DOI:
10.1039/D2TC01773C
(Paper)
J. Mater. Chem. C, 2022,
10, 11579-11587
Investigations on electron beam irradiated rare-earth doped SrF2 for application as low fading dosimeter material: evidence for and DFT simulation of a radiation-induced phase†
Received
29th April 2022
, Accepted 13th July 2022
First published on 14th July 2022
Abstract
A recent approach to measure electron radiation doses in the kGy range is the use of phosphors with an irradiation dose-dependent luminescence decay time. However, the applicability of the previously investigated material NaYF4:Yb3+,Er3+ is limited as it shows pronounced fading. Therefore, in this work, a modified SrF2 synthesis is presented that results in SrF2 nanoparticles codoped with Yb and either Er, Hm, or Tm. To assess their suitability as dosimeter material, dose response, as well as its degree of fading over 50 up to 140 days after irradiation were measured. Fading rates as small as 5% in SrF2:Er,Yb and 4% in SrF2:Ho,Yb were derived, which are comparable to established dosimeter materials. A combination of spectroscopy, diffraction and DFT calculations was used to elucidate the effect of irradiation, pointing towards the formation of a secondary phase of Yb2+ that we predict could be Yb2OF2. This irreversible formation of a secondary phase is considered to be the explanation for the low fading behavior in SrF2-based phosphors compared to NaYF4:Yb, Er, a highly attractive feature for electron beam dosimetry.
Introduction
Electron beam (e-ebeam) irradiation is nowadays routinely used for food decontamination and other applications like radiation sterilization and enhancement of materials properties as it shows less effect on the quality of the treated products than competing methods.1 Lately, low-energy electron beam treatments to limit the effects to the surface of the products have come into focus,2 and have also been considered for vaccine manufacturing by inactivating pathogens.3 However, because of their shape and thickness, currently available dosimeters based on optical or electron paramagnetic resonance read-out are not fully suited for usage in low energy e-beams and thus, new materials are needed. Some inorganic phosphors are sensitive to ionizing radiation in a way that their luminescence decay time decreases as a result of the irradiation. It was first discovered almost 50 years ago in the phosphor barium ytterbium fluoride BaYb2F8:Er.4–6 The transfer of this effect to the upconversion phosphor sodium yttrium fluoride NaYF4:Er,Yb and the investigation of this material as a potential dosimetric material is, however, relatively new.7,8 Although the precise nature of the radiation-induced effect in NaYF4:Yb3+,Er3+ remains to be clarified, the decrease in luminescence decay time is attributed to a higher probability for non-radiative decay of the 2F5/2 level in Yb3+, in analogy to BaYb2F8:Er.5 Nevertheless, the decay time of the luminescence is independent of its intensity, and, in case the phosphor is used as a dose-sensitive component for dosimetry, the measurement signal, in general, is not dependent on the dosimetric layer thickness or the amount of dosimetry material. This property is beneficial for low-energy e–e-beam dosimetry as, for example, it enables the application of thin dosimetric layers directly onto product surfaces to estimate the surface dose or to measure dose distributions on 3D surfaces.9 However, the strong fading of the dose response hampers the application of NaYF4:Er,Yb as a dosimeter material so far: the luminescence decay time re-increases partially over time after irradiation. Fading occurs so far to all radiation-sensitive materials and is extensively studied, for example, in thermoluminescence materials like calcium fluoride CaF2:RE3+
10 (RE stands for a rare earth element). To improve the applicability of decay time dosimetry, we searched for alternative dose-sensitive phosphors which are subject to less fading than NaYF4:Er,Yb.
Therefore, in this work SrF2:Er,Yb phosphor powders were synthesized, characterized, and the influence of electron radiation on their decay time was studied, as well as the associated fading of the reduced decay time. They are compared to NaYF4:Er,Yb; also, two more dopants are tested in SrF2:Ho,Yb and SrF2:Tm,Yb. We identified a radiation-induced secondary phase formation in doped SrF2:Er,Yb and a change in the fluorescence emission spectra. DFT calculations were employed to predict a stable structure for the secondary phase, and to form a hypothesis on the reason of the low decay time fading behavior of SrF2:Er,Yb. The DFT-based data-driven structural prediction method utilized in this paper has previously been used successfully to forecast crystal structures of phosphors, among many other materials classes.11,12 This approach uses data-mined ionic substitutions to predict new structures: such data-driven approaches are computationally significantly cheaper than exhaustive searches based on global optimization techniques in combination with DFT.13
Results and discussion
SrF2 doped with erbium and ytterbium
Powder samples of sub-micron particles (100 nm size) were prepared by precipitation from aqueous solution, as described in the Experimental section. Fig. 1(a) shows X-ray diffractometry (XRD) data from SrF2 doped with 2 mol% erbium and 2 to 20 mol% ytterbium. All reflections correspond to single phase powders of a fluorite type crystal structure in (JCPDS card 06-02612) and in the literature.14,15 The measured reflections are compared to a PowderCell-simulation using the cell parameter9a = 5.799 Å for 2 mol% Yb3+ and 5.732 Å for 20 mol% Yb3+ (not shown).
