DOI:
10.1039/D2AN01516A
(Paper)
Analyst, 2023,
148, 175-181
Visualizing the distribution of curcumin in the root of Curcuma longa via VUV-postionization mass spectrometric imaging†
Received
14th September 2022
, Accepted 14th November 2022
First published on 18th November 2022
Abstract
Curcumin is a dietary spice and coloring agent widely used in food and herbal medicine. Herein, we visualized the distribution of curcumin in fresh Curcuma longa (turmeric) root sections using the state-of-the-art vacuum-ultraviolet (VUV, 118 nm) single photon-postionization mass spectrometric imaging method. Compared with other mass spectrometric imaging methods, the proposed method does not require any sample pre-treatment. The proposed approach could be more conducive to in situ detection of small molecules. The mass spectroscopic imaging (MSI) images of curcumin sections with a lateral resolution of 100 μm indicated that the concentrations of curcumin decreased from the phloem to the xylem of the root. We also show MS imaging of curcumin in the turmeric root at different maturity periods, revealing the transformation of this endogenous species. The result of quantitative analysis indicates that the total curcumin content of the mature turmeric root is estimated to be 3.43%, which is consistent with the previous report that the content of curcumin in the turmeric root is estimated between 3% and 5%. The report indicated that the proposed method of VUV single photon postionization MSI can be used to explore the metabolic process of plants, which is critical for herbal farming, harvest, and its ingredient extraction.
1. Introduction
Curcuma longa, which is also called turmeric, is a widely cultivated herbaceous plant related to the ginger family (Zingiberaceae).1 It is well known that a large amount of curcumin and other physiologically active substances are contained in its root, which is widely used in food seasoning, edible pigments, medicinal materials, and other dietary fields. Curcumin and its analogues are acidic polyphenols whose main chains are unsaturated aliphatic and aromatic groups. It is medically proven that it has antioxidant,2,3 anti-inflammatory,4 anti-coagulant, lipid-lowering, antiseptic,5 and anti-aging properties to eliminate free radicals and inhibit tumor growth6,7 and other pharmacological effects.
It is known that time-consuming and tedious sample pre-treatment processes, including extraction, centrifugation, and concentration,8,9 are required in detecting medical ingredients in herbal plants for traditional methods, such as gas chromatography-mass spectrometry (GC-MS)10 and liquid chromatography-mass spectrometry (LC-MS).11 Although the quantitative analysis of endogenous components in plant tissues can be obtained by these two methods, their pre-treatment destroys the integrity of plant tissues and makes them unable to provide more intuitive information about plant tissues, such as the initial distribution of target molecules in the plant tissues. In order to better understand the metabolism and pharmacological effects of herbal medicine, in situ detection of active ingredients in plant tissues is particularly important.12,13 Moreover, in situ detection can display the distribution of target molecules in the whole plant tissue and reveal the transport routes of nutrients during metabolism. Generally, the sampling methods in the field of mass spectral analysis of plant ingredients mainly include desorption electrospray ionization (DESI),14 plasma-jet,15 and methods based on laser technology including laser ablation-dielectric barrier discharge ionization (LA-DBDI),16 laser ablation-electrospray ionization (LA-ESI)17 and laser desorption ionization (LDI).18
Matrix-assisted laser desorption/ionization-mass spectrometry (MALDI-MS) has long been used in in situ detection of biological macromolecules in animal and plant tissues, such as proteins, polysaccharides, lipids, metabolites and other biological macromolecules.19–21 (Its extremely high resolution and sensitivity make MALDI-MS widely popular in the field of MSI.22) In recent years, many research groups have used this technology to study the biological macromolecules of living organisms, for instance, MALDI imaging analysis of breviscapine and its metabolites in mouse kidneys23 and visualizing the spatial distribution of endogenous molecules in wolfberry fruit at different development stages.24 Although MALDI-MSI has become popular in the detection of biological macromolecules, its capability to analyze low molecular weight compounds (m/z < 500) is limited because of the interferences of the added organic matrix in this mass range. It is easy to interfere the mass spectral peaks when low molecular weight compounds are detected in biological tissues.25
Recently, a new MSI method called laser desorption VUV single photon postionization-mass spectrometry imaging (VUV-postionization MSI)26 was proposed, which has successfully realized in situ detection of the distribution of target molecules in biological tissues.27 Generally, the first laser is irradiated on the surface of the sample to vaporize the analytes, and the second laser horizontally crosses through the vaporized plume and ionizes them. The present technique, laser desorption postionization (LDPI), can greatly maximize the efficiency of ionization and the sensitivity by optimization of the wavelength and the photon density of the two lasers separately.28–30 Previously, polycyclic aromatic hydrocarbons (PAHs) on the Allende meteorite have been detected with a similar approach, which has ever been called two-step laser mass spectrometry.31 It is proved that this ionization method is suitable for the ionization of polycyclic aromatic hydrocarbons. The LDPI approach has also been used to detect exogenous drugs in animal tissues to identify the tumor area in the tissues.32 Compared with MALDI, LDPI technology does not require the sample pretreatment process by adding a matrix and avoids the background interference caused by the matrix; it can achieve direct, rapid and efficient localization and imaging of target molecules in animal and plant tissues.
