DOI:
10.1039/D2AN01374F
(Paper)
Analyst, 2023,
148, 163-174
HClO-triggered interventional probe enabled early detection and intervention of atherosclerosis
Received
20th August 2022
, Accepted 16th November 2022
First published on 23rd November 2022
Abstract
Foam cell formation and further accumulation in the subendothelial space of the vascular wall is a hallmark of early atherosclerosis (AS). Targeting foam cell formation can be a promising approach for the early detection and prevention of AS. However, only a few studies have actually examined foam cells in vivo, and most methods combined nanotechnology with angiography, which is complex and could cause further damage to the endothelium. Herein, based on methylene blue, a biosafe NIR dye approved by the FDA, an interventional probe (HMB-NA@Mp) triggered by hypochlorous acid (HClO) was designed for imaging foam cells easily, safely, and effectively in the early stage of AS. Here, encapsulation of the probe by foam cells targeted platelet membrane (Mp) increased probe targeting and reduced toxicity. Cell and animal experimental results showed that the probe could accumulate at the lesion site and significantly enhance fluorescence in the early AS model group. Remarkably, at the same time, it could also release the metabolite niacin, which played a role in inhibiting atherosclerosis. Thus, HMB-NA@Mp is expected to be a powerful means for the early detection and timely intervention of early AS in the absence of clinical symptoms.
1 Introduction
Atherosclerosis (AS) is the main culprit of a variety of cardiovascular diseases, such as coronary heart disease and myocardial infarction.1,2 A major obstacle to overcome AS is that it has hidden pathogenesis and develops without any symptoms until a traumatic event or sudden death is caused by severe vessel occlusion or plaque rupture. Therefore, it is crucial to take effective measures for the early detection and intervention of AS. As can be seen from the pathogenesis of AS,3–5 the formation of foam cells, as an early event of AS,6,7 plays an important role in the occurrence and all stages of AS.8,9 If the early detection and effective intervention of AS can be carried out in the foam cell stage, it is expected to inhibit or even reverse the progression of AS.5,10–12 At present, some methods for foam cell detection have been reported and most of them combine nanotechnology and angiography to diagnose early AS. For example, Zhang et al. used profilin-1 to detect vascular smooth muscle cells and foam cells in fibrous caps by combining targeted contrast agents with MRI imaging technology,13 while Nicol et al. detected foam cells in tissues by OTC evaluation of the tissue attenuation index of the stent artery.14 However, there are still some shortcomings. First, these methods often require expensive equipment and complex operations. Second, in the detection process, the contrast agent or synthetic nanoparticles may be regarded as foreign bodies by the body, triggering an immune response, aggravating the inflammatory state and possibly causing further damage to the vascular endothelium. Besides, some other studies on foam cell detection are mostly limited to the in vitro cell level,15–17 and in vivo studies are relatively scarce. Therefore, there is an urgent need to find a simple, effective, and highly biosafe method for the early detection of AS in the foam cell stage.
It is known that the formation of foam cells is closely related to the oxidative stress process. Studies have shown that the reactive oxygen species (ROS) in early foam cells is significantly higher than in macrophages and other cells; in addition, foam cells will continue to produce a large number of ROS after stimulation with ox-LDL,18 which may further lead to the difference in ROS levels between foam cells and other cells. Hypochlorous acid (HOCl), as a kind of ROS, can participate in REDOX homeostasis in the body, and its abnormal production is associated with a variety of inflammatory diseases, such as AS.19,20 On the basis of the differences in intracellular HClO content between foam cells and macrophages reported in the literature,21 it is considered that intracellular HClO can be used as a target for the detection of foam cells. Therefore, there is a vital need to develop a method for the detection of HClO in foam cells in situ.
In recent years, small molecular fluorescent probes have become a powerful detection technique for intracellular ROS due to their noninvasiveness, high sensitivity, and superior resolution capability.22–25 However, there are still challenges to overcome to achieve the in situ detection of HClO in vivo. First, because tissue penetration and the self-fluorescent background of the probe must be considered for their biological applications, there is still room for improvement in its fluorescence emission wavelength compared with our previous research.26 Second, due to the complex biochemical reactions under physiological conditions, HOCl only exists for a short time. It is reported that the diffusion distance of HOCl is less than 20 μm,19,27 which means that the probe must be able to respond quickly to HOCl.
According to reports, near-infrared (NIR) fluorescent probes that operate in the excitation and emission wavelengths in the 650–900 nm region are attractive for fluorescent bioimaging because of the deep tissue penetration28–30 and low auto-background fluorescence.31 Methylene blue (MB), a biosafe NIR dye approved by the US FDA, which has strong absorption in the NIR region,32 and its reduced form (leucomethylene blue, LMB) are non-fluorescent and have absorptions only in the ultraviolet region.33 In addition, LMB or its derivatives can respond specifically to HClO through deformylation reactions reported in the literature,34 which can generate intense absorption changes with concomitant NIR emission, which can perfectly meet the design conditions of a methylene blue NIR “off–on” fluorescent probe. Therefore, MB can be selected as the fluorophore framework to design a NIR fluorescent probe for detecting HClO in foam cells.
In order to increase the accumulation capacity of the probe in the disease site and improve the diagnostic accuracy, the platelet membrane (Mp) was chosen for wrapping the probe in this study. Studies have found that platelet membranes can target AS sites and have a strong affinity with foam cells,35 whereby they can recognize foam cells but will not be swallowed by macrophages,35,36 and consequently have been used for drug delivery and the targeted therapy of AS.26,37,38 Moreover, they also can reduce the toxicity of the fluorescent probe. These characteristics can improve the accumulation of internal probes in foam cells and improve the detection accuracy.