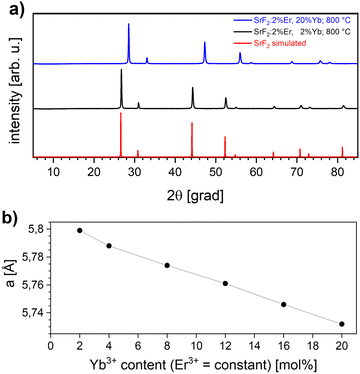 |
| Fig. 1 (a) Representative X-ray diffractograms of SrF2: 2 mol% Er3+, 2 mol% Yb3+ (black line) and SrF2: 2 mol% Er3+, 20 mol% Yb3+ sample (blue line) calcined at 800 °C and TOPAZ-simulation of SrF2 (red line). (b) The calculated cell parameter from XRD-data for calcined SrF2: 2 mol% Er3+, x mol% Yb3+ for x = 2–20 (line guide to the eye). | |
The calculation of the cell parameters is presented in Fig. 1(b): in agreement with literature data,10 a linear decrease of the cell parameter with increasing Yb3+ concentration is observed, which can be attributed to the smaller ion radius of the rare earth ions replacing the divalent Strontium in the unit cell. The phase diagram17 of SrF2–YbF3 shows that a single phase solid solution exists up to 25% YbF3. Upon the excitation with a 980 nm laser beam, the SrF2:Yb3+,Er3+ material exhibits the typical narrow lines from Er3+ upconversion. Fig. 2(a) shows the typical emissions in the range of 537 and 544 nm, which are ascribed to the 2H11/2 → 4I15/2 and 4S3/2 → 4I15/2 transitions,18 and also an emission at 655 nm. With increasing ytterbium concentration, the intensity of the 655 nm emission, originating from the 4F9/2 → 4I15/2 transition, also increases. This occurs because the energy transfer upconversion (ETU) process from 2H11/2 to 4G, 2K is enhanced with higher ytterbium content19 (Fig. 2(b)). The decay times are always lower for the considered upconversion emissions than for those from positive Stokes shifts (as are the emission intensities), and we find the same when comparing our co-doped samples to only Yb3+-doped SrF2.20 Between 2 and 20 at% doping with trivalent ytterbium, a decrease in the decay time of 400 μs oat the upconversion emission was observed (not shown here). A similar decrease of the decay time with the increase of the Yb3+ concentration was described for the case of Yb3+ fluorescence.20 The emission intensity at 544 nm is most intense with 2 at% erbium co-doping, while concentrations of 1, 2, 3, and 5 at% were investigated.
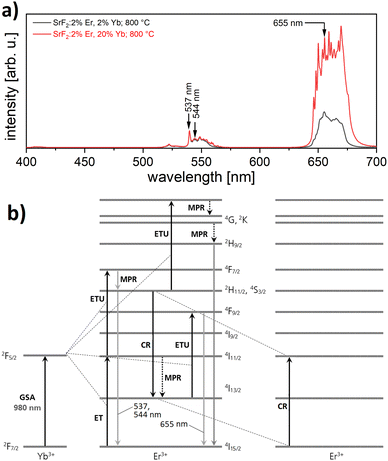 |
| Fig. 2 (a) Luminescence spectra of SrF2:Er,Yb powder (visible range) upon excitation with 980 nm laser light. The spectra are normalized to the peak at 540 nm. (b) Model of the well-known UC process in SrF2:Er3+,Yb3+ (adopted from Lyapin et al.18). GSA: ground state absorption, MPR: multi-phonon relaxation, CR: cross relaxation. | |
Fig. 3(a) and (b) show the field emission scanning electron microscopy (FE-SEM) images of the material, as precipitated and calcined at 800 °C, respectively. Agglomerated particles of approximately 100 nm size can be seen. Particle size decreases slightly after calcination and XRD reflections become sharper (data not shown) as a result of improved crystallinity and a decrease of microstrains.16 Annealing temperatures of 600, 700, and 800 °C were used for our powders, giving almost identical emission intensities. Higher temperatures than that already led to degradation of SrF2 in air. This agrees very well with the observations of the thermoluminescence on ceramic pellets in the literature.21
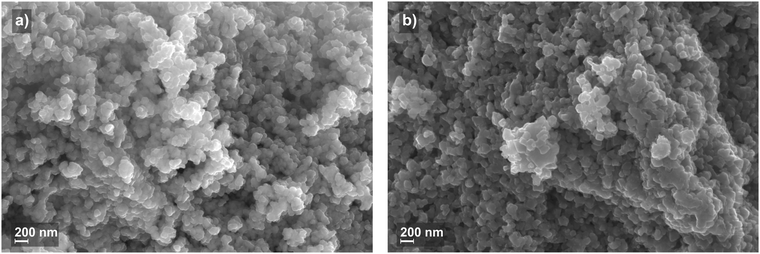 |
| Fig. 3 FE-SEM micrographs of SrF2: 2 mol% Er3+, 2 mol% Yb3+. (a) The precipitated material and (b) the at 800 °C calcined material. | |
After electron beam irradiation (200 keV) of a thin immobilized phosphor layer, the luminescence decay time of the SrF2: 2%Er, 2%Yb phosphor decreases from 1520 μs to values between 1250 and 1020 μs, depending on the irradiation dose, as shown in Fig. 4(a). For doses up to 10 kGy, a strong dose dependence is evident that becomes weaker as the irradiation increases further and seems to saturate above 50 kGy. The initial (i.e. measured within minutes after irradiation) decay time reduction for 50 kGy amounts to 30%. Comparing these results with the dose response of NaYF4:Er,Yb (purchased from Honeywell; refer to Reitzig et al.8 for the dose reponse curve), we see the following: (1) the shapes of the dose response curves are comparable. (2) NaYF4:Er,Yb shows a greater initial decrease of its decay time upon radiation (90% for 50 kGy).