In this work, we used VUV-postionization MSI to visualize the distribution of curcumin in the turmeric sections. Slices of the turmeric root at different maturity stages were imaged. By observing the distribution of curcumin at different maturity stages, the accumulation process of curcumin in turmeric root was revealed. Furthermore, the difference of the distribution of curcumin in the xylem and the phloem of root was compared to explore the transport mechanism of curcumin in turmeric root.
2. Materials and methods
2.1. Reagents, turmeric cultivation and tissue preparation
The standard curcumin (C21H20O6) was purchased from Sigma-Aldrich (St. Louis, MO, USA), and it can be used without further purification. Alcohol (C2H5OH), glutaraldehyde (C5H8O2), and Ultrapure deionized water (≥18 MΩ cm−2) was obtained from a Milli-Q ultrapure water system (Millipore, MA, USA). High-quality turmeric roots, obtained from the local market, were buried in nutritional soil, for quick germination. After one week, the turmeric sprouted. Another one week later, the germinated turmeric was transferred to organic soil for cultivation. After two months of cultivation, turmeric buds grew into stem leaves and new mature turmeric grew in the soil. The turmeric samples at the different maturity stages were chosen as the experimental samples.
In the experiment, turmeric sections with a thickness of 1 mm for the MSI experiment were manually cut using a blade and kept under dry air before being analyzed. A double-sided carbon tape was used to adhere the slice onto a sample plate which was mounted onto a three-dimensional linear positioner. The schematic of the procedures of the whole experiment is shown in Fig. 1.
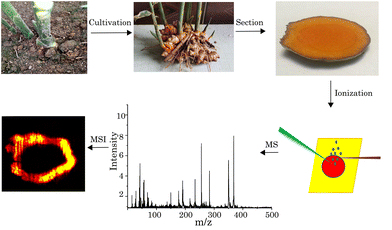 |
| Fig. 1 Schematic of the procedures of the whole experiment. | |
2.2. Apparatus
A schematic diagram of the experimental apparatus is shown in Fig. 2. The whole apparatus consists of a home-made time-of-flight mass spectrometer and a high vacuum chamber with a pressure of about 10−7 Torr equipped with a third harmonic generation (THG) cell. On the other side of the high vacuum chamber, a set of program-controlled three-dimensional platform was installed where the sampling rod can be transferred to the ionization region in the vacuum chamber.
 |
| Fig. 2 Schematic diagram of the VUV-postionization mass spectrometer imaging apparatus. | |
In this experiment, a 532 nm Nd:YAG laser (Minilite, Continuum Inc., San Jose, CA, USA) with a 10 Hz operational repetition rate was focused onto the sample surface for desorption. In the present setup, the pulse energy was controlled in the range of 200–500 μJ, which corresponded to laser fluences of approximately 20–60 MW cm−2. A vacuum ultraviolet (VUV 118 nm, 10.5 eV) light was used as the ionization source, which is generated by the second Nd:YAG laser (Beamtech Optronic Co., Ltd) with a fundamental wavelength of 1064 nm, operating at 10 Hz repetition rate. The 355 nm laser (∼20 mJ per pulse) output was obtained through a frequency tripling generator (FTG), which was focused into a cell filled with a high-purity nonlinear medium consisting of a Xe/Ar (v/v 1
:
10) gas mixture, wherein VUV (118 nm) radiation is thus generated.33,34 The pulse energy of the focused VUV light was not measured precisely, but it was estimated to be ca. 200 nJ based on an approximate conversion efficiency of 1.2 × 10−5 reported in the literature.35,36 The MgF2 lens with a focal length of 25 cm, placed between the gas cell and the high-vacuum chamber, was used to focus the VUV light to the detection area. In addition, the 355 nm laser that has not been transformed into the 118 nm laser will spread and not focus on the center of the ionization chamber.37 The VUV (118 nm) laser beam, which was parallel to the corundum sample plate, intercepted the desorption plume. The surface of the sample plate had inconsiderable topography in the Z direction to ensure the focal plane changed very slightly upon scanning the tissue sections.
