. Initially, uniform nanospheres less than 4 nm were obtained by the polymerization of pyrrole and BPDA (Fig. S1a†). Subsequently, these nanospheres gradually grew to about 600 nm by combining with each other after 30 min of the reaction (Fig. S1b–d†). After 2 h of the polymerization reaction at room temperature, a 1 μm spherical POF was observed as shown in Fig. S1e.† Finally, the reaction temperature was raised to 110 °C and the morphology of the POF did not change after solvothermal polymerization at high temperature (Fig. S1f†). Therefore, a sphere-coalescence formation mechanism was proposed for the spherical POF (BPDA-POF).
SEM and TEM tests were performed to analyse the morphological changes of as-synthesized BPDA-POF, POF-NC600, and Ru@POF-NC600. As shown in Fig. 2b and S2,† all SEM and TEM images of BPDA-POF, POF-NC600, and Ru@POF-NC600 exhibit a uniform spherical morphology with a diameter of 1.0 μm. No destruction of the spherical structure is observed in Ru@POF-NC600 after the pyrolysis of the POF and introduction of MNPs. Ru NPs in Ru@POF-NC600 are highly dispersed in POF-NC600 and the mean size is determined to be 3.2 nm as shown in Fig. 2c. In contrast, the POF-NC600-free Ru NPs display a conspicuous aggregation with a much bigger particle size of 8.7 nm (Fig. S3†). According to high resolution TEM (HRTEM) (Fig. 2d), the lattice spacing of Ru NPs in Ru@POF-NC600 is measured to be 0.206 nm, which is indexed to the (101) plane of Ru and also confirmed by the selected area electron diffraction (SAED) pattern (Fig. 2e). In order to determine the location of Ru NPs in the spherical Ru@POF-NC600 catalyst, a series of reconstructed slices were investigated by high-angle annular dark-field scanning transmission electron microscopy (HAADF-STEM). Tomographic slice images show that Ru NPs are dispersed throughout the whole spherical skeleton, confirming that almost all Ru NPs are indeed encapsulated inside the inner pores of spherical POF-NC600 (Fig. 2f and g). The confinement effect can effectively prevent the aggregation of Ru NPs, which is beneficial for an excellent stability and activity during the dehydrogenation reaction of AB. The energy dispersive X-ray (EDX) spectra of Ru@POF-NC600 are demonstrated in Fig. S4,† and C, N, and Ru were elucidated to be present in Ru@POF-NC600 with a content of 92.83 wt%, 6.70 wt%, and 0.43 wt%, respectively, which is fairly close to the designed value. To dig into the effect of pyrolysis temperatures, the particle size distributions of Ru NPs in POF-NCT pyrolyzed at different temperatures were further studied. As shown in Fig. S5,† Ru@POF-NC500, Ru@POF-NC600, Ru@POF-NC700, and Ru@POF-NC800 show an average size of Ru NPs of about 5.7, 3.2, 3.9, and 5.1 nm, respectively. The size distributions of Ru NPs are in the order of Ru@POF-NC600 < Ru@POF-NC700 < Ru@POF-NC800 < Ru@POF-NC500. Moreover, the metal dispersion (DM) is calculated on the basis of the average particle diameter of Ru NPs (see the ESI†). Ru@POF-NC500, Ru@POF-NC600, Ru@POF-NC700, and Ru@POF-NC800 exhibit a DM of 27.3%, 42.2%, 34.6%, and 26.5%, respectively (Table S1†). Undoubtedly, the smaller the particle size of Ru NPs, the higher the metal dispersion and surface atomic utilization.
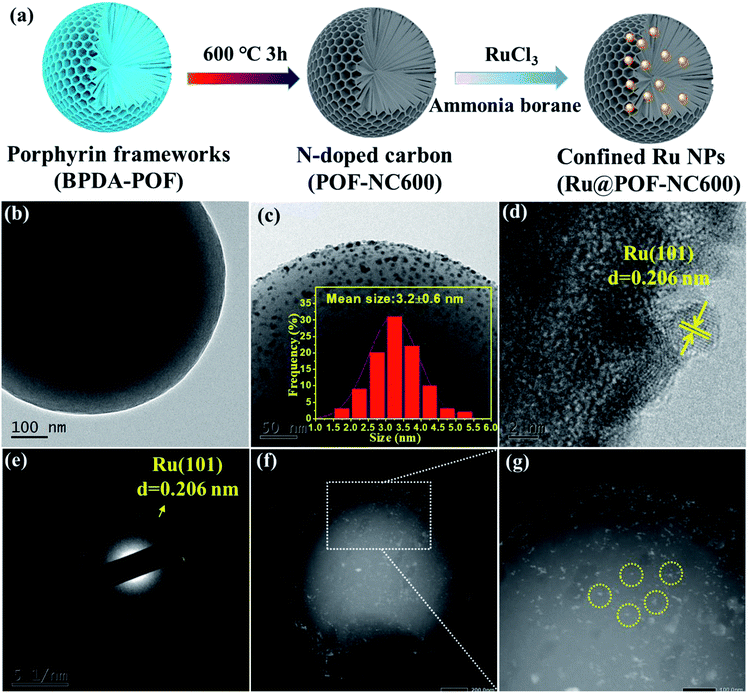 |
| Fig. 2 (a) Schematic illustration of the synthesis of the N-doped carbon (POF-NC600) and confined Ru NP catalyst (Ru@POF-NC600); (b) and (c) TEM images of POF-NC600 and Ru@POF-NC600; the inset in (c) shows the corresponding size distribution of Ru NPs; (d) high-resolution TEM image of Ru@POF-NC600; (e) the electron diffraction pattern of Ru@POF-NC600; (f) HAADF-STEM image of a reconstructed slice for spherical Ru@POF-NC600; (g) enlarged area of the dotted line box of (f). | |
Powder X-ray diffraction (PXRD) patterns were recorded to analyse the crystal structure of the POF, POF-NCT, and the catalysts. As shown in Fig. S6a,† BPDA-POF materials prepared at different reaction times exhibit a distinct diffraction peak at about 18°, suggesting an inherent ordered porous structure.45 After pyrolysis, the distinct peaks of the POF (18°) disappear, and two broad peaks at around 25° and 43° (Fig. S6b†) can be observed, belonging to the (002) and (101) planes of the graphitic phase.56 Additionally, with the increase of pyrolytic temperature, the intensity of diffraction patterns at 25° and 43° becomes stronger, suggesting the increase in the graphitization degree from POF-NC500 to POF-NC800. The XRD patterns of POF-NC600, Ru@POF-NC600, and support-free Ru are shown in Fig. 3a. The diffraction peak of metallic Ru (101) (at 44°) in Ru@POF-NC600 is inconspicuous as compared to that of support-free Ru NPs, probably owing to the ultrafine size of Ru NPs confined in POF-NC600. As depicted in the Raman spectra of POF-NCT prepared at different temperatures (Fig. 3b), two typical carbon peaks are found at 1333 cm−1 (D band) and 1591 cm−1 (G band), belonging to the lattice defects or edges, and graphitic structure carbon, respectively.57–59 At the initial stage of the pyrolysis (500 °C), weak D and G peaks are observed with a minimum value of ID/IG (1.00), owing to the small amount of carbonization. POF-NC600 shows the maximum value of ID/IG (1.15), suggesting the most defect sites on the framework of POF-NC600, which may provide more stable anchoring sites for MNPs.36 Additionally, the defect sites reduce with the further increase of the carbonization temperature, due to the increase of the graphitization degree.