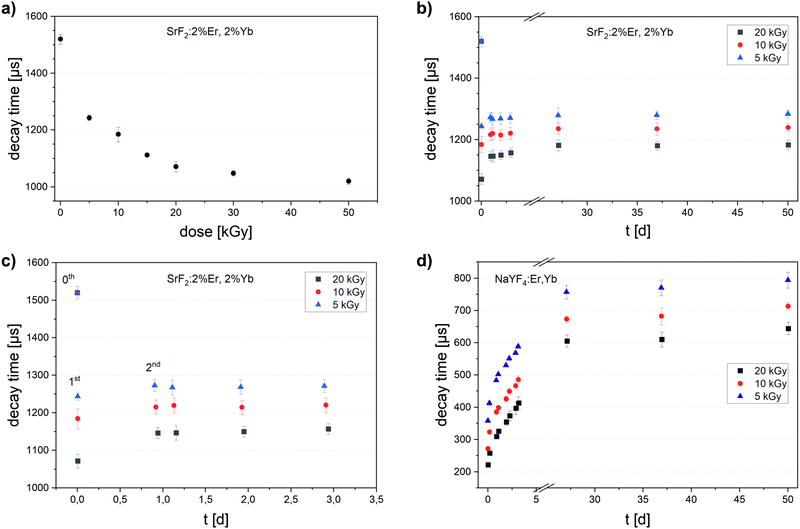 |
| Fig. 4 (a) Luminescence decay times of SrF2: 2 mol% Er3+, 2 mol% Yb3+ after 200 keV electron irradiation with the given doses (dose response curve). (b) Luminescence decay times of SrF2: 2 mol% Er3+, 2 mol% Yb3+ irradiated with 5, 10 and 20 kGy up to 50 days after irradiation (fading curve). (c) The same fading curve as before, shown only for the first three days (0th measurement represents decay times before irradiation). (d) Fading curve of NaYF4:Er,Yb up to 50 days after irradiation for comparison; decay time before irradiation is 1224 μs ± 8 μs (not shown in the diagram). | |
For SrF2:Er,Yb samples irradiated with 5, 10 and 20 kGy, the luminescence decay times were repeatedly measured until 50 days after irradiation (Fig. 4(b) and (c)). They increased in the first day of storage at 20 °C (first and second measurements) towards the initial value, but, during the following two days, the decay times remained almost constant. Looking into the decay times 25 to 50 days after irradiation (Fig. 4(b)), they only slightly increased compared to day 2 and 3. Furthermore, between the 25th and 50th day, the decay time did not rise anymore, and the fading therefore appeared to have finished. On the other hand, we can see that the decay time of NaYF4:Er,Yb rose strongly in the first days after irradiation (Fig. 4(d)). It still rose between the 25th and 50th day, although slower and to values much lower than the unirradiated sample (650–800 μs vs. 1220 μs initially).
Clearly, one day after irradiation, the decay time of SrF2:Er,Yb was already close to the long-term value and could be read out in a dosimeter with sufficient precision. In fact, after 50 days of storage, the decay time of SrF2:Er,Yb had increased by only 5% with respect to the first measurement after irradiation. This degree of fading is comparable to established dosimeter materials, e.g. 2–5% in alanine over 100 days after 14 kGy irradiation.22 For a possible application as an electron radiation dosimetry material, this very low fading of SrF2:Er,Yb outweighs the lower dose resolution compared to NaYF4:Er,Yb.
SrF2 doped with holmium/ytterbium and thulium/ytterbium
To build on these results, Sr1−x–yHoxYbyF2 and Sr1−x−yTmxYbyF2 powders were synthesized in the same way as SrF2:Er,Yb. The effect of very low fading could also be observed in Ho/Yb and Tm/Yb codoping.
The optimum concentration of the dopants Yb and Ho or Tm for highest upconversion emission intensity (Fig. 5) was determined for x = 0.02, y = 0.2 (for Ho and Yb) and x = 0.01, y = 0.2 (for Tm and Yb). All subsequent measurements were carried out with these doping concentrations. The powders were calcined at 600 °C. Fig. 6(a) and (b) show the X-ray diffractograms of the samples Sr0.78Ho0.02Yb0.2F2 and Sr0.79Tm0.01Yb0.2F2 in comparison with the simulated SrF2 diffractogram. Despite the high contents of trivalent rare earth ions, no secondary phases could be observed. This corresponds well to the literature18,23 in which no phase changes were observed for RE doping concentrations up to 20 mol% for the calcination temperature of 600 °C used here.
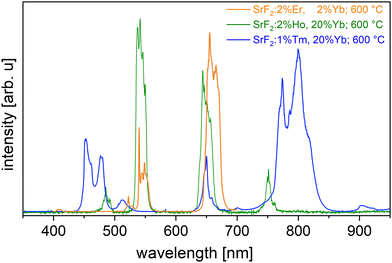 |
| Fig. 5 UC emission of Yb doped SrF2 codoped with Er (orange line), Holmium (green line) and Thulium (blue line). | |
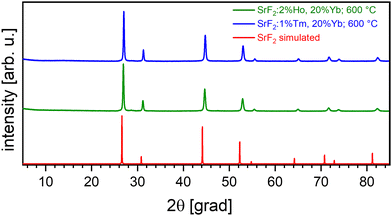 |
| Fig. 6 X-ray diffractograms of Yb-doped Strontium fluoride codoped with Ho (green) and Tm (blue). | |
The dependence of the cell parameter on the Yb content for codoped SrF2 is shown in Table 1. A shift to higher diffraction angles is observable (Fig. 6) with respect to the simulated SrF2 diffractogram.