Combining the above ideas, a NIR fluorescent probe, HMB-NA, with an intervention function was designed successfully with MB as the fluorophore framework and modified with nicotinic acid (NA), a lipid regulating drug with anti-AS effects. Then, a bionic fluorescence probe (HMB-NA@Mp) with high biosafety was prepared by wrapping the natural platelet membrane (Mp) on the probe surface with a liposome extruder. In the fluorescent probe, the toxicity of HMB-NA was reduced and the affinity of HMB-NA@Mp for AS vascular was improved because of Mp. After reaching the lesion site, the HClO-triggered NIR probe could be selectively taken up by foam cells. In foam cells, HMB-NA generated strong NIR fluorescence because of its rapid response to HClO through specific deacylation.34,39 Significantly, it could also release metabolites NA at the same time, which could improve endothelial disorders and inhibit the formation of foam cells.40–45 The in vivo fluorescence images showed that the fluorescent signal generated by the probe could be observed in the thoracic aorta in early AS rats. Also, pharmacodynamic experiments also indicated that it had an intervention effect on the regulation of blood lipids in AS rats. Hence, the HMB-NA@Mp designed in this study can achieve the goals of “early detection, early intervention” and also provides a theoretical basis for the early detection and intervention of other oxidative stress diseases (Fig. 1).
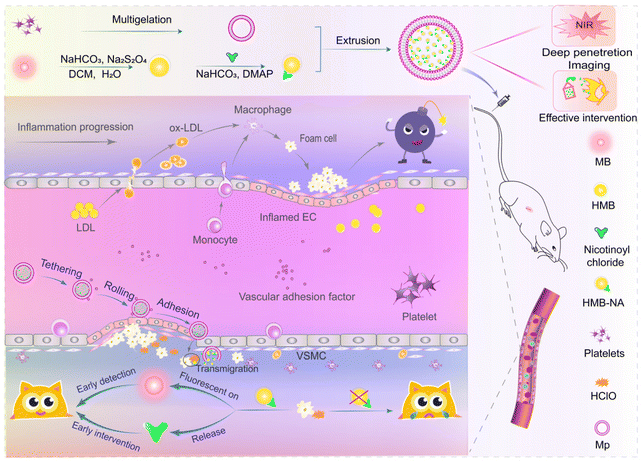 |
| Fig. 1 Schematic diagram of the HClO-triggered interventional probe HMB-NA@Mp in vivo. | |
2 Experimental section
2.1 Materials
Methylene blue (MB) and sodium hydrosulfite (Na2S2O4) were purchased from Shanghai Jiuding Chemical Technology Co., Ltd. Prostaglandin EI (PGEI) and 4,6-diamidino-2-phenylindole (DAPI) were obtained from American Sigma Co. Phenylmethanesulfonyl fluoride (PMSF) was bought from Beijing Leagene Biotechnology Co., Ltd. Nicotinyl chloride hydrochloride was provided by Shanghai Jizhi Biochemical Technology Co., Ltd. RPMI 1640 cell culture medium, DMEM cell culture medium, and fetal bovine serum (FBS) were obtained from Beyotime Biotechnology Co., Ltd. 3-(4,5-Dimethyl-2-thiazolyl)-2,5-diphenyl-2-H-tetrazolium bromide (MTT) was provided by Biotopped. All the reagents were of analytical purity and used without further purification.
2.2 Synthesis of the HMB-NA probe
HMB-NA was synthesized on the basis of a previous study.39 MB (319.5 mg, 1 mmol), Na2S2O4 (700.1 mg, 4 mmol), and NaHCO3 (336.0 mg, 4 mmol) were placed in a 25 mL round-bottom flask, which was fitted with a magnetic stirrer and was evacuated and backfilled three times with N2. Then, 5 mL dichloromethane and 7.5 mL water were added into the flask under N2. The solution was refluxed for 30 min at 40 °C until the solution became yellow. The organic layer was separated from the water layer and dried with anhydrous sodium sulfate quickly, and then the dried organic phases were quickly poured into a mixture of DMAP (122.6 mg, 1 mmol) and NaHCO3 (336.1 mg, 4 mmol) in 5 mL dichloromethane under N2. Then, the nicotinoyl chloride solution (213.6 mg, 1.2 mmol) was added dropwise and stirred slowly in an ice water bath. After the addition, the mixture was stirred at room temperature until the reaction was completed, as indicated by TLC analysis.
After the reaction was completed, the solution was poured into 200 mL of ice water and extracted with 3 × 100 mL of ethyl acetate. Then the combined extracts were washed with brine, dried over anhydrous sodium sulfate, and evaporated on a rotary evaporator, and finally, the concentrate was purified by column chromatography (ethyl acetate/petroleum ether = 1/2, v
:
v) to yield HMB-NA (67.3 mg, 17.2%) as a white solid.
2.3 Extraction of the platelet membrane (Mp)
Mp was extracted according to the method previously reported.26 Male SD rats were anesthetized by the injection of 10% chloral hydrate (1.0 mL per 200 g) through the tail vein, and blood was collected from the abdominal aorta. Then, fresh whole blood was immediately centrifuged at room temperature for 20 min at 2000 rpm to obtain platelet-rich plasma (PRP), and the PRP supernatant was centrifuged 3 times (2000 rpm, 20 min) to remove residual RBC. The obtained supernatant was dispersed into PBS buffer containing 1 mM EDTA solution and 2 μM PGE1 to inhibit platelet activation, and then centrifuged at 3000 rpm for 20 min. Next, the platelet precipitate was resuspended in PBS buffer containing 1 mM EDTA and 1 mM PMSF. After freeze–thaw treatment was repeated 3 times, ultrasonic treatment was performed for 5 min (42 kHz, 100 W) to break the platelet membrane. After that, the solution was centrifuged at 8000 rpm for 5 min and the precipitate was washed with PBS buffer containing 1 mM PMSF 3 times to collect the platelet membrane precipitates.
2.4 Synthesis of HMB-NA@Mp
The reserve liquid of the HMB-NA fluorescent probe was prepared with DMSO (1 mM), and then 10 μm solutions were prepared in PBS buffer for subsequent experiments and spectral measurement. At the same time, 0.5 mL PBS solution was added into the 5 mL centrifuge tube to re-suspend the platelet membrane precipitation, and then 0.5 mL 10 μM HMB-NA solution was added. After homogenization, the obtained solution was extruded successively through 400 nm and 200 nm polycarbonate films on the liposome extruder 40 times, and the obtained solution was HMB-NA@Mp.