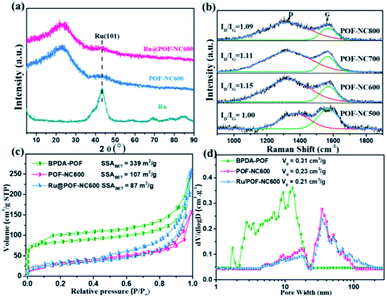 |
| Fig. 3 (a) Powder XRD patterns of POF-NC600, Ru@POF-NC600, and support-free Ru; (b) Raman spectra of POF-NCT with different pyrolysis temperatures; (c) and (d) N2 adsorption/desorption isotherms and the corresponding pore size distribution of BPDA-POF, POF-NC600 and Ru@POF-NC600. | |
To analyse the Brunauer–Emmett–Teller (BET) specific surface area (SSABET) and the pore size distribution of the synthesized materials, their N2-sorption isotherms have been recorded (Fig. 3c). The SSABET and pore volume of BPDA-POF are 339 m2 g−1 and 0.31 cm3 g−1, respectively. According to the pore size distribution curve (Fig. 3d), a typical hierarchically microporous (1.7 nm) and mesoporous structure (2–20 nm) can be observed in BPDA-POF. After the introduction of Ru NPs, the SSABET and pore volume of Ru@POF-NC600 reduce from 107 m2 g−1 and 0.23 cm3 g−1 of POF-NC600 to 87 m2 g−1 and 0.21 cm3 g−1, indicating the successful encapsulation of Ru NPs inside the pores of POF-NC600.
The surface interactions and chemical states of POF-NCT, support-free Ru, and Ru@POF-NC600 were analyzed by X-ray photoelectron spectroscopy (XPS). As depicted in the XPS survey spectrum of Ru@POF-NC600 (Fig. S7†), the existence of C, N, and Ru is clearly revealed. The C 1s spectra of Ru@POF-NC600 and POF-NC600 can be well fitted to C–C/C
C/Ru 3d3/2, C–N, and C
N, respectively (Fig. 4a). No shifts of the binding energy can be observed in the C–C/C
C/Ru 3d3/2 and C–N bonds of Ru@POF-NC600 and POF-NC600. Meanwhile, the binding energy of C
N shifts from 287.1 to 287.3 eV, indicating an electron-donating effect of the C
N bond, which can be attributed to the electron resonance transfer of pyridinic-N. It is notable that the overlap of the Ru 3d3/2 and C 1s peaks at around 284.6 eV makes it difficult to identify the peak of Ru. So, the 3p orbital of Ru is selected to investigate surface interactions and electron transfer. In the Ru 3p XPS spectra of Ru@POF-NC600 (Fig. 4b), two prominent bands observed at 461.8 and 484.0 eV can be ascribed to Ru0 3p3/2 and Ru0 3p1/2, respectively, suggesting the Ru(0) state. The other two slightly higher energy peaks show the existence of the Ru(III) state (Fig. 4b), which may arise from the surface oxidation of Ru NPs during XPS sampling. A negative shift of the binding energy can be obtained in Ru@POF-NC600 from 462.1 (Ru 3p3/2) and 484.3 eV (Ru 3p1/2) to 461.8 and 484.0 eV compared with support-free Ru NPs, suggesting that electron transfer occurred from the support to Ru. The electron-rich Ru NPs can efficiently weaken the B–N bond, which is beneficial for the further breaking of B–N and B–H bonds.23,60 In addition, a strong metal-support interaction between Ru NPs and POF-NC600 can be revealed, which is helpful to immobilize MNPs, increase the dispersity, and reduce the size of MNPs. The high-resolution N 1s XPS spectra of POF-NCT prepared at different pyrolysis temperatures are shown in Fig. 4c and S8.† Pyrrolic-N and pyridinic-N are confirmed to be the primary N components in the N 1s spectra of all POF-NCT materials, belonging to the pyrrole ring derived from porphyrin and pyridine rings produced by pyrolysis.
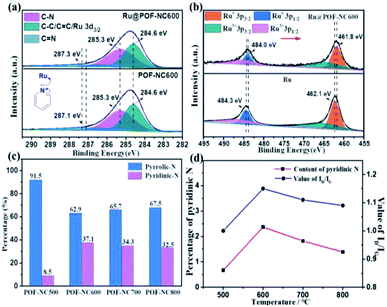 |
| Fig. 4 (a) C 1s XPS spectra of Ru@POF-NC600 and POF-NC600; the inset of (a) shows the corresponding electron resonance structure; (b) Ru 3p XPS spectra of unsupported Ru and Ru@POF-NC600; (c) the corresponding percentages of pyrrolic-N and pyridinic-N in POF-NCT prepared at different pyrolysis temperatures; (d) correlation between the content of pyridinic-N and ID/IG value. | |
The contents of C and N atoms in POF-NCT prepared at different pyrolysis temperatures were measured by OEA characterization. As illustrated in Table S2,† the content of C increases gradually with the increase of pyrogenation temperature; however, the content of N decreases, indicating an increase in the degree of graphitization, which is consistent with Raman and XRD characterization (Fig. 3b and S6b†). Furthermore, the content of pyridinic-N in POF-NCT shows an increasing trend first and then decreasing with the increase of pyrolysis temperature and the changing trend of the pyridinic-N content is consistent with that of the ID/IG value (Fig. 4d). To explain this phenomenon, the transformations of nitrogen species during the pyrolysis process are analyzed. At the initial stage of pyrolysis (500–600 °C), pyrrole rings in the porphyrin frameworks open and rearrange into more stable pyridine rings, leading to the increase of the pyridinic-N content (Fig. 4d and Table S2†). Meanwhile, with the destruction of the original porphyrin framework's structure, more defect sites and an increased value of ID/IG can be observed. At high pyrolysis temperatures (600–800 °C), the gradual dissociation of nitrogen species from the carbon skeleton results in the increase of the graphitization degree and the decrease of the ID/IG value. As a result, pyridinic-N directly reflects the number of defect sites. POF-NC600 with more pyridinic-N and/or more defect sites will provide more anchor sites for MNPs than POF-NC500, POF-NC700, and POF-NC800.