Table 1 Change of the cell parameter a with Yb doping level and different codopants
Codoping |
None |
Er (2 mol%) |
Ho (2 mol%) |
Tm (1 mol%) |
Yb (at %) |
None |
2 |
20 |
2 |
20 |
1 |
20 |
a (Å) |
5.799 |
5.789(4) |
5.736(4) |
5.782(2) |
5.737(6) |
5.782(4) |
5.738(3) |
After electron beam irradiation (200 keV) of a thin immobilized phosphor layer, the luminescence decay time of the SrF2:Ho,Yb and SrF2:Tm,Yb phosphors decrease from 95 and 104 μs, respectively, to values which are 15 to 30 μs lower, depending on the irradiation dose, as shown in Fig. 7(a). The shape of the dose response curve is comparable to SrF2:Er,Yb (Fig. 4(a)). The initial relative decay time reduction for 50 kGy amounts to 29% for SrF2:Ho,Yb and 27% for SrF2:Tm,Yb phosphor, similar to the 30% for SrF2:Er,Yb.
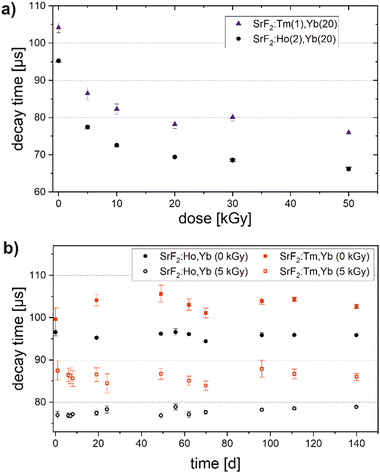 |
| Fig. 7 (a) Dose response of the decay time of thin layers of SrF2 powder codoped with Tm, Yb or Ho, Yb upon 200 keV electron radiation. (b) Luminescence decay times of SrF2:Tm,Yb and SrF2:Ho,Yb phosphor samples (unirradiated and irradiated with 5 kGy) over the course of 140 days starting from day 1 after irradiation. | |
For samples irradiated with 5 kGy and for unirradiated samples the luminescence decay times were repeatedly measured until 140 days after irradiation (Fig. 7(b)). Measurements on SrF2:Tm,Yb suffer from significantly higher uncertainties compared to SrF2:Ho,Yb despite the same processing of the powders and treatment of the samples. For Ho, Yb codoped SrF2, we see all decay times lying within a range of 3 μs which corresponds to about 4% of the mean value measured after 5 kGy irradiation. This is, therefore, the upper estimation for the measured fading over 140 days, as no trend is observed in the time series. That is even lower than in SrF2:Er,Yb, although with Er, a higher dose resolution was achieved.
Radiation-induced changes in doped SrF2
After irradiation of the SrF2:Er,Yb samples, we observed new reflections in their powder XRD (Fig. 8), likely from a newly formed secondary phase. The reference material NaYF4:Er,Yb shows no additional reflections after the same irradiation dose (data not shown). Moreover, fluorescence spectroscopy measurements were performed on irradiated and non-irradiated powders, using an excitation wavelength of 365 nm and fluorescence detection in the visible range (Fig. 9). We observe an emission band between 400 nm and 450 nm (peak at 421 nm) and another one between 460 nm and 700 nm (peak at about 560 nm) for irradiated powders. Both emissions are only barely observed in unirradiated powders. Based on literature data,24–26 we assign the peak at 421 nm to the regular emission 4f13 5d → 4f14 of Yb2+. The literature data on the spectral position of this emission ranges from 412 nm25 to 447 nm.26 Differences can be attributed to the varying crystal field in different host lattices and its effect on the excited d-levels of Yb2+. The second, broader emission band can be attributed to the inter valence charge transfer (IVCT) emission of Yb in SrF2 which is described as the transition Yb2+ [2F5/2 Eg (5d)–2A1u] + Yb3+ –> Yb3+ [2F7/2–G7u] + Yb2+ [4f14–A1g] at 521 nm.24
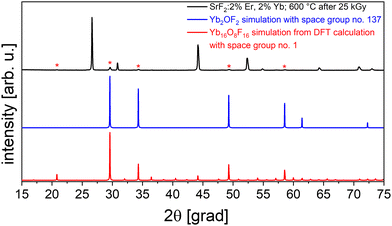 |
| Fig. 8 X-ray diffraction pattern of SrF2: 2%Er,2% Yb after irradiation (top, black; 10 MeV electron radiation, 25 kGy) with reflections observed only after irradiation marked with asterisks. Calculated X-ray diffraction pattern of the structure derived from Sm2OF2 (blue), and from the DFT calculation after refinement (red, structure 1 derived from Mn2OF2). | |
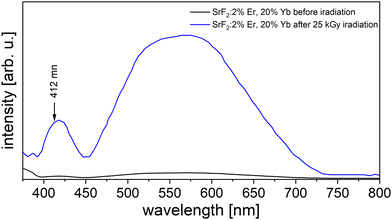 |
| Fig. 9 Fluorescence emissions spectra of 25 kGy irradiated (blue) and non-irradiated (black) SrF2 with 2 mol% Er and 20 mol% Yb. | |
Apparently, electron irradiation of SrF2:Er,Yb led to the reduction of Yb3+ to Yb2+ (as observed e.g. upon irradiation in Yb3+-doped CaF227) and to the formation of a secondary crystalline phase.