2.5 Characterization and spectral measurements of HMB-NA and HMB-NA@Mp
DLS, TEM, UV–Vis spectra, and fluorescence analyses, and the encapsulation rate were used to characterize HMB-NA and HMB-NA@Mp. Dynamic light scattering laser nanoparticle size analysis (DLS, Nano ZS90, Malvern, UK) was used to measure the average sizes and potentials of HMB-NA and HMB-NA@Mp at 25 °C. The morphology of HMB-NA@Mp was observed by transmission electron microscopy (TEM, JEM-1230, Japan). A Nicolet iS10 spectrometer (Thermo) was used to obtain the Fourier transform infrared (FT-IR) spectra of HMB-NA. The absorbance spectra of HMB-NA and HMB-NA@Mp in the presence and absence of HClO were analyzed using an UV–vis spectrophotometer (Shimadzu, Japan). The fluorescence spectra, pH stability, and response time to HClO of HMB-NA and HMB-NA@Mp were measured by a fluorescence spectrometer (LFS920, Japan).
2.6 Cell culture and cytotoxicity assay on RAW 264.7, HUVEC, and foam cells
In this study, RAW 264.7 cells (from Shanghai Cell Bank of the Chinese Academy of Sciences) were treated with different concentrations of ox-LDL to form foam cells. RAW 264.7 cells were seeded in a 6-well culture plate at a density of 2.5 × 105 cells per well, and then 0, 60, or 80 μg mL−1 of ox-LDL was added to each well successively and incubated for 24 h. After 24 h, the cells were stained with oil red O and the formation of foam cells was observed under a laser scanning confocal microscope (Zeiss LSM 880).
The cytotoxicity of HMB-NA in RAW 264.7 cells, human umbilical vein endothelial cells (HUVEC, from Shanghai Cell Bank of the Chinese Academy of Sciences), and foam cells were tested by MTT assay. The RAW 264.7, HUVEC, and foam cells were seeded in a 96-well plate at a density of 8000 cells per well and cultured in 5% CO2 at 37 °C. After cell attachment for 24 h, these cells were incubated with various concentrations of HMB-NA and HMB-NA@Mp (0, 10, 20, 40, 80, 100, 120, 160, and 200 μg mL−1) for 24 h or 48 h. Afterward, the medium was replaced with 20 μL of MTT solution (5 mg mL−1) per well and the cells were coincubated for another 4 h. Thereafter, 150 μL DMSO was added to each well of the 96-well plate to dissolve the purple formazan after elimination of the supernatants. Then a microplate reader (ELISA of PerkinElmer) was used to measure the absorbance of each well at 490 nm.
2.7 Fluorescence imaging and the uptake of foam cells
In order to determine the cellular uptake of the probe by the foam cells and RAW 264.7 cells, RAW 264.7 and foam cells were seeded in 6-well plates at a density of 2.5 × 105 cells per well and cultured for 24 h. Then, HMB-NA or HMB-NA@Mp was added to the medium. After culturing for 1, 2, and 4 h, respectively, the cells were washed 3 times with PBS, and then trypsinized and resuspended in PBS for flow cytometry analysis (Cytomics™ FC 500, Beckman Coulter, Miami, FL, USA).
In order to evaluate the imaging ability of the probe for foam cells, RAW 264.7 and foam cells were seeded in 6-well plates at a density of 2.5 × 105 cells per well. After 24 h, the fresh medium with different probes (HMB-NA, HMB-NA@Mp) were replaced. The cells were washed 3 times with PBS after 1, 2, and 4 h. The cell nucleus was stained with DAPI (10 μg mL−1) for 15 min. After removing excessive DAPI and washing again, 2 mL fresh medium was added and the cells were imaged by a laser scanning confocal microscope (Zeiss LSM 880).
2.8 Construction of the early AS model
All the animal procedures were performed in accordance with the Guidelines for Care and Use of Laboratory Animals and were approved by the Animal Ethics Committee of Zhengzhou University.
According to the Guidelines for The Care and Use of Experimental Animals, we started the animal experiments only after the approval of the Animal Ethics Committee of Zhengzhou University. We constructed a rat model of early AS by combining a high-fat diet with the intraperitoneal injection of vitamin D3 as per our previous report.26 In the second week, fourth week, and sixth week after modeling, the rats in the model and control group were randomly selected for the detection of four indexes of the blood lipids, and the thoracic aorta (including the aortic arch part) was dissected and removed for hematoxylin–eosin (H&E) staining. Then, the model rats were compared with the control rats to judge whether the model had been successfully constructed.
2.9 Fluorescence imaging in the early AS SD rats
After successful modeling of early AS, the control and model group rats were injected with the foam cell-targeted interventional probe and the corresponding free probe of 2 mg kg−1 through the tail vein. Then, imaging experiments were performed successively at 1, 2, 4, 8, 12, and 24 h after injection. Fluorescence differences between the control and model rats were observed in a small animal live imaging system and the images were analyzed using a Bruker MI SE system.
2.10 Biosafety of HMB-NA@Mp
The remaining rats in each group were respectively injected with the interventional probe HMB-NA@Mp (2 mg kg−1) through the tail vein, respectively. After 24 h, the main organs (including heart, liver, spleen, lung, kidney, and thoracic aorta) were dissected and H&E stained to evaluate their acute toxicity, in order to investigate the biological safety of the prepared interventionable fluorescent probe. Then we observed the morphological changes under a microscope (Zeiss LSM 510).
2.11 Investigation of the intervention effect of HMB-NA@Mp
After the successful modeling of early AS, the model rats were randomly divided into an intervention group and non-intervention group. The intervention group was injected with HMB-NA@Mp (2 mg kg−1 100 μg mL−1) every 2 days through the tail vein, and this was replaced by normal saline in the non-intervention group. Two weeks later, the main organs (including heart, liver, spleen, lung, kidney, and thoracic aorta) of the rats in each group were dissected and H&E staining was performed. Morphological changes were observed under a Zeiss LSM 510 microscope. The blood lipid indicators of the rats in each group were detected and the thoracic aorta was dissected for oil red O staining to investigate the intervention effect of the interventional probe on AS.