3. Results and discussion
3.1. Direct analysis of standard curcumin and turmeric tissue
It is well-known that the ionization energy (IE) of most organic molecules is below 10 eV,38 while single photon energy at 118 nm is 10.5 eV. Therefore, the 118 nm laser can thus “soft” ionize most of the organic molecules. Additionally, we have estimated the ionization energy of the curcumin molecule theoretically before starting the experimental measurement. It is to confirm that the single VUV (118 nm) photon energy is higher than the IE of the curcumin molecule. Furthermore, we have used Gaussian 09 software (Gaussian Inc., Wallingford, CT, USA) to analyze the structure and configuration of curcumin molecules, and the theoretical calculation results showed that the IE of curcumin was ca. 6.5 eV. Hence, single photon ionization of the curcumin molecule by using 118 nm radiation is feasible, though the photo-fragmentation won't be avoided because of the excessive photon energy.
In the detection of mass spectra of standard curcumin, we evenly coated the curcumin on the sample stage, which was then transferred to the ionization chamber through the electric three-dimensional platform. The mass spectra of standard curcumin obtained by LDPI-MS are shown in Fig. 3 (green trace). The peak in the mass spectrum at m/z 368 corresponds to the molecule cations of curcumin compounds. Different from MALDI, we did not add any organic matrix, so there was no background interference peak in the low-mass region in the mass spectrum (as shown in Fig. 3 (green trace)). The other three relatively intense peaks at m/z 177, m/z 191 and m/z 252 could be derived from two fragments by the cleavage of the carbon chain between two benzene rings, respectively. In order to characterize the fragment peaks, tandem mass spectrometric (MS/MS) analysis of curcumin was conducted by using collision-induced dissociation (CID) with a collision energy of 10–40 eV and nitrogen as the collision gas. Ions were characterized using a high-resolution quadrupole/time-of-flight mass spectrometry (Q-TOF MS) system (Impact II, Bruker Daltonics Inc., Bremen, Germany). In the mass spectra, we also found a fragment peak at m/z 177 (as shown in Fig. S1, ESI†). Moreover, we conducted control experiments to confirm that these peaks came from the same precursor, i.e., curcumin molecule. We have also tried to analyze the sample with a single beam of laser (with the desorption laser at 532 nm or the ionization laser at 118 nm only) emitting on the curcumin sample. However, no mass spectrum signal was observed (red and black trace in Fig. 3). It is thus confirmed that the mass spectrum signal is the result of the synergistic action of the two laser beams.
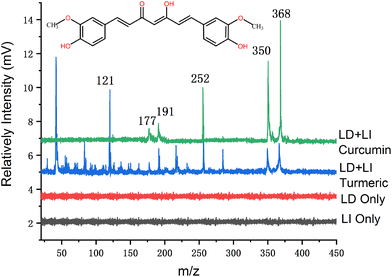 |
| Fig. 3 Mass spectra of the standard curcumin sample (green) and turmeric root slice (blue) obtained by laser desorption VUV-postionization mass spectrometry. The red trace and black trace were obtained by only desorption laser emitted and only VUV light emitted, respectively. The inset shows the chemical formula of curcumin. | |
For real sample analysis, a 1 mm-thickness circular thin slice was fixed on the sample table and transferred to the ionization region through the three-dimensional platform. The mass spectrum measured in the turmeric root section is shown in Fig. 3 (blue trace). More mass spectra peaks can be seen in the mass spectrum of the turmeric sample than that of the standard curcumin. The peaks related to the standard curcumin also appeared in the mass spectrum of turmeric (blue trace). Thus, it could be recognized that curcumin was detected in the turmeric root section. These mass spectra peaks with low mass weight could originate from other endogenous species of the turmeric. The intensity of the curcumin peak (m/z 368) in turmeric tissue is high enough, but three characteristic fragments (m/z 177, m/z 191 and m/z 252) can still be clearly identified in the mass spectrum, and there are no background peaks. In addition, the m/z 121 peak signal was strong in the turmeric tissue, but it did not appear in the curcumin standard samples, which can be assigned to other metabolites of turmeric. It indicates that the proposed method can be feasible to apply to the detection of organic ingredients in complex biological tissues.