Returning to the XRD again, the new reflections were all weak in intensity compared to doped SrF2 but fit well to a cubic indexation with a volume per formula unit V/FU = 150 Å3. The reflections did not fit to known, plausible compounds of both Yb2+ or Yb3+; in fact, YbO is smaller (V/FU = 103 Å3)28 while YbF2 is larger (V/FU = 175 Å3)29 than the observed phase. The formation of an oxide fluoride with an intermediate volume is thinkable: the powders were irradiated under air, and Yb3+ forms the well-described YbOF30 (that however does not fit). Using the OQMD (Open Quantum Materials Database),31 we identified the structure of Sm2OF2 as a possible starting point for the radiation-induced phase in SrF2:Er,Yb. After substituting Sm by Yb and refining the cell parameters, the calculated reflections of the hypothetical structure principally match the measured ones (see Fig. 8, blue graph). However, there is a weak reflection in the measurements at 21° that cannot be explained by this structural model.
At this point, we turned to ab initio simulations in our search for a structural understanding of the secondary phase formed under irradiation. Thus, we performed DFT-based harmonic phonon computations and obtained additional structural models for Yb2OF2 from the Materials Project.32 The computation of phonon band structures allows to confirm the dynamic stability of a material and thus to estimate the plausibility of a structural model. Further computational details can be found in the Method section and the ESI.†
As the result, among five candidates we found a dynamically stable structure based on a Mn2OF2 prediction32 that is also the energetically most stable (see Table S1 in ESI† for further details). VESTA33 was used to visualize its crystal structure, as seen in Fig. 10. With this structure predicted from DFT, after refinement of the cell parameters, we obtain a very good agreement of calculated and measured reflections in the XRD pattern (Fig. 8, red curve). The proposed compound is triclinic with a cell volume of 598 Å3 and 4 formula units per elementary cell. This is in close agreement with our earlier assumption that the normalized cell volume should be approximately 150 Å3.
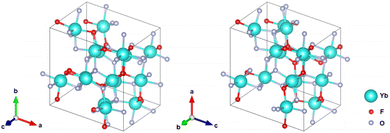 |
| Fig. 10 Most stable predicted structure for Yb2OF2 by the DFT calculations as visualized by VESTA.33 | |
The combined interpretation of our measurements and simulations indicates that Yb3+ in SrF2:Er,Yb is partially reduced by the electron beam, and (some) Yb2+ segregates to form a secondary phase. Likely, this involves a reaction with air to form Yb2OF2, isostructural to Mn2OF2, but this must be verified independently. In any case, the formation of a secondary phase seems to be responsible for the irreversible change in the decay time of SrF2:Er,Yb (at least in the time frame of our study).
Experimental
Synthetic procedures
To synthesize different rare-earth doped SrF2 powders, we followed the procedure from ref. 16. Sr(NO3)2 (Sigma-Aldrich, purity 99.98%), Yb(NO3)3·6H2O (Sigma-Aldrich, purity 99.99%), an additional rare earth nitrate (all Sigma Aldrich purity 99.99%), and distilled water were used as starting materials without additional purification. The additional RE nitrate used was either Er(NO3)3·5H2O, Ho(NO3)3·5H2O or Tm(NO3)3·5H2O. As precipitant, ammonium fluoride (MerckMillipore) was used. Aqueous solutions of nitrates (0.08 M) and a 0.16 M aqueous solution of ammonium fluoride (for 2% Yb 7% molar excess10,16 and 3-fold excess for 20% Yb19) were prepared by mixing the aforementioned salts with deionized water. The ammonium fluoride solution was added dropwise to the solution of nitrates under vigorous stirring: the formed suspension was stirred for 3 hours and then washed with a dilute solution of NH4F. Finally, the suspension was centrifuged at 5000 rpm, and the precipitate recovered and dried at 40 °C for 6 h. Calcination of the material was carried out at 800 °C (SrF2:Er,Yb) and 600 °C (SrF2:Ho,Yb; SrF2:Tm,Yb) in air.
Optical methods
Thin phosphor film preparation.
Thin layers containing the phosphor particles were prepared as follows: phosphor powder was pestled in a mortar to break down agglomerations. Then, a varnish was prepared by first suspending the particles in ethanol and acetone, then adding a dispersing agent (Tego Dispers 755 W) and finally polyvinyl acetate (molecular weight approx. 500
000 g mol−1) as binding agent and film former. Several single drops of a few μl were pipetted onto glass substrates to obtain phosphor particles immobilized in a thin layer that can be penetrated by the 200 keV electron beam radiation. The layer thickness was 50 to 60 μm.
Irradiation.
The thin film samples were irradiated in an EBLab 200 electron irradiator (Comet) using 200 kV acceleration voltage. The air gap between the exit window of the electron radiation and the sample surface was 10 mm. Doses of 5 kGy up to 50 kGy were applied to the samples. Powder samples for X-ray diffractometry were irradiated at 10 MeV in a Van de Graaff electron beam accelerator.