3 Results and discussion
3.1 Design and optical properties of HMB-NA
The new HOCl-triggered probe HMB-NA was synthesized by incorporating an anti-atherosclerosis part (NA) into the fluorophore framework MB with nicotinoyl chloride (Fig. 2A). The addition of NA with MB via a successive reduction and amide reaction46 was proposed to destroy the conjugation system of MB, leading to a complete annihilation of its absorption and fluorescence. In the presence of HOCl, the tertiary amide portion of HMB-NA underwent HOCl-mediated deacylation to release NA and MB. The successful synthesis of HMB-NA was characterized by infrared spectroscopy (Fig. 2B). The infrared spectrum of HMB-NA showed that the skeleton vibration peak of the benzene ring appeared at about 1604 and 1488 cm−1. In addition, compared with the MB, the probe produced new absorption peaks at 1648 and 1567 cm−1, corresponding to the –C
O stretching vibration peak of the tertiary amide compound, while the absorption peak at 1260 cm−1 corresponded to the stretching vibration peak of –CN, suggesting that HMB-NA included the formation of a tertiary amide, which could verify the successful synthesis of HMB-NA.
 |
| Fig. 2 (A) Schematic diagram of the HMB-NA probe's synthetic route and response to HClO. (B) Absorption spectra of HMB-NA (10 μM) before/after the addition of different concentrations of HOCl (0, 0.5, 1.0, 1.5, 2.5, 5, 10, and 20 μM). (C) Fluorescent spectra of HMB-NA (10 μM) before/after the addition of different concentrations of HOCl (0, 0.5, 1.0, 1.5, 2.5, 5, 10, and 20 μM). (D) Infrared spectra of MB and HMB-NA. | |
We evaluated the fluorescence response of HMB-NA to HOCl under simulated physiological conditions (PBS buffer, pH 7.4). As expected, it could be clearly seen that the UV/vis and fluorescence spectra of HMB-NA (10 μM) displayed almost no absorption and emission in the near-infrared wavelength region (Fig. 2C and D) in the absence of HOCl. In contrast, upon treatment with different concentrations of HOCl, the fluorescence emission intensity of HMB-NA at 683 nm and the absorbance at 652 nm were dramatically increased, together with a change of the solution color from colorless to blue, suggesting that HMB-NA can serve as a potential indicator for the “naked eye” detection of HOCl. In addition, the fluorescence emission and the absorbance intensity increased as the HOCl concentration increased. These changes were well consistent with the optical characteristics of MB, which implied the formation of MB. In fact, in addition to the generated MB, the reaction of HMB-NA with HOCl also generated the AS intervention group, NA, which was confirmed by HPLC (Fig. 3C).
 |
| Fig. 3 (A) Fluorescence enhancement time profiles of HMB-NA (10 μM) with the addition of HOCl in PBS buffer (pH 7.4). The excitation and emission wavelength (λex/λem) were 620/683 for HMB-NA. (B) Changes in HMB-NA's fluorescence intensity with pH before/after the addition of HClO. (C) HPLC analysis of HMB-NA after reaction with HOCl: (a) 10 μM HMB-NA + 15 μM HOCl, and (b) 5 μM nicotinic acid and 2 μM methylene blue. | |
Due to the high reactivity of HOCl to biomolecules in vivo, its existence time in vivo is very short, which puts forward a high requirement for the reaction speed of HMB-NA. Therefore, we measured the ability of the rapid fluorescence response of HMB-NA toward HOCl. We can see from Fig. 3A that the fluorescence intensity of the probe was very weak and remained unchanged before the addition of HClO. However, after the addition of HOCl at 25 s, the fluorescence intensity increased sharply, reaching the maximum value within 5 s and then keeping stable. The results demonstrated that the fluorescence response speed between HMB-NA and HOCl was very fast and the reaction products MB were stable in PBS buffer, indicating the feasibility for the rapid detection of HOCl in vivo.
It is necessary for the probe to keep a stable structure and have a stable response to the marker under the pH condition found in vivo. Therefore, the pH stability of the HMB-NA probe was determined by fluorescence spectrophotometry. We can see from Fig. 3B that in the absence of HClO, the fluorescence intensity of HMB-NA in each pH solution was very weak and there was no significant difference among them, which indicated that the structure of the probe could remain stable at pH 3–11. However, in the presence of HClO, the fluorescence intensity of the probe at 683 nm was significantly enhanced. By comparing the fluorescence intensity under different pH conditions, it could be found that when the pH was in the range of 4–8, the fluorescence response of HMB-NA to HClO was strong and stable; while the pH under a normal physiological environment was about 7.4, which indicated that the probe could successfully detect HClO under the pH condition in vivo.
3.2 Characterization and the optical properties of HMB-NA@Mp
In order to increase the enrichment of the probes in the disease site and improve the accuracy of detection, we extruded the Mp and HMB-NA through a carbonate membrane to synthetize the interventional probe HMB-NA@Mp. The synthetic route of HMB-NA@Mp is shown in Fig. 4A. We first compared the particle sizes and the potential of Mp with HMB-NA@MP by dynamic light scattering (DLS). As shown in Fig. 4B, coating with the Mp vesicles increased the mean sizes from 78.8 nm (Mp) to 164.2 nm (HMB-NA@Mp). In addition, Mp had a zeta potential of −12.2 mV, whereas HMB-NA@Mp (−18.4 mV) had similar values (Fig. 4B). Next, we measured the morphologies and sizes of Mp and HMB-NA@Mp by TEM, which showed that they were both spherical and evenly distributed and their sizes were slightly smaller than that measured by DLS (Fig. 4C). Collectively, these results showed that there were very little changes in the size, potential, and morphology before and after the TMB-NA was co-extruded with Mp, which indicated that the biological function of Mp had also been well preserved.