3.2. Imaging of curcumin in tissue by VUV-postionization
The mass spectrum images were obtained by scanning the surface of the slices of the turmeric root with a program-controlled three-dimensional platform. Mass spectra were obtained point by point where the scanning step size was kept at 100 μm. Under the radiation of two laser pulses, the displacement interval of the sample stage is 100 μm. From the MSI image and the diameter of the laser spot, we can calculate that the lateral spatial resolution of our MSI experiments is 100 μm. (Fig. S2 shows the optical microscopy image of laser ablated craters, ESI,† which was obtained using a laser scanning microscope (LSM, model VHX-600 manufactured by KEYENCE Corporation, Tokyo, Japan).)
Fig. 4 shows the optical microscopy image and MSI images of a root cross-section, m/z 368 is the characteristic peak of curcumin, m/z 177 and m/z 191 are the fragment characteristic peaks of curcumin, and their imaging images are basically consistent with the real picture of turmeric slices. Turmeric organization m/z 121 mass spectrum peak intensity is high, but there was no corresponding signal peak in the mass spectrum of curcumin. In order to further explore the source of this peak, we imaged the m/z 121 signal peak, and the image obtained is highly similar to that of the characteristic peak of curcumin, so we speculate that m/z 121 is most likely a metabolite of curcumin.
 |
| Fig. 4 (a) Optical microscopy image of a root cross-section. MSI images of turmeric derived from different peaks, (b–d) are derived from the fragment cations of m/z 121, m/z 177 and m/z 191, and (e) is derived from the parent cations of curcumin at m/z 368. | |
3.3. Imaging of curcumin in tissue by VUV-postionization
As we know, the root of turmeric is composed of the outermost epidermis, the phloem, the cambium, the xylem, and the central pith. In terms of botanical functionality, the xylem and the phloem are the main transport systems in plant tissues. Xylem transport is unidirectional (upwards) and driven by the gradient in hydrostatic pressure along with water potential,39 in addition to supporting the plant body. The phloem locates between the epidermis and the cambium. The phloem consists of sieve molecules, sclerenchyma cells and parenchyma cells. As we know, phloem transport, another important translocation component, is multidirectional.40
The distribution of curcumin in the root showed different regularities in different maturity stages of turmeric. The root tissue images of turmeric in different periods are shown in Fig. 5. As the turmeric grows, the amount of curcumin in the middle region of the xylem accumulates slowly. In the immature turmeric root, a large amount of curcumin is found in the phloem near the epidermis, and very little of it accumulates in the xylem region. However, a certain amount of curcumin accumulates in the middle region of the xylem region as the turmeric grows. The distribution of curcumin in the rhizomes at different maturity stages is obviously different. In mature rhizomes, curcumin was mainly distributed in the phloem near the epidermis, and a small amount of curcumin was distributed in the middle region of the xylem. Compared with the rhizome of turmeric at the mature stage, curcumin in immature rhizomes is concentrated entirely in the phloem, while the xylem area has almost no curcumin. The distribution of curcumin showed a circular shape and basically matched the shape of the slice. Moreover, the amount of curcumin tends to decrease from the epidermis to the interior pith.
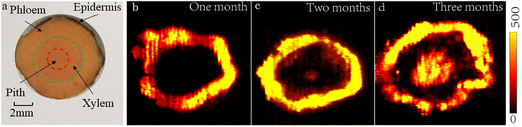 |
| Fig. 5 (a) Optical microscopy image of a root cross-section. MSI image of turmeric at different stages of maturity: (b) one month, (c) two months and (d) three months. | |
Generally, organic compounds in plants are transported from the phloem downward. So, mostly organic ingredients like curcumin should be accumulated in the middle of the pith of the root. Therefore, we hypothesized that curcumin in the pith of mature turmeric did not originate from the longitudinal transport of the plant, but probably accumulated slowly through the horizontal transport system of the plant. Plasmodesma is the channel for material and information transfer between cells. Therefore, organic compounds are transferred from one cell to another through plasmodesmata, and finally transported to the stele by means of protoplasm flow. Usually, ions and small organic molecules can pass through these channels. This transport manner could explain why the curcumin tends to accumulate in the central pith of the root. In addition, the xylem of plant tissue is made up of vessels and the cytoderm membranes between the adjacent cells make the ducts disappear. The xylem is thus shown as the hollow duct system. Because of this hollow system, organic ingredients would extend inward continually and eventually accumulate in the pith of the root. As can be seen from the measured images, the mature turmeric curcumin is mainly distributed in the epicuticle phloem near the epidermis and the pith of the root.