3.2 Catalytic performance measurement for AB methanolysis
The catalytic activities of different catalysts including Ru@POF-NC600, Ru@POF, Ru@PVP, and support-free Ru NPs are investigated for H2 generation from AB methanolysis. As shown in Fig. 5a and b, the POF-NC600 support is inactive toward the methanolysis of AB, confirming that pyridinic-N itself has no effect on the methanolysis of AB. Ru NPs supported on pyrrolic-N-rich BPDA-POF (Ru@POF) displays a low catalytic performance with an initial TOF value of 374 min−1, suggesting the weak promoting effect of pyrrolic-N on the catalytic performance. Support-free Ru NPs and Ru NPs stabilized by PVP (Ru@PVP) exhibit a high TOF value of 514 and 538 min−1; however, the entire dehydrogenation time increases significantly, due to the aggregation of Ru NPs during the reaction process. Remarkably, Ru@POF-NC600 displays the highest catalytic activity with an initial TOF value of 727 min−1 (total TOF = 423 min−1), which is 2 times higher than that of Ru@POF and 1.5 times higher than that of support-free Ru NPs and Ru@PVP, surpassing all heterogeneous catalysts ever reported for the methanolysis of AB (Table S3†). Gas chromatography (GC) was used to detect the gas produced during the methanolysis of AB catalyzed by Ru@POF-NC600. No other gas was found except H2 (Fig. S9†). The catalytic performances of Ru@POF-NC600 with different loadings of Ru are shown in Fig. S10.† The optimal loading of Ru is observed at 0.50 wt%. Fig. 5c shows the catalytic performances of Ru NPs immobilized by POF-NCT obtained at different pyrolysis temperatures. Ru@POF-NC500, Ru@POF-NC600, Ru@POF-NC700, and Ru@POF-NC800 provide an initial TOF value of 391, 727, 585, and 442 min−1 toward AB methanolysis, respectively (Fig. 5d). In order to identify the role of surface active sites in the catalytic activity, the corrected TOF values (TOFcorrected) based on the surface atoms of Ru NPs are calculated by using the equation TOFcorrected = TOF/DM. The TOFcorrected values of Ru@POF-NC500, Ru@POF-NC600, Ru@POF-NC700, and Ru@POF-NC800 are 1650, 1723, 1682, and 1668 min−1, respectively (Table S4†). As expected, Ru@POF-NC600 with the smallest particle size of Ru NPs and maximum DM of 42.2% demonstrates the highest TOFcorrected for AB methanolysis. The catalytic activities are in the order of Ru@POF-NC600 > Ru@POF-NC700 > Ru@POF-NC800 > Ru@POF-NC500 for AB methanolysis. Significantly, the variation tendency of the pyridinic-N content is opposite to that of the particle size of Ru NPs in these Ru@POF-NCT samples (Fig. S5† and 5d), but consistent with that of the catalytic performances of Ru@POF-NCT samples, further confirming that the supported Ru NPs are indeed active sites. These results suggest that pyridinic-N plays a pivotal role in the particle size distribution of Ru NPs and catalytic activity of Ru@POF-NCT. To explain these findings, the electronic structures of the pyridine ring and pyrrole ring are analysed in detail. In the pyridine ring, sp2 hybridized N bonds with two adjacent C and two p-electrons perpendicular to the hexatomic ring are not conjugate with the π-system. However, in the pyrrole ring, sp3 hybridized N incorporated in the pentagonal ring contributes two p-electrons to the π-system.61,62 Therefore, pyridinic-N with unconjugated lone pair electrons is more likely to coordinate with the empty orbit of Ru atoms and undergo electron transfer. Accordingly, the strong metal–support interaction between Ru NPs and POF-NC600 is beneficial to immobilize MNPs, increase the dispersity, and reduce the size of MNPs. As a result, POF-NC600 with more pyridinic-N will provides more anchoring sites for Ru NPs, resulting in a higher dispersity and smaller size of MNPs than those of POF-NCT prepared at other pyrolysis temperatures. Ru@POF-NC600 with the highest content of pyridinic-N and the smallest particle size of Ru NPs among the investigated catalysts demonstrates the highest catalytic performance for the methanolysis of AB. The more pyridinic-N, the better the catalytic activity.