Luminescence decay time measurements: to measure luminescence decay curves, excitation of the phosphor samples was carried out with a 1 ms light pulse from a 980 nm laser diode (max. 80 mW, Roithner) and the temporal luminescence decay was recorded by a silicon photodiode (Osram SFH2200). Note that no spectral filter is built into the detection path and, therefore, both visible UC and near-IR fluorescence emission contribute to the signal. Ten consecutive curves were averaged and then exponentially fitted to obtain the decay time. To assess the fading behavior after electron irradiation, we repeatedly measured the decay times of the samples in a time lapse of up to 140 days, starting with the first measurement immediately after irradiation. These first measurements were used to obtain the dose response curve. Between the measurements, the samples were stored in the dark at room temperature. The luminescence spectra were acquired by the excitation of a pressed powder sample with 980 nm continuous wave laser light and recording the emitted light using a spectrometer (Horiba iHR 550).
Fluorescence spectroscopy.
Yb2+-fluorescence in SrF2 was measured with a fluorescence spectrometer (Edinburgh Inst. FLS 920). Emission spectra were recorded upon the excitation at 365 nm.25
X-Ray and electron microscopy method
X-Ray.
The X-ray phase analyses were carried out using a Bragg–Brentano X-ray diffractometer Bruker D8 Advance in reflection geometry. Powder samples were installed in the rotating sample holder. Monochromatic Cu Kα radiation (λ = 0.15406 nm) with an excitation voltage of 40 kV and a current of 30 mA were used. The radiation was diffracted on the sample and registered by an energy-dispersive 1D detector (LYNXEYE XE). There are constructive and destructive interferences depending on the measured angle (0.02 2θ-steps). The recorded reflections in the measuring range 5 ≤ 2θ ≤ 90 were assigned and evaluated by comparison with databases of known structures in the form of comparison line diagrams (EVA evaluation software DIFFRAC.EVA version 3.0 from Bruker).
Electron microscopy.
Powder particles were carefully deposited onto a conductive tape, and then coated with an approx. 10 nm thick carbon layer. In the field emission electron microscope (Zeiss Extremist 55 plus), the particle morphology was well recognizable by means of secondary electrons (SEM, topography contrast). The images were acquired with 10.00 kV acceleration voltage and a working distance of WD = 6.8 mm, using an InLens detector.
DFT computations.
We created structural models for Yb2OF2 based on chemically similar structures from the OQMD and the Materials Project. Some of the structures were chosen based on similar ionic radii of the cations, others were created with the algorithm described by Hautier et al.13 We optimized the structures with periodic DFT in VASP34–37 with strict convergence criteria. After the optimization, we derived five different structures (see ESI,† Fig. S1) using the PBE functional for all calculations.38 All k-point settings were converged and can be found in the raw data ESI.† For electronic and structural optimization, a criterion of ΔE < 10−7 eV and ΔE < 10−5 eV per cell was used, respectively. Once the optimized structures were obtained, the harmonic interatomic force constants were computed using the finite displacement method as implemented in Phonopy,39 with a displacement of 0.01 Å and with the help of supercell of the optimized cell (with cell parameters as close as possible to 20 Å or larger in each direction to ensure convergence). The forces for this evaluation were computed at the Γ-point. The depicted band structures have not been corrected with the non-analytical term correction around the Gamma point as this is not decisive for predicting dynamical stability. Robocrystallographer40 and ChemEnv41 were used to help describing and understanding the newly predicted structures. More information on the other structures can be found in the ESI.†
Conclusion
Considering the importance of the reliability of a dosimeter signal after irradiation, in this work, a series of SrF2-based phosphors was synthesized aiming for their application as low-fading dosimeter materials. To this end, sub-micron SrF2 powders codoped with Yb3+ sensitizer and different activator ions (Er3+, Ho3+ and Tm3+) were synthesized by the precipitation from aqueous solutions and calcination, and their crystal structures were characterized. Encouragingly, the SrF2 materials show a dose-dependent reduction of their luminescence decay time and – most important – very low fading of the luminescence decay time compared to the previously studied NaYF4:Yb3+,Er3+. Upon irradiation, we observed the formation of Yb2+ from spectroscopy and a secondary phase from XRD. Together with DFT calculations, we suggest that this phase could be Yb2OF2 and predict a stable structure of it. The formation of this secondary phase could be the reason for the irreversible change in decay time, i.e. low fading. Therefore, future investigations will focus on a detailed multifactorial study of parameters like dose, dose rate, radiation energy and irradiation modality to further evaluate this promising dosimeter material.
Conflicts of interest
There are no conflicts to declare.
Acknowledgements
The results were acquired within the “SensorHit” project (03VP05751 and 03VP05752), funded by the German Federal Ministry of Education and Research (BMBF). The authors acknowledge Marisa Rio's support in the measurement of luminescence decay series. Florent Kuntz (Aerial, France) provided the 10 MeV electron irradiation. J. G. and A. N. gratefully acknowledge the Gauss Centre for Supercomputing e.V. (http://www.gauss-centre.eu) for funding this project by providing computing time on the GCS Supercomputer SuperMUC-NG at Leibniz Supercomputing Centre (http://www.lrz.de) (project pn73da).