3.4 Quantitative analysis of curcumin in the turmeric
The results above indicate that qualitative analysis can be realized by the proposed method. The method of isotope labeling is better for quantitative analysis, but the cost of isotope labelling is expensive. We also considered the standard addition method, but the curcumin standard solution is very different from the turmeric. The distribution of curcumin in turmeric is uneven. In mature turmeric, curcumin is mainly distributed in the phloem and pith but not found in the xylem. Therefore, the standard addition method is not suitable for the detection of curcumin content in turmeric. Combined with our experimental techniques, the standard curve method may be more suitable. Hence, we explored the feasibility of the quantitative analysis of curcumin in the tissue. Firstly, we detected the detection of limit (LOD) for curcumin. Herein, a series of concentration gradients of pure curcumin solutions were prepared. 1 g standard curcumin was dissolved in 50 mL organic solvent to obtain 20 mg mL−1 standard solution. The standard curcumin solution was diluted multiple times for detection (5000 μg mL−1, 3000 μg mL−1, 2000 μg mL−1, 1000 μg mL−1, 500 μg mL−1, 200 μg mL−1, 100 μg mL−1, 50 μg mL−1, and 5.0 μg mL−1, respectively). In order to reduce the matrix-effect and tally with the biological environment of curcumin in the tissue of turmeric, we selected potato as an alternative, and sprayed curcumin solution evenly on the cross section. The mass spectrometric signal of different concentrations of standard solutions was measured, and the signal intensity of curcumin in tissue was compared to obtain the real concentration of turmeric. The results indicate that the signal of the lowest concentration of curcumin that we can detect is ca. 5.0 μg mL−1. In addition, the mass spectrometry signal intensity of curcumin solutions with different concentrations showed a good linear relationship in the concentration range of 5.0–5000 μg mL−1, and its corresponding correlation coefficient (r2) was 0.9978 (see Fig. 6).
 |
| Fig. 6 Calibration curve of curcumin standard solutions spotted on a plant tissue section using LDPI-MS. | |
Considering that the lowest concentration of curcumin signal that we can detect is 5.0 μg mL−1, the desorption amount of curcumin in each laser spot is estimated to be ca. 1.475 ng. The amount of molecules ionized by the laser is proportional to the area of the slice. The turmeric is cut into round slices with a diameter of 5 mm, and the surface area is about 19.63 mm2, whereas the diameter of the 532 nm laser spot was ca. 100 um. The turmeric section was scanned radially by the laser with 50 shots. The concentration corresponding to the intensity of each laser spot can be obtained from the standard curve in Fig. 6, and thus the average concentration of curcumin in ginger can be calculated. From the volume of turmeric, the content of curcumin in the whole section can be calculated. Finally, by weighing the ginger slices, the percentage content of curcumin in mature turmeric is estimated to be about 3.43%, which is consistent with the previous report that the content of curcumin in the turmeric is estimated between 3% and 5%.41 This result confirmed that the present VUV-postionization method can also have potential for application in the quantitative analysis of endogenous substances in plant tissues.
(
Mt: the amount of curcumin in the turmeric slices.
ML: the amount of curcumin ionized by a single laser.
St: the area of the turmeric slice.
SL: the area of the laser spot.
M: the area of the turmeric slice.)
4. Conclusion
We have achieved visualization imaging of endogenous compounds in the turmeric root by using LDPI-MSI with VUV as a postionization laser. The MSI images can clearly show the distribution of curcumin in different parts at different maturity stages. The results reveal that the distribution of curcumin in the turmeric root varied greatly at different maturation stages. When the turmeric is immature, curcumin is mainly distributed in the phloem near the outer epidermis of the root. When the turmeric is fully mature, however, curcumin was not only distributed in the phloem of the root, but also accumulated in the pith of the root.
Additionally, experimental results indicate that the proposed method can be not only used for the qualitative analysis of curcumin, but also conducted for the quantitative estimation of curcumin content in the turmeric root. The results revealed that the percentage content of curcumin in this variety of the mature turmeric is 3.43%. The present report indicates that VUV-postionization MSI can act as a promising method to be used for the quantitative analysis of the active ingredients and in situ detection of the distribution of ingredients in the plant. This technique has a great prospect in the study of plant pharmaceutical ingredient accumulation and metabolism pathway, and is likely to be widely used in the analysis of natural ingredients of plants.