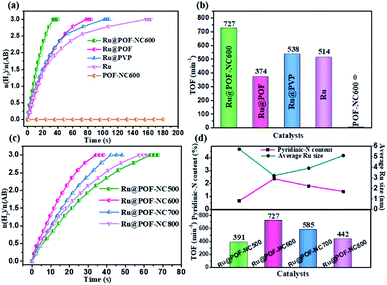 |
| Fig. 5 (a) Time course plots of H2 generation from AB methanolysis over different catalysts at 25 °C; (b) the corresponding initial TOF value of (a); (c) time course plots of H2 generation from AB methanolysis over Ru@POF-NCT with different pyrolysis temperatures; (d) the correlation between the content of pyridinic-N and the particle size of Ru NPs, as well as the corresponding initial TOF value of Ru@POF-NCT with different pyrolysis temperatures. | |
The influence factors of the catalytic activity toward AB methanolysis, such as the dosages of AB and catalyst as well as the reaction temperature were also investigated. Fig. 6a shows the time course plots of hydrogen generation over Ru@POF-NC600 with different AB concentrations at 25 °C. An obvious linear relationship between the logarithm of the hydrogen generation rate and AB initial concentration is obtained and the slope is calculated to be 0.07, suggesting a zero-order dependence on AB concentration, which implies that AB is easily activated under the present reaction conditions, excluding the possibility of the activation of AB in the rate-determining step (RDS).63 The relationship between the logarithm of the hydrogen evolution rate and metal initial concentration is also investigated, and a straight line with a slope of 1.3 can be observed (Fig. 6b), indicating the acceleration of the hydrogen generation rate with increasing catalyst amount.64,65 As shown in Fig. S11,† the rate of H2 release is also significantly accelerated with the increase of reaction temperature. According to the Arrhenius equation, the activation energies (Ea) for AB methanolysis are calculated to be 48.3 kJ mol−1 for Ru@POF-NC500, 39.4 kJ mol−1 for Ru@POF-NC600, 45.5 kJ mol−1 for Ru@POF-NC700, and 47.8 kJ mol−1 for Ru@POF-NC800 (Fig. 6c), and the tendency is consistent with that trend of the TOF value, confirming the strong dynamic dependence of Ea rather than diffusion control.66 Additionally, Ru@POF-NC600 retains relatively high catalytic performance (TOFinitial = 129 min−1) for AB methanolysis even at 0 °C (Fig. S11b†). The prominent low-temperature catalytic performance of the Ru@POF-NC600 catalyst is extremely crucial to practical applications in cold regions, highlighting the advantage of hydrogen production from AB methanolysis as compared to that from AB hydrolysis.
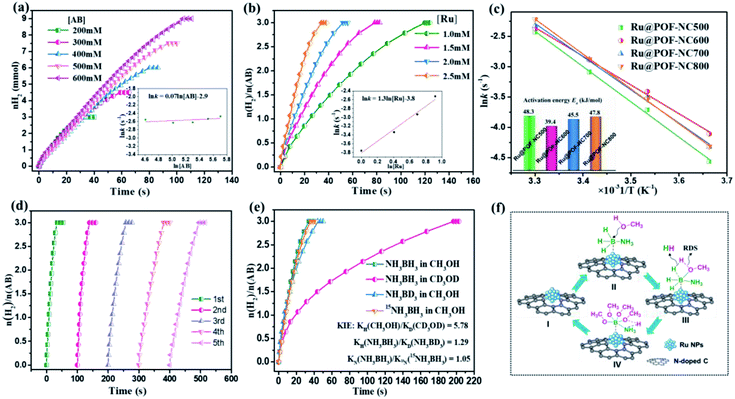 |
| Fig. 6 Time course plots of H2 generation for AB methanolysis over Ru@POF-NC600 at 25 °C: (a) with different AB concentrations; inset of (a) shows the ln[AB] vs. ln k plot; (b) with different catalyst concentrations; inset of (b) shows the ln[Rh] vs. ln k plot; (c) Arrhenius plots and the corresponding activation energies of Ru@POF-NCT; (d) durability tests for AB methanolysis over Ru@POF-NC600 at 25 °C; (e) isotopic experiments of catalytic methanolysis of AB over Ru@POF-NC600; (f) a plausible mechanism of AB methanolysis catalyzed by Ru@POF-NC600. | |
To avoid the oxidation of Ru NPs during the recycle test, durability experiments were carried out under the protection of argon. As shown in Fig. 6d, a complete production of H2 from AB catalyzed by Ru@POF-NC600 can be observed five times, but the hydrogen generation rate slightly decreases. A series of characterization experiments were carried out to analyse the change of the catalysts before and after the cycles. XRD diffraction patterns (Fig. S12†) and SEM images (Fig. S13a†) demonstrate an inconspicuous variation of the morphology of Ru@POF-NC600 after recycle experiments. A slight aggregation of Ru NPs with an average particle size of 3.6 nm can be found in the TEM image of the recycled Ru@POF-NC600 (Fig. S13b†), confirming the good cycling stability of Ru NPs confined in the pores of POF-NC600. In addition, the Ru 3p and N 1s XPS spectra of Ru@POF-NC600 before and after recycle experiments are shown in Fig. S14.† No obvious migrations of binding energy are observed in Ru 3p and N 1s orbitals as compared with that of the fresh catalyst, confirming the durability of the valence states and coordination structures of Ru NPs and nitrogen species.
| NH3BD3 + 4CH3OH → NH4B(CH3O)4 + 3HD | (3) |
| 15NH3BH3 + 4CH3OH → 15NH4B(CH3O)4 + 3H2 | (4) |
| NH3BH3 + 4CD3OD → NH3DB(CD3O)4 + 3HD | (5) |
For the methanolysis reaction of AB, the cleavages of the B–H and B–N bonds in AB and O–H bonds in methanol have been reported to be involved in the hydrogen evolution reaction;61,63 however, the effect of these bonds in the RDS has not been clear. To confirm the role of the B–H, B–N, and O–H bonds in the AB methanolysis reaction, for the first time, a series of isotopic experiments were performed by replacing NH3BH3 with NH3BD3 (eqn (3)) and 15NH3BH3 (eqn (4)), and CH3OH with CD3OD (eqn (5)). As shown in Fig. 6e, the methanolysis of both NH3BD3 and 15NH3BH3 in CH3OH shows a slightly lower hydrogen release rate compared to NH3BH3 under the same reaction conditions. The kinetic isotope effect (KIE) constant for NH3BD3 and 15NH3BH3 is calculated to be 1.29 and 1.05, suggesting a secondary kinetic isotope effect (SKIE) and revealing that the cleavage of B–H and B–N bonds in AB is a rapid reaction step of the methanolysis reaction, consistent with the zero-order dependence on AB concentration (Fig. 6a). However, the methanolysis of NH3BH3 in CD3OD demonstrates a significantly lower reaction rate compared to NH3BH3 in CH3OH with a primary kinetic isotope effect (PKIE) of 5.78, indicating that methanol plays an important role in the process of AB methanolysis. Therefore, it is firstly proved that the cleavage of the O–H bond in CH3OH is indeed involved in the RDS for the methanolysis of AB. In addition, the bond energy of O–H, B–H, and B–N bonds is 493, 430, and 117 kJ mol−1.67,68 Therefore, the involvement of the cleavage of the O–H bond in the RDS may be partially explained by the higher bond energy of the O–H bond than that of B–N and B–H bonds. 11B NMR spectroscopy was used to monitor the changes of boron species before and after the methanolysis reaction of AB in CD3OD. The 11B NMR spectrum of the reaction system before the methanolysis of AB shows a quartet at −24.20 to −22.75 ppm (Fig. S15†), belonging to the typical peak of borohydride (NH3BH3). After the methanolysis of AB, the peak of AB disappears and a broad singlet at 6.62 ppm can be observed (Fig. S16†), which can be assigned to alkoxy borate (methanolysis product), confirming the complete transformation of NH3BH3 into NH3DB(CD3O)4. Therefore, a plausible mechanism for AB methanolysis catalyzed by Ru@POF-NC600 was proposed as shown in Fig. 6f. In the first step, the AB molecule is absorbed on Ru NPs stabilized by pyridinic-N in the monovacancy of an N-doped carbon (II). Subsequently, an activated complex (III) can be formed via the attack of CH3OH on the B atom, in which the cleavage of the O–H bond in CH3OH has been proved to be involved in the RDS and a molecule of H2 is released by the cleavages of O–H and B–H bonds. The further attacks of three molecules of CH3OH lead to the formation of an alkoxy borate (NH4B(CH3O)4) (IV). Finally, the catalyst Ru@POF-NC600 (I) can be regenerated accompanied by the dissociation of NH4B(CH3O)4.