References
- H. Aisala, H. Nygren, T. Seppänen-Laakso, R.-L. Heiniö, M. Kießling, K. Aganovic, A. Waser, H. Kotilainen and A. Ritala, Comparison of low energy and high energy electron beam treatments on sensory and chemical properties of seeds, Food Res. Int., 2021, 148, 110575 CrossRef CAS PubMed.
- Y. Zhang, N. Huber, R. Moeller, J. Stülke, B. Dubovcova, G. Akepsimaidis, N. Meneses, D. Drissner and A. Mathys, Role of DNA repair in Bacillus subtilis spore resistance to high energy and low energy electron beam treatments, Food Microbiol., 2020, 87, 103353 CrossRef CAS PubMed.
- J. Fertey, M. Thoma, J. Beckmann, L. Bayer, J. Finkensieper, S. Reißhauer, B. S. Berneck, L. Issmail, J. Schönfelder, J. P. Casado, A. Poremba, F.-H. Rögner, B. Standfest, G. R. Makert, L. Walcher, A.-K. Kistenmacher, S. Fricke, T. Grunwald and S. Ulbert, Automated application of low energy electron irradiation enables inactivation of pathogen- and cell-containing liquids in biomedical research and production facilities, Sci. Rep., 2020, 10, 12786 CrossRef CAS PubMed.
- M. R. Farukhi and C. F. Swinehart, Barium fluoride as a gamma ray and charged particle detector, IEEE Trans. Nucl. Sci., 1971, 18, 200–204 CAS.
- G. Ban and H. N. Hersh, Degradation of some IR upconverting phosphors by ionizing radiation, J. Electron. Mater., 1972, 320–332 CrossRef CAS.
- H. N. Hersh and G. Ban, X-ray—induced variation of time constant of Yb3+ sensitizer, Appl. Phys. Lett., 1972, 20, 101–102 CrossRef CAS.
- M. Reitzig, M. Winkler, T. Härtling, O. Röder and J. L. Opitz, Evaluation of low energy electron beam dose application by means of a portable optical device, Opt. Eng., 2014, 53, 1–3 CrossRef.
- M. Reitzig, R. J. Goodband, C. Schuster and T. Härtling, Optical electron beam dosimetry with ceramic phosphors as passive sensor material for broad dose ranges, Tech. Mess., 2016, 83, 174 Search PubMed.
- C. Schuster, F. Kuntz, D. Cloetta, M. Zeller, J. Katzmann, A. Strasser, T. Härtling and M. Lavalle, Depth dose curve and surface dose measurement with a μm thin dosimetric layer, Radiat. Phys. Chem., 2022, 193, 109881 CrossRef CAS.
- G. Wang, Q. Peng and Y. Li, Upconversion luminescence of monodisperse CaF2:Yb3+/Er3+ nanocrystals, J. Am. Chem. Soc., 2009, 131, 14200–14201 CrossRef CAS PubMed.
- Z. Wang, J. Ha, Y. H. Kim, W. B. Im, J. McKittrick and S. P. Ong, Mining unexplored chemistries for phosphors for high-color-quality white-light-emitting diodes, Joule, 2018, 2, 914–926 CrossRef CAS.
- J. George and G. Hautier, Chemist versus machine: Traditional knowledge versus machine learning techniques, Trends Chem., 2021, 3, 86–95 CrossRef CAS.
- G. Hautier, C. Fischer, V. Ehrlacher, A. Jain and G. Ceder, Data mined ionic substitutions for the discovery of new compounds, Inorg. Chem., 2011, 50, 656–663 CrossRef CAS PubMed.
- P. P. Fedorov, A. A. Alexandrov, V. V. Voronov, M. N. Mayakova, A. E. Baranchikov and V. K. Ivanov, Low-temperature phase formation in the SrF2–LaF3 system, J. Am. Ceram. Soc., 2021, 104, 2836–2848 CrossRef CAS.
- W. Li, B. Mei, J. Song and Z. Wang, Fabrication and optical property of highly transparent SrF2 ceramic, Mater. Lett., 2015, 159, 210–212 CrossRef CAS.
- S. Kuznetsov, Y. Ermakova, V. Voronov, P. Fedorov, D. Busko, I. A. Howard, B. S. Richards and A. Turshatov, Up-conversion quantum yields of SrF2:Yb3+,Er3+ sub-micron particles prepared by precipitation from aqueous solution, J. Mater. Chem. C, 2018, 6, 598–604 RSC.
- S. V. Kuznetsov and P. P. Fedorov, Morphological stability of solid-liquid interface during melt crystallization of M1−xRxF2+x solid solutions, Inorg. Mater., 2008, 44, 1434–1458 CrossRef CAS.
- A. A. Lyapin, P. A. Ryabochkina, S. V. Gushchin, S. V. Kuznetsov, M. V. Chernov, V. Y. Proydakova, V. V. Voronov and P. P. Fedorov, Upconversion luminescence of fluoride phosphors SrF2:Er,Yb under laser excitation at 1.5 μm, Opt. Spectrosc., 2018, 125, 537–542 CrossRef CAS.
- D. Przybylska, A. Ekner-Grzyb, B. F. Grześkowiak and T. Grzyb, Upconverting SrF2 nanoparticles doped with Yb3+/Ho3+, Yb3+/Er3+ and Yb3+/Tm3+ ions – optimisation of synthesis method, structural, spectroscopic and cytotoxicity studies, Sci. Rep., 2019, 9, 8669 CrossRef PubMed.