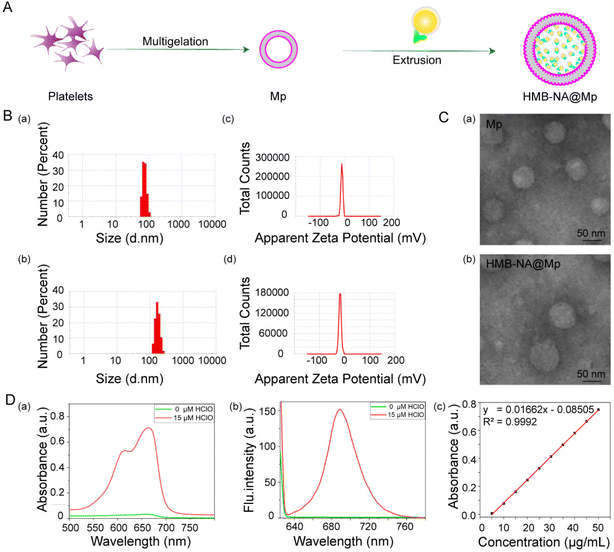 |
| Fig. 4 (A) Preparation process of HMB-NA@Mp. (B) (a) Particle size and (b) potential of the blank Mp; (c) particle size and (d) potential of HMB-NA@Mp. (C) TEM images of (a) blank Mp, (b) HMB-NA@Mp. (D) (a) Absorption spectra of HMB-NA@Mp (10 μM) before/after the addition of HOCl (15 μM). (b) Fluorescent spectra of HMB-NA@Mp (10 μM) before/after the addition of HOCl (15 μM). (c) Standard curve of the HMB-NA content. | |
Then, in order to further verify the successful encapsulation of HMB-NA by Mp, we measured the fluorescence and ultraviolet absorption spectra of HMB-NA@Mp in the presence and absence of HClO (Fig. 4D). The results showed that the optical properties of HMB-NA@Mp were consistent with HMB-NA by comparison. At the same time, the content standard curve of HMB-NA was established by UV spectrophotometry (Fig. 4D). According to the regression equation of the standard curve, the encapsulation efficiency was measured as 45.34%. All the results showed that HMB-NA had been successfully coated in Mp. Moreover, it still retained favorable spectral response properties, which is key to ensure that HMB-NA@Mp can be enriched at the disease site and achieve accurate detection.
3.3 Cell viability of RAW 264.7, HUVEC, and foam cells
Endothelial cells and macrophages play a key role in the development of AS. To evaluate the biocompatibility of the probe, we investigated the effects of HMB-NA and HMB-NA@Mp on the survival rates of RAW264.7, HUVEC, and foam cells by the MTT method. First, HMB-NA and HMB-NA@Mp were incubated with RAW264.7 and HUVEC cells for 24 h and 48 h, respectively. As shown in Fig. 5A and B, the survival rate of RAW 264.7 and HUVEC cells decreased with the increasing concentration of free HMB-NA, thus showing a dose dependence. However, when coated with Mp, the toxicity was reduced at the same dose, indicating that HMB-NA@Mp formed by coating with biofilm materials may not be phagocytosed by macrophages and a reduced leakage of free HMB-NA, which made HMB-NA@Mp have low toxicity. It was noteworthy that the survival rate of HMB-NA@Mp for both types of cells was above 80% even at a high concentration. The toxicities of HMB-NA and HMB-NA@Mp to RAW 264.7 and HUVEC cells were higher than that at 24 h when the cells were incubated for 48 h, but HMB-NA@Mp still showed low toxicity when the incubation time was increased compared to free HMB-NA. This suggested that HMB-NA@Mp would not exacerbate the development of endothelial damage and inflammation in AS, which lays the foundation for the safe use of the interventional probe in living organisms.
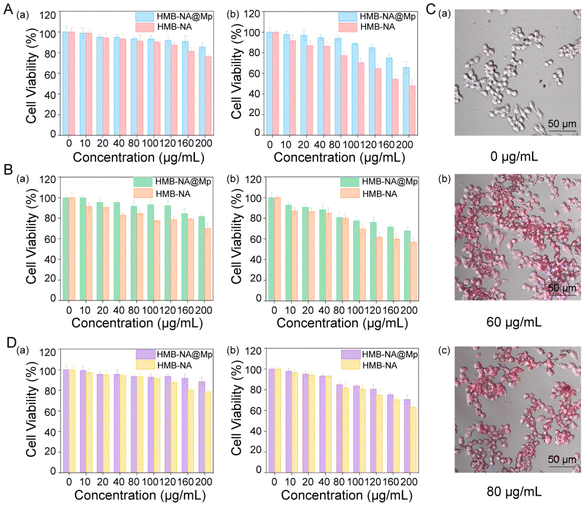 |
| Fig. 5 (A) Cytotoxicity of different treatments on RAW 264.7 cells for: (a) 24 h; (b) 48 h. (B) Cytotoxicity of different treatments on HUVEC cells for: (a) 24 h; (b) 48 h. (C) Cytotoxicity of different treatments on foam cells for: (a) 24 h; (b) 48 h. (D) Foam cells formation. | |
According to the mechanism of AS, the formation and apoptosis of foam cells are early events and key factors in the development of AS. So we induced foam cell models by treating RAW 264.7 cells with different concentrations of ox-LDL. As could be seen from Fig. 5C, when the concentration of ox-LDL was 60 μg mL−1, the RAW 264.7 cells contained a large number of lipid droplets, which was consistent with the characteristics of foam cells, indicating the formation of foam cells. When the concentration of ox-LDL was 80 μg mL−1, some lipid droplets aggregated into larger lipid droplets. Therefore, in subsequent cell experiments we used the concentration of 60 μg mL−1 ox-LDL to treat RAW 264.7 cells. Then, the influence of HMB-NA@Mp on the survival rate of foam cells was also studied. As shown in Fig. 5D, compared with uncoated HMB-NA, HMB-NA@Mp showed lower toxicity at the same dose, and the foam cells still had a higher survival rate even at a high concentration and after being incubated for a long time. This also indicated that even at a high concentration, HMB-NA@Mp will not cause the apoptosis and rupture of foam cells or promote the formation of AS plaques.