4. Conclusions
In conclusion, pyridinic-N-rich N-doped porous carbons (POF-NCT) were synthesized using a porphyrin framework (POF) as the self-sacrificing template. POF-NCT-confined Ru NP catalysts were then prepared by encapsulating ultrafine Ru NPs in the pores of N-doped porous carbons. The tomographic characterization of reconstructed slices confirmed that the highly dispersed Ru NPs with a mean size of 3.2 nm were successfully encapsulated in the pores of POF-NC600. The nanoscale confining effect of the pores can effectively prevent the aggregation and loss of Ru NPs. The resultant pyridinic-N-rich Ru@POF-NC600 composite displayed the highest activity toward methanolysis of AB for hydrogen production. The effect of nitrogen atoms on the catalytic activity was explored and the results reveal that the anchoring effects of pyridinic-N in POF-NCT can lead to a high dispersity and small size of Ru NPs. Ru@POF-NC600 with the highest content of pyridinic-N demonstrates the smallest size of Ru NPs among the investigated catalysts Ru@POF-NCT, resulting in the lowest activation energy and the highest catalytic activity toward AB methanolysis. In light of the promoting effect of pyridinic-N on the small size of MNPs, we anticipate that this discovery will help us search for more stable and active catalysts for various applications.
Conflicts of interest
There are no conflicts to declare.
Acknowledgements
This work was financially supported by the National Natural Science Foundation of China (No. 22162014, 22162013, and 21763012), Natural Science Foundation of Jiangxi Province of China (No. 20212ACB204009), and Sponsored Program for Academic and Technical Leaders of Major Disciplines of Jiangxi Province of China (No. 20212BCJL23059).
References
- N. Armaroli and V. Balzani, The hydrogen issue, Chemsuschem, 2011, 4, 21–36 CrossRef CAS PubMed.
- J. Yang, A. Sudik, C. Wolverton and D. J. Siegel, High capacity hydrogen storage materials: attributes for automotive applications and techniques for materials discovery, Chem. Soc. Rev., 2010, 39, 656–675 RSC.
- L. Schapbach and A. Zuttel, Hydrogen-storage materials for mobile applications, Nature, 2001, 414, 353–358 CrossRef PubMed.
- H.-L. Jiang, S. K. Singh, J.-M. Yan, X.-B. Zhang and Q. Xu, Liquid-phase chemical hydrogen storage: catalytic hydrogen generation under ambient conditions, ChemSusChem, 2010, 3, 541–549 CrossRef CAS PubMed.
- Z.-H. Lu, H.-L. Jiang, M. Yadav, K. Aranishi and Q. Xu, Synergistic catalysis of Au-Co@SiO2 nanospheres in hydrolytic dehydrogenation of ammonia borane for chemical hydrogen storage, J. Mater. Chem., 2012, 22, 5065 RSC.
- V. Sit, R. A. Geanangel and W. W. Wendlandt, The thermal dissociation of NH3BH3, Thermochim. Acta, 1987, 113, 379–382 CrossRef CAS.
- A. Gutowska, L. Li, Y. Shin, C.-M. Wang, X.-S. Li, J. C. Linehan, R. S. Smith, B. D. Kay, B. Schmid, W. Shaw, M. Gutowski and T. Autrey, Nanoscaffold Mediates Hydrogen Release and the Reactivity of Ammonia Borane, Angew. Chem., Int. Ed., 2005, 44, 3578–3582 CrossRef CAS.
- C.-Y. Peng, L. Kang, S. Cao, Y. Chen and W.-F. Fu, Nanostructured Ni2P as a Robust Catalyst for the Hydrolytic Dehydrogenation of Ammonia–Borane, Angew. Chem., Int. Ed., 2016, 127, 15951–15955 CrossRef.
- H.-E. Wang, C. Hua, C. Liu, C. Xue, Q. Jiang, Z. Lu, Y.-L. Yang, C.-Y. Chung, W. Zhang and J. A. Zapien, Facile synthesis and electrochemical characterization of porous and dense TiO2 nanospheres for lithium-ion battery applications, J. Power Sources, 2011, 196, 6394–6399 CrossRef CAS.
- H.-L. Jiang, T. Akita, T. Ishida, M. Haruta and Q. Xu, Synergistic catalysis of Au@Ag core-shell nanoparticles stabilized on metal-organic framework, J. Am. Chem. Soc., 2011, 133, 1304–1306 CrossRef CAS PubMed.
- J.-K. Sun, Z. Kochovski, W.-Y. Zhang, H. Kirmse and J. Yuan, A General Synthetic Route Towards Highly Dispersed Metal Clusters Enabled by Poly(ionic liquid)s, J. Am. Chem. Soc., 2017, 139, 8971 CrossRef CAS PubMed.
- A. Brockman, Z. Yuan and J. Gore, A study of catalytic hydrolysis of concentrated ammonia borane solutions, Int. J. Hydrogen Energy, 2010, 35, 7350–7356 CrossRef CAS.