- Y. Gao, B. Mei, W. Li, Z. Zhou and Z. Liu, Effect of Yb3+ concentration on microstructure and optical properties of Yb: SrF2 transparent ceramics, Opt. Mater., 2020, 105, 109869 CrossRef CAS.
- M. S. Bhadane, K. H. Gavhane, V. S. Ghemud, S. S. Dahiwale, P. S. Patil, A. Kshirsagar and S. D. Dhole, A post annealing effect on SrF2 nano particles: Structural, morphological, functional and dosimetric properties, J. Alloys Compd., 2020, 846, 156343 CrossRef CAS.
- T. Kojima, L. Chen, Y. Haruyama, H. Tachibana and R. Tanaka, Fading characteristics of an alanine-polystyrene dosimeter, Appl. Radiat. Isot., 1992, 43, 863–867 CrossRef CAS.
- I. Nicoara, L. Lighezan, M. Enculescu and I. Enculescu, Optical spectroscopy of Yb2+ ions in YbF3-doped CaF2 crystals, J. Cryst. Growth, 2008, 310, 2026–2032 CrossRef CAS.
- Z. Barandiarán and L. Seijo, Intervalence charge transfer luminescence: Interplay between anomalous and 5d–4f emissions in Yb-doped fluorite-type crystals, J. Chem. Phys., 2014, 141, 234704 CrossRef PubMed.
- M. Suta, W. Urland, C. Daul and C. Wickleder, Photoluminescence properties of Yb(2+) ions doped in the perovskites CsCaX3 and CsSrX3 (X = Cl, Br, and I) – a comparative study, Phys. Chem. Chem. Phys., 2016, 18, 13196–13208 RSC.
- M. Suta, T. Senden, J. Olchowka, M. Adlung, A. Meijerink and C. Wickleder, Decay times of the spin-forbidden and spin-enabled transitions of Yb2+ doped in CsCaX3 and CsSrX3 (X = Cl, Br, I), Phys. Chem. Chem. Phys., 2017, 19, 7188–7194 RSC.
- D. S. McClure and Z. Kiss, Survey of the spectra of the divalent rare-earth ions in cubic crystals, J. Chem. Phys., 1963, 39, 3251–3257 CrossRef CAS.
- O. Reckeweg and F. J. DiSalvo, Single-crystal structure refinement of YbF2 with a remark about YbH2, Z. Naturforsch., B: J. Chem. Sci., 2017, 72, 995–998 CrossRef CAS.
- A. Goto, Y. Ohta and M. Kitayama, Solid-state synthesis of metastable ytterbium(II) oxide, J. Mater. Sci. Chem. Eng., 2018, 6, 85 CAS.
- T. Wen, Y. Zhou, Y. Guo, C. Zhao, B. Yang and Y. Wang, Color-tunable and single-band red upconversion luminescence from rare-earth doped Vernier phase ytterbium oxyfluoride nanoparticles, J. Mater. Chem. C, 2016, 4, 684–690 RSC.
- The Open Quantum Materials Database, https://oqmd.org/.
- The Materials Project database, https://materialsproject.org/.
- K. Momma and F. Izumi, VESTA 3 for three-dimensional visualization of crystal, volumetric and morphology data, J. Appl. Crystallogr., 2011, 44, 1272–1276 CrossRef CAS.
- G. Kresse, J. Furthmüller and J. Hafner, Theory of the crystal structures of selenium and tellurium: The effect of generalized-gradient corrections to the local-density approximation, Phys. Rev. B: Condens. Matter Mater. Phys., 1994, 50, 13181 CrossRef CAS PubMed.
- G. Kresse and J. Hafner,
Ab initio molecular dynamics for liquid metals, Phys. Rev. B: Condens. Matter Mater. Phys., 1993, 47, 558–561 CrossRef CAS PubMed.
- G. Kresse and J. Furthmüller, Efficiency of ab initio total energy calculations for metals and semiconductors using a plane-wave basis set, Comput. Mater. Sci., 1996, 6, 15–50 CrossRef CAS.
- G. Kresse and J. Furthmüller, Efficient iterative schemes for ab initio total-energy calculations using a plane-wave basis set, Phys. Rev. B: Condens. Matter Mater. Phys., 1996, 54, 11169 CrossRef CAS PubMed.
- J. P. Perdew, K. Burke and M. Ernzerhof, Generalized gradient approximation made simple, Phys. Rev. Lett., 1996, 77, 3865 CrossRef CAS PubMed.
- A. Togo and I. Tanaka, First principles phonon calculations in materials science, Scr. Mater., 2015, 108, 1–5 CrossRef CAS.
- A. M. Ganose and A. Jain, Robocrystallographer: Automated crystal structure text descriptions and analysis, MRS Commun., 2019, 9, 874–881 CrossRef CAS.
- D. Waroquiers, J. George, M. Horton, S. Schenk, K. A. Persson, G.-M. Rignanese, X. Gonze and G. Hautier, ChemEnv: A fast and robust coordination environment identification tool, Acta Crystallogr., Sect. B: Struct. Sci., Cryst. Eng. Mater., 2020, 76, 683–695 CrossRef CAS PubMed.
|
This journal is © The Royal Society of Chemistry 2022 |
Click here to see how this site uses Cookies. View our privacy policy here.