3.4 Fluorescent imaging and uptake of foam cells
As is well known, the uptake of by foam cells is a critical basis to make sure of the specific recognition on foam cells. Therefore, we further studied the uptake of HMB-NA@Mp in RAW 264.7 and foam cells. According to the flow cytometry data of cell uptake in Fig. 6A, the interventional probe HMB-NA@Mp could be uptaken by foam cells rather than RAW 264.7 cells. Also, laser scanning confocal microscopy was used to observe the red fluorescence of the interventional probe and the blue fluorescence of DAPI of the nucleus in the two cells. As shown in Fig. 6B, even if the free probe HMB-NA was absorbed by RAW 264.7 cells, almost no obvious fluorescence was observed around the nucleus in the RAW 264.7 cells. However, both the HMB-NA and HMB-NA@Mp groups showed strong red fluorescence emission in foam cells, especially the HMB-NA@Mp group. This perfectly illustrated that HMB-NA@Mp could selectively accumulate in foam cells and could specifically identify foam cells from macrophages at the same time. This was probably because the intracellular HClO in foam cells stimulated by ox-LDL was significantly higher than in macrophages. Moreover, the fluorescence in the foam cells and RAW 264.7 cells at 4 h (Fig. 6C) observed by confocal laser scanning microscopy also confirmed that HMB-NA had an excellent ability to selectively identify foam cells in vivo.
 |
| Fig. 6 (A) Cell uptake of HMB-NA@Mp and free HMB-NA by flow cytometry. (B) Cell uptake of HMB-NA@Mp and free HMB-NA by RAW 264.7 and foam cells. (C) CLSM images of cell uptake at 4 h. | |
3.5
In vivo imaging and biosafety evaluation of HMB-NA@Mp
In order to evaluate whether the early AS model was successfully constructed, we stained the thoracic aorta with hematoxylin & eosin (H&E) and measured the changes of blood lipids in each group (Fig. 7A and B). Compared with the results at week 0, with the increase in modeling time, the aorta of the model group gradually showed endothelial injury. After 6 weeks of high-fat diet, it showed severe endothelial damage and large foam cell formation, and the level of LDL-C in the blood lipids was increased significantly. This indicated that the early AS model was successfully constructed.
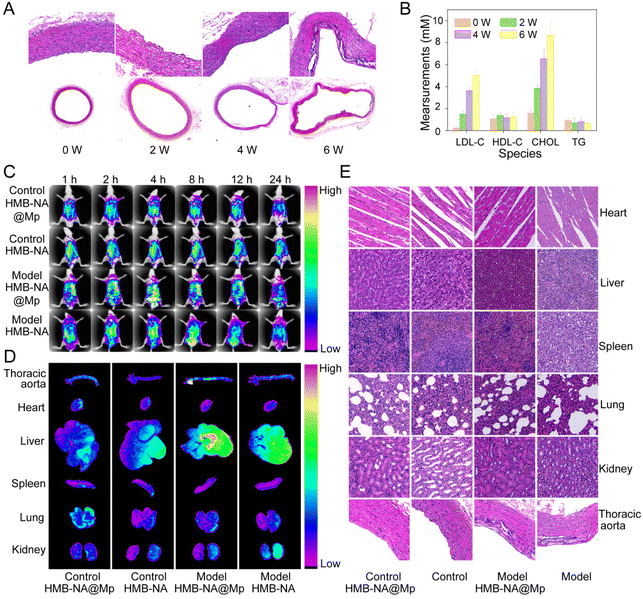 |
| Fig. 7 (A) Changes in H&E staining sections of the thoracic aorta. (B) Changes in blood lipids in the evolution of AS. (C) Fluorescence imaging of HMB-NA@Mp in different groups of rats. (D) Main organs’ distribution in different groups of rats after the injection of HMB-NA@Mp (4 h). (E) H&E stain of main organs of rats in each group after the injection of HMB-NA@Mp. | |
After the early AS model was constructed, we used the small animal in vivo imaging system (IVIS) to examine the ability of the interventional probe to detect foam cells in early AS rats in vivo. As Fig. 7C shows, after injecting HMB-NA@Mp for 1 h, the fluorescence signal could be observed in the thoracic aorta of the model group rats. After 4 h, the fluorescence observed was significantly enhanced. However, there were no obvious fluorescence signal in the control group rats. These results indicated that HMB-NA@Mp could successfully detect early AS at the animal level. Moreover, we observed the disappearance of the fluorescence signal after injecting HMB-NA@Mp for 24 h, which indicated that the probe could be quickly cleared without residual toxicity and had high biosafety.
To more accurately characterize the foam cells targeted-detection ability to HMB-NA@Mp, after the injection 4 h of different probes, the heart, liver, spleen, lung, kidney, and aorta of the rats in each group were dissected, and then we obtained the in vitro fluorescence images from the imaging system. As the images show in Fig. 7D, in the thoracic aortic arch of the model group rats injected with HMB-NA@Mp, we could observe that there was a stronger fluorescence accumulation than those injected with HMB-NA. While there was no fluorescence signal found in the aorta of the control group rats. These results once again verified the foam cells targeting characteristics and excellent AS detection ability of HMB-NA@Mp, which were consistent with the in vivo imaging results.
In order to further evaluate the biosafety of HMB-NA@Mp, we dissected the main organs of the rats in each groups and stained them with H&E. As the results show in Fig. 7E, through comparison of the injected probe group with the non-injected group, it could be observe that the probe caused no injury to the major organs or thoracic aorta, which indicated that HMB-NA@Mp had no acute injury effect on rats and can be used as a safe detection agent for early AS.
3.6 Therapeutic effect evaluation of HMB-NA@Mp
In order to investigate the intervention effect and the long-term biosafety of HMB-NA@Mp, we injected HMB-NA@Mp in to the tail vein of rats for two weeks. First, according to the H&E staining results shown in Fig. 8A, compared with the rats in the intervention group and the non-intervention group, the main organs and aorta did not show toxic effects, which indicated that the probe had good biosafety. An abnormal blood lipid level, especially an abnormal increase in LDL, is the key risk factor and important feature of AS. Thus, we studied the blood lipid changes during the intervention process and the results are shown in Fig. 8B, and by comparing the two groups of rats, it could be seen that the levels of LDL and CHOL in the blood lipids in the intervention group were decreased. This may be due to the metabolites released by the interventional probe, which played a role in regulating the blood lipids. In addition, as the results from the gross oil red O staining of the thoracic aorta shown in Fig. 8C, lipid droplets were found in the thoracic aortic arches of the rats in the non-intervention group, but not in the intervention group and control group. This preliminary indicated that the probe had an intervention effect on the regulation of blood lipids in AS rats, and further research is needed in this area.