- Q. Yao, M. Huang, Z.-H. Lu, Y. Yang, Y. Zhang, X. Chen and Z. Yang, Methanolysis of ammonia borane by shape-controlled mesoporous copper nanostructures for hydrogen generation, Dalton Trans., 2015, 44, 1070–1076 RSC.
- J. Song, X. Gu, J. Cheng, N. Fan, H. Zhang and H. Su, Remarkably boosting catalytic H2 evolution from ammonia borane through the visible-light-driven synergistic electron effect of non-plasmonic noble-metal-free nanoparticles and photoactive metal-organic frameworks, Appl. Catal., B, 2018, 225, 424–432 CrossRef CAS.
- Q. Yao, W. Shi, G. Feng, Z.-H. Lu, X. Zhang, D. Tao, D. Kong and X. Chen, Ultrafine Ru nanoparticles embedded in SiO2 nanospheres: Highly efficient catalysts for hydrolytic dehydrogenation of ammonia borane, J. Power Sources, 2014, 257, 293–299 CrossRef CAS.
- Q. Yao, Z.-H. Lu, Y. Yang, Y. Chen, X. Chen and H.-L. Jiang, Facile synthesis of graphene-supported Ni-CeOx nano-composites as highly efficient catalysts for hydrolytic dehydrogenation
of ammonia borane, Nano Res., 2018, 11, 4412–4422 CrossRef CAS.
- J. Liu, L. Chen, H. Cui, J. Zhang, L. Zhang and C.-Y. Su, Applications of metal–organic frameworks in heterogeneous supramolecular catalysis, Chem. Soc. Rev., 2014, 43, 6011–6061 RSC.
- P. Kaur, J. T. Hupp and S. T. Nguyen, Porous Organic Polymers in Catalysis: Opportunities and Challenges, ACS Catal., 2011, 1, 819–835 CrossRef CAS.
- Z. Liang, R. Zhao, T. Qiu, R. Zou and Q. Xu, Metal-organic framework-derived materials for electrochemical energy applications, EnergyChem, 2019, 1, 100001 CrossRef.
- F.-C. Meng, S.-Y. Zhang, L. Ma, W.-N. Zhang, M. Li, T.-P. Wu, H. Li, T. Zhang, X.-H. Lu, F.-W. Huo and J. Lu, Construction of Hierarchically Porous Nanoparticles@ Metal-Organic Frameworks Composites by Inherent Defects for the Enhancement of Catalytic Efficiency, Adv. Mater., 2018, 30, 1803263 CrossRef PubMed.
- W.-N. Zhang, G. Lu, C.-L. Cui, Y.-Y. Liu, S.-Z. Li, W.-J. Yan, C. Xing, Y.-R. Chi, Y.-H. Yang and F.-W. Huo, A Family of Metal-Organic Frameworks Exhibiting Size-Selective Catalysis with Encapsulated Noble-Metal Nanoparticles, Adv. Mater., 2014, 26, 4056–4060 CrossRef CAS PubMed.
- P. Pachfule, M. K. Panda, S. Kandambeth, S. M. Shivaprasad and R. Banerjee, Multifunctional and robust covalent organic framework-nanoparticle hybrids, J. Mater. Chem. A, 2014, 2, 7944–7952 RSC.
- X. Li, C. Zhang, M. Luo, Q. Yao and Z.-H. Lu, Ultrafine Rh nanoparticles confined by nitrogen-rich covalent organic frameworks for methanolysis of ammonia borane, Inorg. Chem. Front., 2020, 7, 1298–1306 RSC.
- J.-K. Sun, W.-W. Zhan, T. Akita and Q. Xu, Toward Homogenization of Heterogeneous Metal Nanoparticle Catalysts with Enhanced Catalytic Performance: Soluble Porous Organic Cage as a Stabilizer and Homogenizer, J. Am. Chem. Soc., 2015, 137, 7063–7066 CrossRef CAS PubMed.
- X. Yang, J.-K. Sun, M. Kitta, H. Pang and Q. Xu, Encapsulating highly catalytically active metal nanoclusters inside porous organic cages, Nat. Catal., 2018, 1, 214–220 CrossRef CAS.
- Y. Fang, J. Li, T. Togo, F. Jin, Z. Xiao, L. Liu, H. Drake, X. Lian and H.-C. Zhou, Ultra-Small Face-Centered-Cubic Ru Nanoparticles Confined within a Porous Coordination Cage for Dehydrogenation, Chem, 2018, 4, 555–563 CAS.
- J. Yang, D.-H. Guo, S.-L. Zhao, Y. Lin, R. Yang, D.-D. Xu, N.-E. Shi, X.-S. Zhang, L.-Z. Lu, Y.-Q. Lan, J.-C. Bao and M. Han, Cobalt Phosphides Nanocrystals Encapsulated by P-Doped Carbon and Married with P-Doped Graphene for Overall Water Splitting, Small, 2019, 15, 1804546 CrossRef PubMed.
- C. Zhang, J. Liu, Y.-X. Ye, Q. Chen and C.-H. Liang, Encapsulation of Co-based nanoparticle in N-doped graphitic carbon for efficient oxygen reduction reaction, Carbon, 2020, 156, 31–37 CrossRef CAS.
- T.-Y. Zhang, X. Han, H.-B. Yang, A.-J. Han, E.-Y. Hu, Y.-P. Li, X. Q. Yang, L. Wang, J.-F. Liu and B. Liu, Atomically Dispersed Nickel(I) on an Alloy-Encapsulated Nitrogen-Doped Carbon Nanotube Array for High-Performance Electrochemical CO2 Reduction Reaction, Angew. Chem., Int. Ed., 2020, 59, 12055–12061 CrossRef CAS PubMed.
- N.-Y. Cheng, N.-N. Wang, L. Ren, G. Casillas-Garcia, N.-N. Liu, Y.-N. Liu, X. Xu, W.-C. Hao, S.-X. Dou and Y. Du, In-situ grafting of N-doped carbon nanotubes with Ni encapsulation onto MOF-derived hierarchical hybrids for efficient electrocatalytic hydrogen evolution, Carbon, 2020, 163, 178–185 CrossRef CAS.
- Z. Zhao, Z. Zhu, H. Zhang, W. Fang, W. Jiang, S. Lin and Y. Yang, Polyurethane sponge-derived nitrogen-doped carbon-encapsulation composite for enhanced lithium-ion battery performances, Appl. Surf. Sci., 2020, 534, 147631 CrossRef CAS.