Author contributions
Wuyi Nie: conceptualization, methodology, validation, investigation, data curation, and writing – original draft. Qiao Lu: writing – review & editing. Tao Hu: formal analysis, resources, and visualization. Min Xie: software. Yongjun Hu: supervision, project administration, and funding acquisition. All authors read and approved the final manuscript.
Conflicts of interest
There are no conflicts to declare.
Acknowledgements
We thank Dr Jun Zhou in South China Normal University for helpful discussions and suggestions on this paper. This work was supported by the National Natural Science Foundation of China (No. 22027808, 22073031 and 22003018) and Guangdong Basic and Applied Basic Research Foundation (2021A1515012344).
References
- R. R. Kotha and D. L. Luthria, Curcumin: Biological, Pharmaceutical, Nutraceutical, and Analytical Aspects, Molecules, 2019, 24, 2930 CrossRef PubMed.
- K. Fateme, S. Elnaz, M. R. Immaculada, Y. Ibraheem, M. H. Ghahremani and K. Sharmin, Infrared Microspectroscopy Studies On the Protective Effect of Curcumin Coated Gold Nanoparticles Against H2O2-Induced Oxidative Stress in Human Neuroblastoma SK-N-SH Cells, Analyst, 2021, 146, 902–6916 Search PubMed.
- M. Kharat, M. Skrzynski, E. A. Decker and D. J. McClements, Enhancement of Chemical Stability of Curcumin-Enriched Oil-In-Water Emulsions: Impact of Antioxidant Type and Concentration, Food Chem., 2020, 320, 126653 CrossRef CAS PubMed.
- E. V. Piletska, B. H. Abd, A. S. Krakowiak, A. Parmar, D. L. Pink, K. S. Wall, L. Wharton, E. Moczko, M. J. Whitcombe, K. Karim and S. A. Piletsky, Magnetic High Throughput Screening System for the Development of Nano-Sized Molecularly Imprinted Polymers for Controlled Delivery of Curcumin, Analyst, 2015, 140, 3113–3120 RSC.
- O. V. Dement'eva, K. A. Naumova, E. M. Shishmakova, I. N. Senchikhin, S. K. Zhigletsova, M. V. Klykova, I. A. Dunaitsev, D. A. Kozlov and V. M. Rudoy, Synthesis of Bifunctional Silica Container Particles on Antiseptic Micelles with Solubilized Curcumin and Assessment of Their Biological Activity, Colloid J., 2022, 83, 651–661 CrossRef.
- T. Benameur, R. Soleti, M. A. Panaro, M. E. La Torre, V. Monda, G. Messina and C. Porro, Curcumin as Prospective Anti-Aging Natural Compound: Focus on Brain, Molecules, 2021, 26, 4794 CrossRef CAS.
- N. Geline, I. R. Suhito, C. H. Kim, G. P. Hong, C. G. Park, S. H. Bhang, Z. T. Luo and T. H. Kim, A Fibronectin-Coated Gold Nanostructure Composite for Electrochemical Detection of Effects of Curcumin-Carrying Nanoliposomes on Human Stomach Cancer Cells, Analyst, 2020, 145, 675–684 RSC.
- Y. R. Jin, M. J. Oh, H. J. Yuk, H. J. An and D. S. Kim, Novel Analysis Procedure for Red Ginseng Polysaccharides by Matrix-Assisted Laser Desorption/ionization Time-of-Flight/Time-of-Flight Mass Spectrometry, J. Ginseng Res., 2021, 45, 539–545 CrossRef PubMed.
- C. Bortolini, A. Pivato, S. Bogialli and P. Pastore, “One-Shot” Analysis of PDE-5 Inhibitors and Analogues in Counterfeit Herbal Natural Products Using an LC-DAD-QTOF System, Anal. Bioanal. Chem., 2015, 407, 6207–6216 CrossRef CAS.
- B. Han, J. Vial, S. Sakamoto and M. Sablier, Identification of Traditional East Asian Handmade Papers Through the Multivariate Data Analysis of Pyrolysis-GC/MS Data, Analyst, 2019, 144, 1230–1244 RSC.
- D. S. Su, Y. Y. Zheng, Z. Q. Chen and Y. W. Chi, Simultaneous Determination of Six Glycosidic Aroma Precursors in Pomelo by Ultra-High Performance Liquid Chromatography-Tandem Mass Spectrometry, Analyst, 2021, 146, 1698–1704 RSC.