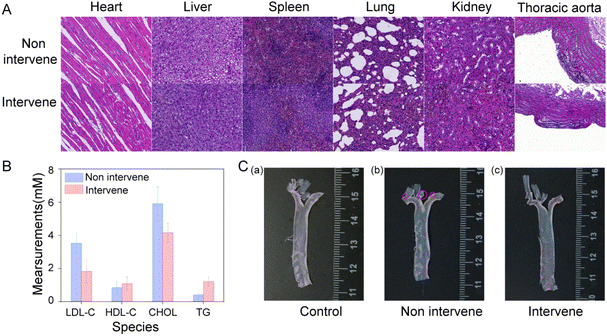 |
| Fig. 8 (A) H&E staining of the main organs of the rats in each group. (B) Changes in blood lipids of the rats in each group. (C) Gross oil red O staining of the thoracic aorta in each group. | |
4 Conclusion
In summary, a novel NIR fluorescent interventional probe was constructed for the first time for imaging HClO in foam cells and for simultaneously preventing the further development of AS. This probe displayed high sensitivity and reactivity, which could rapidly produce a fluorescence “off–on” response to HClO within 5 s. Additionally, the high biocompatibility and deep tissue penetration of HMB-NA@Mp enabled it to better monitor HOCl generation in vivo in real time. Compared to the control group, HMB-NA@Mp could accumulate in the thoracic aorta of early AS model rats and generate significant fluorescent signals enhancement. Importantly, HMB-NA@Mp enabled a very satisfactory effectiveness in improving the blood lipids, which was because of the metabolites NA. Based on the pathogenesis of AS, we speculated that HMB-NA@Mp could be used to detect AS a decade or two earlier in patients. We thus envision that the successful design of the interventional probe could serve as a robust approach to contribute to future biomedical research on AS early detection and early intervention, and it could also provide a theoretical basis for the early detection of other oxidative stress diseases.
Author contributions
Jie Zhou: conceptualization, writing – review & editing, supervision, funding acquisition. Ruhe Yang: conceptualization, methodology, investigation, writing – original draft. Yiwen Sun: methodology, investigation. Fusui Luo: methodology, investigation. Jin Zhang: methodology. Huili Ma: methodology. Min Guan: methodology. All of the authors have read and approved the manuscript.
Conflicts of interest
There are no conflicts of interest to declare.
Acknowledgements
The work was supported by the National Natural Science Foundation of China (grant no. U1904135).
References
- W. Gao, Y. Zhao, X. Li, Y. Sun, M. Cai, W. Cao, Z. Liu, L. Tong, G. Cui and B. Tang, Chem. Sci., 2018, 9, 439–445 RSC.
- M. Zhou, H. Wang, X. Zeng, P. Yin, J. Zhu, W. Chen, X. Li, L. Wang, L. Wang, Y. Liu, J. Liu, M. Zhang, J. Qi, S. Yu, A. Afshin, E. Gakidou, S. Glenn, V. S. Krish, M. K. Miller-Petrie, W. C. Mountjoy-Venning, E. C. Mullany, S. B. Redford, H. Liu, M. Naghavi, S. I. Hay, L. Wang, C. J. L. Murray and X. Liang, Lancet, 2019, 394, 1145–1158 CrossRef PubMed.
- L. Huang, K. L. Chambliss, X. Gao, I. S. Yuhanna, E. Behling-Kelly, S. Bergaya, M. Ahmed, P. Michaely, K. Luby-Phelps, A. Darehshouri, L. Xu, E. A. Fisher, W. P. Ge, C. Mineo and P. W. Shaul, Nature, 2019, 569, 565–589 CrossRef CAS.
- R. S. Rosenson, H. B. Brewer Jr., W. S. Davidson, Z. A. Fayad, V. Fuster, J. Goldstein, M. Hellerstein, X. C. Jiang, M. C. Phillips, D. J. Rader, A. T. Remaley, G. H. Rothblat, A. R. Tall and L. Yvan-Charvet, Circulation, 2012, 125, 1905–1919 CrossRef.
- P. Libby, Arterioscler., Thromb., Vasc. Biol., 2012, 32, 2045–2051 CrossRef CAS.
- X. Zhang, B. Wang, C. Wang, L. Chen and Y. Xiao, Anal. Chem., 2015, 87, 8292–8300 CrossRef CAS.
- J. Zhang, Y. Zu, C. S. Dhanasekara, J. Li, D. Wu, Z. Fan and S. Wang, Wiley Interdiscip. Rev.: Nanomed. Nanobiotechnol., 2017, 9 DOI:10.1002/wnan.1412.
- S. Robichaud, A. Rasheed, A. Pietrangelo, A. D. Kim, D. M. Boucher, C. Emerton, V. Vijithakumar, L. Gharibeh, G. Fairman, E. Mak, M. A. Nguyen, M. Geoffrion, R. Wirka, K. J. Rayner and M. Ouimet, Circ. Res., 2022, 130, 831–847 CrossRef CAS PubMed.
- B. G. Childs, D. J. Baker, T. Wijshake, C. A. Conover, J. Campisi and M. v. J. Deursen, Science, 2016, 354, 472–477 CrossRef CAS PubMed.
- A. C. Li and C. K. Glass, Nat. Med., 2002, 8, 1235–1242 CrossRef CAS PubMed.
- R. P. Choudhury, J. M. Lee and D. R. Greaves, Nat. Clin. Pract. Cardiovasc. Med., 2005, 2, 309–315 CrossRef CAS.
- M. Nahrendorf and F. K. Swirski, Circ. Res., 2015, 116, 884–894 CrossRef CAS PubMed.