- L. Tao, Y. Wang, Y. Zou, N. Zhang, Y. Zhang, Y. Wu, Y. Wang, R. Chen and S. Wang, Charge Transfer Modulated Activity of Carbon-Based Electrocatalysts, Adv. Energy Mater., 2020, 10, 1901227 CrossRef CAS.
- D. A. Bulushev, M. Zacharska, E. V. Shlyakhova, A. L. Chuvilin, Y. Guo, S. Beloshapkin, A. V. Okotrub and L. G. Bulusheva, Single Isolated Pd2+ Cations Supported on N-Doped Carbon as Active Sites for Hydrogen Production from Formic Acid Decomposition, ACS Catal., 2016, 6, 681–691 CrossRef CAS.
- N. Lopez, F. Illas and G. Pacchioni, Adsorption of Cu, Pd, and Cs Atoms on Regular and Defect Sites of the SiO2 Surface, J. Am. Chem. Soc., 1999, 121, 813–821 CrossRef CAS.
- J.-H. Kwak, J. Hu, D. Mei, C.-W. Yi, D.-H. Kim, C. H. F. Peden, L. F. Allard and J. Szanyi, Coordinatively Unsaturated Al3+ Centers as Binding Sites for Active Catalyst Phases of Platinum on γ-Al2O3, Science, 2009, 325, 1670 CrossRef CAS PubMed.
- C. Xie, D.-F. Yan, H. Li, S.-Q. Du, W. Chen, Y.-Y. Wang, Y.-Q. Zou, R. Chen and S.-Y. Wang, Defect Chemistry in Heterogeneous Catalysis: Recognition, Understanding, and Utilization, ACS Catal., 2020, 10, 11082–11098 CrossRef CAS.
- Q.-Y. Bi, J.-D. Lin, Y.-M. Liu, H.-Y. He, F.-Q. Huang and Y. Cao, Dehydrogenation of Formic Acid at Room Temperature: Boosting Palladium Nanoparticle Efficiency by Coupling with Pyridinic-Nitrogen-Doped Carbon, Angew. Chem., Int. Ed., 2016, 55, 11849–11853 CrossRef CAS PubMed.
- B. Wang, Y. Yue, C. Jin, J. Lu and X. Li, Hydrochlorination of Acetylene on Single-Atom Pd/N-Doped Carbon Catalysts: Importance of Pyridinic-N Synergism, Appl. Catal., B, 2020, 272, 118944 CrossRef CAS.
- W. Wang, X. Hong, Q. Yao and Z.-H. Lu, Bimetallic Ni–Pt nanoparticles immobilized on mesoporous N-doped carbon as a highly efficient catalyst for complete hydrogen evolution from hydrazine borane, J. Mater. Chem. A, 2020, 8, 13694–13701 RSC.
- Y. Wang, J. L. Li, W. X. Shi, Z. M. Zhang, S. Guo, R. Si, M. Liu, H. C. Zhou, S. Yao, C. H. An and T. B. Lu, Unveiling Single Atom Nucleation for Isolating Ultrafine fcc Ru Nanoclusters with Outstanding Dehydrogenation Activity, Adv. Energy Mater., 2020, 10, 2002138 CrossRef CAS.
- H. Chu, N. Li, S. Qiu, Y. Zou, C. Xiang, F. Xu and L. Sun, Ruthenium supported on nitrogen-doped porous carbon for catalytic hydrogen generation from NH3BH3 hydrolysis, Int. J. Hydrogen Energy, 2019, 44, 1774–1781 CrossRef CAS.
- M. Navlani-Garcia, D. Salinas-Torres and D. Cazorla-Amoros, Hydrolytic dehydrogenation of ammonia borane attained by Ru-based catalysts: An auspicious option to produce hydrogen from a solid hydrogen carrier molecule, Energies, 2021, 14, 2199 CrossRef CAS.
- Y. Cao, S. Mao, M. Li, Y. Chen and Y. Wang, Metal/Porous Carbon Composites for Heterogeneous Catalysis: Old Catalysts with Improved Performance Promoted by N-Doping, ACS Catal., 2017, 7, 8090–8112 CrossRef CAS.
- R. Arrigo, M. E. Schuster, Z. Xie, Y. Yi, G. Wowsnick, L. L. Sun, K. E. Hermann, M. Friedrich, P. Kast, M. Hävecker, A. Knop-Gericke and R. Schlögl, Nature of the N–Pd Interaction in Nitrogen-Doped Carbon Nanotube Catalysts, ACS Catal., 2015, 5, 2740–2753 CrossRef CAS.
- B. Xia, T. Liu, W. Luo and G. Cheng, NiPt–MnOx supported on N-doped porous carbon derived from metal–organic frameworks for highly efficient hydrogen generation from hydrazine, J. Mater. Chem. A, 2016, 4, 5616–5622 RSC.
- B.-Q. Li, S.-Y. Zhang, B. Wang, Z.-J. Xia, C. Tang and Q. Zhang, A porphyrin covalent organic framework cathode for flexible Zn–air batteries, Energy Environ. Sci., 2018, 11, 1723–1729 RSC.
- B.-Q. Li, S.-Y. Zhang, L. Kong, H.-J. Peng and Q. Zhang, Porphyrin Organic Framework Hollow Spheres and Their Applications in Lithium-Sulfur Batteries, Adv. Mater., 2018, 30, 1707483 CrossRef PubMed.
- B.-Q. Li, S.-Y. Zhang, X. Chen, C.-Y. Chen, Z.-J. Xia and Q. Zhang, One-Pot Synthesis of Framework Porphyrin Materials and Their Applications in Bifunctional Oxygen Electrocatalysis, Adv. Funct. Mater., 2019, 29, 1901301 CrossRef.
- X. Fan, F. Kong, A. Kong, A. Chen, Z. Zhou and Y. Shan, Covalent Porphyrin Framework-Derived Fe2P@Fe4N-Coupled Nanoparticles Embedded in N-Doped Carbons as Efficient Trifunctional Electrocatalysts, ACS Appl. Mater. Interfaces, 2017, 9, 32840–32850 CrossRef CAS PubMed.
- L. J. Boucher and J. J. Katz, The Infared Spectra of Metalloporphyrins (4000–160 cm−1), J. Am. Chem. Soc., 1967, 89, 1340–1345 CrossRef CAS PubMed.