- L. P. Zhan, X. Huang, J. J. Xue, H. H. Liu, C. Q. Xiong, J. Y. Wang and Z. X. Nie, MALDI-TOF/TOF Tandem Mass Spectrometry Imaging Reveals Non-Uniform Distribution of Disaccharide Isomers in Plant Tissues, Food Chem., 2020, 338, 127984 CrossRef PubMed.
- S. L. Li, N. L. Zhu, C. P. Tang, H. N. Duan, Y. W. Wang, G. R. Zhao, J. Liu and Y. Ye, Differential Distribution of Characteristic Constituents in Root, Stem and Leaf Tissues of Salvia Miltiorrhiza Using MALDI Mass Spectrometry Imaging, Fitoterapia, 2020, 146, 104679 CrossRef CAS PubMed.
- X. W. Song, Q. C. Zang and R. N. Zare, Hydrogen-Deuterium Exchange Desorption Electrospray Ionization Mass Spectrometry Visualizes an Acidic Tumor Microenvironment, Anal. Chem., 2021, 93, 10411–10417 CrossRef CAS PubMed.
- Y. Y. Liu, X. X. Ma, Z. Q. Lin, M. J. He, G. J. Han, C. D. Yang, Z. Xing, S. C. Zhang and X. R. Zhang, Imaging Mass Spectrometry with a Low-Temperature Plasma Probe for the Analysis of Works of Art, Angew. Chem., Int. Ed., 2010, 49, 4435–4437 CrossRef.
- Q. Lu, Z. Y. Xu, X. You, S. Y. Ma and R. Zenobi, Atmospheric Pressure Mass Spectrometry Imaging Using Laser Ablation, Followed by Dielectric Barrier Discharge Ionization, Anal. Chem., 2021, 93, 6232–6238 CrossRef PubMed.
- S. A. Stopka, E. A. Wood, R. Khattar, B. J. Agtuca, W. M. Abdelmoula, N. Y. R. Agar, G. Stacey and A. Vertes, High-Throughput Analysis of Tissue-Embedded Single Cells by Mass Spectrometry with Bimodal Imaging and Object Recognition, Anal. Chem., 2021, 93, 9677–9687 CrossRef PubMed.
-
L. Z. Samarah, T. H. Tran, G. Stacey and A. Vertes, Mass Spectrometry Imaging of Bio-oligomer Polydispersity in Plant Tissues by Laser Desorption Ionization from Silicon NanopostAngew. Chem., Int. Ed. 2021609071–9077 Search PubMed.
- X. Huang, L. P. Zhan, L. Sun, J. J. Xue, H. H. Liu, C. Q. Xiong and Z. X. Nie, Utilizing a Mini-Humidifier to Deposit Matrix for MALDI Imaging, Anal. Chem., 2018, 90, 8309–8313 CrossRef PubMed.
- B. B. Liu, X. X. Wang, K. Li and Z. W. Cai, Spatially Resolved Metabolomics and Lipidomics Reveal Salinity and Drought-Tolerant Mechanisms of Cottonseeds, J. Agric. Food Chem., 2021, 69, 8028–8037 CrossRef PubMed.
- T. Zeng, R. Zhang, Y. Y. Chen, W. J. Guo, J. N. Wang and Z. W. Cai, In Situ Localization of Lipids on Mouse Kidney Tissues with Acute Cadmium Toxicity Using Atmospheric Pressure-MALDI Mass Spectrometry Imaging, Talanta, 2022, 245, 123466 CrossRef.
- S. Mohammadi and H. Parastar, Quantitative Analysis of Multiple High-Resolution Mass Spectrometry Images Using Chemometric Methods: Quantitation of Chlordecone in Mouse Liver, Analyst, 2018, 143, 2416–2425 RSC.
- T. Wang, H. K. Lee, G. G. L. Yue, A. C. K. Chung, C. B. S. Lau and Z. W. Cai, A Novel Binary Matrix Consisting of Graphene Oxide and Caffeic Acid for the Analysis of Scutellarin and Its Metabolites in Mouse Kidney by MALDI Imaging, Analyst, 2021, 146, 298–295 Search PubMed.
- R. Y. Sun, Y. Zhang, W. W. Tang and B. Li, Submicron 3,4-Dihydroxybenzoic Acid-TiO2 Composite Particles for Enhanced MALDI MS Imaging of Secondary Metabolites in the Root of Differently Aged Baical Skullcap, Analyst, 2022, 147, 3017–3024 RSC.