- D. W. Thomas and A. E. Martell, Metal Chelates of Tetraphenylporphine and of Some p-Substituted Derivatives1,2, J. Am. Chem. Soc., 1959, 81, 5111–5119 CrossRef CAS.
- Z. Han, Y. Ai, X. Jiang, Y. You, F. Wei, H. Luo, J. Cui, Q. Bao, J. Fu, Q. He, S. Liu and J. Cheng, Pre-Polymerization Enables Controllable Synthesis of Nanosheet-Based Porphyrin Polymers towards High-Performance Li-Ion Batteries, Chem.–Eur. J., 2020, 26, 10433–10438 CrossRef CAS PubMed.
- H. Yang, S. Zhang, L. Han, Z. Zhang, Z. Xue, J. Gao, Y. Li, C. Huang, Y. Yi, H. Liu and Y. Li, High Conductive Two-Dimensional Covalent Organic Framework for Lithium Storage with Large Capacity, ACS Appl. Mater. Interfaces, 2016, 8, 5366–5375 CrossRef CAS PubMed.
- B. C. Patra, S. Khilari, R. N. Manna, S. Mondal, D. Pradhan, A. Pradhan and A. Bhaumik, A Metal-Free Covalent Organic Polymer for Electrocatalytic Hydrogen Evolution, ACS Catal., 2017, 7, 6120–6127 CrossRef CAS.
- Z.-S. Wu, L. Chen, J. Liu, K. Parvez, H. Liang, J. Shu, H. Sachdev, R. Graf, X. Feng and K. Müllen, High-Performance Electrocatalysts for Oxygen Reduction Derived from Cobalt Porphyrin-Based Conjugated Mesoporous Polymers, Adv. Mater., 2014, 26, 1450–1455 CrossRef CAS PubMed.
- Z. Xie, Z. He, X. Feng, W. Xu, X. Cui, J. Zhang, C. Yan, M. A. Carreon, Z. Liu and Y. Wang, Hierarchical Sandwich-Like Structure of Ultrafine N-Rich Porous Carbon Nanospheres Grown on Graphene Sheets as Superior Lithium-Ion Battery Anodes, ACS Appl. Mater. Interfaces, 2016, 8, 10324–10333 CrossRef CAS PubMed.
- Y. Zhang, Y. Ma, Y.-Y. Chen, L. Zhao, L.-B. Huang, H. Luo, W.-J. Jiang, X. Zhang, S. Niu, D. Gao, J. Bi, G. Fan and J.-S. Hu, Encased Copper Boosts the Electrocatalytic Activity of N-Doped Carbon Nanotubes for Hydrogen Evolution, ACS Appl. Mater. Interfaces, 2017, 9, 36857–36864 CrossRef CAS PubMed.
- X. Cui, Y. Long, X. Zhou, G. Yu, J. Yang, M. Yuan, J. Ma and Z. Dong, Pd-doped Ni nanoparticle-modified N-doped carbon nanocatalyst with high Pd atom utilization for the transfer hydrogenation of nitroarenes, Green Chem., 2018, 20, 1121–1130 RSC.
- R. Zhang, X. Gu, Y. Liu, D. Hua, M. Shao, Z. Gu, J. Wu, B. Zheng, W. Zhang, S. Li, F.-W. Huo and W. Huang, Hydrophilic nano-porous carbon derived from egg whites for highly efficient capacitive deionization, Appl. Surf. Sci., 2020, 512, 145740 CrossRef CAS.
- W. Luo, W. Cheng, M. Hu, Q. Wang, X. Cheng, Y. Zhang, Y. Wang, D. Gao, J. Bi and G. Fan, Ultrahigh Catalytic Activity of L-Proline-Functionalized Rh Nanoparticles for Methanolysis of Ammonia Borane, ChemSusChem, 2019, 12, 535–541 CrossRef CAS PubMed.
- P. Lazar, R. Mach and M. Otyepka, Spectroscopic Fingerprints of Graphitic, Pyrrolic, Pyridinic, and Chemisorbed Nitrogen in N-Doped Graphene, J. Phys. Chem. C, 2019, 123, 10695–10702 CrossRef CAS.
- H. Jiang, L. Liu, K. Zhao, Z. Liu and S. Hu, Effect of pyridinic- and pyrrolic-nitrogen on electrochemical performance of Pd for formic acid electrooxidation, Electrochim. Acta, 2020, 337, 135758 CrossRef CAS.
- C. Wang, J. Tuninetti, Z. Wang, C. Zhang, R. Ciganda, L. Salmon, S. Moya, J. Ruiz and D. Astruc, Hydrolysis of Ammonia-Borane over Ni/ZIF-8 Nanocatalyst: High Efficiency, Mechanism, and Controlled Hydrogen Release, J. Am. Chem. Soc., 2017, 139, 11610–11615 CrossRef CAS PubMed.
- Ö. M. Huriye Erdoğan and S. Özkar, Hydrogen generation from the methanolysis of ammonia borane catalyzed by in situ generated, polymer stabilized ruthenium(0) nanoclusters, Catal. Today, 2011, 170, 93–98 CrossRef.
- D. Özhava and S. Özkar, Nanoceria supported rhodium(0) nanoparticles as catalyst for hydrogen generation from methanolysis of ammonia borane, Appl. Catal., B, 2018, 237, 1012–1020 CrossRef.
- Z. Wang, S. Liang, X. Meng, S. Mao, X. Lian and Y. Wang, Ultrasmall PdAu alloy nanoparticles anchored on amine-functionalized hierarchically porous carbon as additive-free catalysts for highly efficient dehydrogenation of formic acid, Appl. Catal., B, 2021, 291, 120140 CrossRef CAS.
- M. H. Matus, D. J. Grant, M. T. Nguyen and D. A. Dixon, Fundamental thermochemical properties of ammonia borane and dehydrogenated derivatives (BNHn, n = 0-6), J. Phys. Chem. C, 2009, 113, 16553–16560 CrossRef CAS.
- L. R. Peebles and P. Marshall, High-accuracy coupled-cluster computations of bond dissociation energies in SH, H2S, and H2O, J. Chem. Phys., 2002, 117, 3132–3138 CrossRef CAS.
Footnote |
† Electronic supplementary information (ESI) available. See DOI: 10.1039/d1ta06807e |
|
This journal is © The Royal Society of Chemistry 2022 |
Click here to see how this site uses Cookies. View our privacy policy here.