- D. S. Peterson, Matrix-Free Methods for Laser Desorption/Ionization Mass Spectrometry, Mass Spectrom. Rev., 2006, 26, 19–34 CrossRef.
- G. L. Gasper, R. Carlson, A. Akhmetov, J. F. Moore and L. Hanley, Laser Desorption 7.87 eV Postionization Mass Spectrometry of Antibiotics in Staphylococcus Epidermidis Bacterial Biofilms, Proteomics, 2008, 8, 3816–3821 CrossRef PubMed.
- Y. Cui, C. Bhardwaj, S. Milasinovic, R. P. Carlson, R. J. Gordon and L. Hanley, Molecular Imaging and Depth Profiling of Biomaterials Interfaces by Femtosecond Laser Desorption Postionization Mass Spectrometry, ACS Appl. Mater. Interfaces, 2013, 5, 9269–9275 CrossRef PubMed.
- A. E. Pomerantz, M. R. Hammond, A. L. Morrow, O. C. Mullins and R. N. Zare, Two-Step Laser Mass Spectrometry of Asphaltenes, J. Am. Chem. Soc., 2008, 130, 7216–7217 CrossRef.
- J. Soltwisch, H. Kettling, S. Vens-Cappell, M. Wiegelmann, J. Muthing and K. Dreisewerd, Mass Spectrometry Imaging with Laser-Induced Postionization, Science, 2015, 348, 211–215 CrossRef PubMed.
- Q. H. Wu and R. N. Zare, Laser Desorption Lamp Ionization Source for Ion Trap Mass Spectrometry, J. Mass Spectrom., 2015, 50, 160–164 CrossRef.
- R. Zenobi, J. M. Philippoz, R. N. Zare and P. R. Buseck, Spatially Resolved Organic Analysis of the Allende Meteorite, Science, 1989, 246, 1026–1029 CrossRef.
- Q. Lu, Y. J. Hu, J. X. Chen and S. Jin, Laser Desorption Postionization Mass Spectrometry Imaging of Folic Acid Molecules in Tumor Tissue, Anal. Chem., 2017, 89, 8238–8243 CrossRef PubMed.
- J. L. Trevor, K. R. Lykke, M. J. Pellin and L. Hanley, Two-Laser Mass Spectrometry of Thiolate, Disulfide, and Sulfide Self-Assembled Monolayers, Langmuir, 1998, 14, 1664–1673 CrossRef.
- S. Milasinovic, Y. Liu, C. Bhardwaj, M. T. M. Blaze, R. J. Gordon and L. Hanley, Feasibility of Depth Profiling of Animal Tissue by Ultrashort Pulse Laser Ablation, Anal. Chem., 2012, 84, 3945–3951 CrossRef.
- Y. Hu, J. Guan and E. R. Bernstein, Mass-Selected IR-VUV (118 nm) Spectroscopic Studies of Radicals, Aliphatic Molecules, and Their Clusters, Mass Spectrom. Rev., 2013, 32, 484–501 CrossRef PubMed.
- A. H. Kung, Third-Harmonic Generation in a Pulsed Supersonic Jet of Xenon, Opt. Lett., 1983, 8, 24–26 CrossRef PubMed.
- J. M. Gray, J. Bossert, Y. Shyur, B. Saarel, T. C. Briles and H. J. Lewandowski, Characterization of a Vacuum Ultraviolet Light Source at 118 nm, J. Chem. Phys., 2020, 154, 024201 CrossRef.
- L. Hanley and R. Zimmermann, Light and Molecular Ions: The Emergence of Vacuum UV Single-Photon Ionization in MS, Anal. Chem., 2009, 81, 4174–4182 CrossRef PubMed.
- H. K. Kim, J. Park and I. Hwang, Investigating Water Transport Through the Xylem Network in Vascular Plants, J. Exp. Bot., 2014, 65, 1895–1904 CrossRef.
- S. Endo, Y. Iwai and H. Fukuda, Cargo-Dependent and Cell Wall-Associated Xylem Transport in Arabidopsis, New Phytol., 2019, 222, 159–170 CrossRef PubMed.
- R. C. Alves, R. P. Fernandes, B. Fonseca-Santos, F. D. Victorelli and M. Chorilli, A Critical Review of the Properties and Analytical Methods for the Determination of Curcumin in Biological and Pharmaceutical Matrices, Crit. Rev. Anal. Chem., 2018, 49, 138–149 CrossRef.
|
This journal is © The Royal Society of Chemistry 2023 |
Click here to see how this site uses Cookies. View our privacy policy here.