- S. Zhang, W. Xu, P. Gao, W. Chen and Q. Zhou, Artif. Cells, Nanomed., Biotechnol., 2020, 48, 169–179 CrossRef CAS.
- P. Nicol, P. Hoppman, K. Euller, E. Xhepa, T. Lenz, H. Rai, H. Jinnouchi, A. Bulin, M. I. Castellanos, A. L. Lahmann, T. Koppara, A. Kastrati and M. Joner, Int. J. Cardiovasc. Imaging, 2021, 37, 25–35 CrossRef PubMed.
- I. L. Aanei, T. Huynh, Y. Seo and M. B. Francis, Bioconjugate Chem., 2018, 29, 2526–2530 CrossRef PubMed.
- R. A. Chmielowski, D. S. Abdelhamid, J. J. Faig, L. K. Petersen, C. R. Gardner, K. E. Uhrich, L. B. Joseph and P. V. Moghe, Acta Biomater., 2017, 57, 85–94 CrossRef PubMed.
- S. Park, J. W. Ahn, Y. Jo, H. Y. Kang, H. J. Kim, Y. Cheon, J. W. Kim, Y. Park, S. Lee and K. Park, ACS Nano, 2020, 14, 1856–1865 CrossRef PubMed.
- G. Schmitz and M. Grandl, Antioxid. Redox Signal., 2007, 9, 1499–1518 CrossRef.
- H. Feng, Z. Zhang, Q. Meng, H. Jia, Y. Wang and R. Zhang, Adv. Sci., 2018, 5, 1800397 CrossRef PubMed.
- B. Wang, F. Zhang, S. Wang, R. Yang, C. Chen and W. Zhao, Chem. Commun., 2020, 56, 2598–2601 RSC.
- M. M. Zhang, Y. H. Ma, P. Li, Y. Jia and K. L. Han, Chem. Commun., 2020, 56, 2610–2613 RSC.
- X. Wu, W. Shi, X. Li and H. Ma, Acc. Chem. Res., 2019, 52, 1892–1904 CrossRef.
- J. Han, X. Liu, H. Xiong, J. Wang, B. Wang, X. Song and W. Wang, Anal. Chem., 2020, 92, 5134–5142 CrossRef PubMed.
- C. Duan, M. Won, P. Verwilst, J. Xu, H. S. Kim, L. Zeng and J. S. Kim, Anal. Chem., 2019, 91, 4172–4178 CrossRef.
- T. Yudhistira, S. V. Mulay, Y. Kim, M. B. Halle and D. G. Churchill, Chem. – Asian J., 2019, 14, 3048–3084 CrossRef PubMed.
- Q. Wang, R. Lou, Q. Yin, R. Yang, S. Li and J. Zhou, Analyst, 2021, 146, 4674–4682 RSC.
- C. C. Winterbourn, Nat. Chem. Biol., 2008, 4, 278–286 CrossRef.
- S. Lu, L. Sui, J. Liu, S. Zhu, A. Chen, M. Jin and B. Yang, Adv. Mater., 2017, 29, 1603443–1603448 CrossRef.
- C. L. Shen, Q. Lou, J. H. Zang, K. K. Liu, S. N. Qu, L. Dong and C. X. Shan, Adv. Sci., 2020, 7, 1903525 CrossRef.
- K. Hola, M. Sudolska, S. Kalytchuk, D. Nachtigallova, A. L. Rogach, M. Otyepka and R. Zboril, ACS Nano, 2017, 11, 12402–12410 CrossRef PubMed.
- X. Lian, M. Y. Wei and Q. Ma, Front. Bioeng. Biotechnol., 2019, 7, 386 CrossRef.
- H. C. Junqueira, D. Severino, L. G. Dias, M. S. Gugliotti and M. S. Baptista, Phys. Chem. Chem. Phys., 2002, 4, 2320–2328 RSC.
- M. Wainwright, D. A. Phoenix, L. Rice, S. M. Burrow and J. Waring, J. Photochem. Photobiol., B, 1997, 40, 233–239 CrossRef.
- P. Wei, W. Yuan, F. Xue, W. Zhou, R. Li, D. Zhang and T. Yi, Chem. Sci., 2018, 9, 495–501 RSC.
- X. Wei, M. Ying, D. Dehaini, Y. Su, A. V. Kroll, J. Zhou, W. Gao, R. H. Fang, S. Chien and L. Zhang, ACS Nano, 2018, 12, 109–116 CrossRef.
- C. M. Hu, R. H. Fang, K. C. Wang, B. T. Luk, S. Thamphiwatana, D. Dehaini, P. Nguyen, P. Angsantikul, C. H. Wen, A. V. Kroll, C. Carpenter, M. Ramesh, V. Qu, S. H. Patel, J. Zhu, W. Shi, F. M. Hofman, T. C. Chen, W. Gao, K. Zhang, S. Chien and L. Zhang, Nature, 2015, 526, 118–121 CrossRef PubMed.
- M. Yin, J. Lin, M. Yang, C. Li, P. Wu, J. Zou, Y. Jiang and J. Shao, Colloids Surf., B, 2022, 214, 112464 CrossRef PubMed.
- Y. Song, Z. Huang, X. Liu, Z. Pang, J. Chen, H. Yang, N. Zhang, Z. Cao, M. Liu, J. Cao, C. Li, X. Yang, H. Gong, J. Qian and J. Ge, Nanomedicine, 2019, 15, 13–24 CrossRef.
- P. Wei, L. Liu, Y. Wen, G. Zhao, F. Xue, W. Yuan, R. Li, Y. Zhong, M. Zhang and T. Yi, Angew. Chem., 2019, 131, 4595–4599 CrossRef.
- L. C. Pereira, E. S. de Paula, M. Pazin, M. F. H. Carneiro, D. Grotto, F. Barbosa Jr. and D. J. Dorta, Drug Chem. Toxicol., 2020, 43, 64–70 CrossRef.
- S. Montserrat-de la Paz, M. C. Naranjo, S. Lopez, R. Abia, F. J. G. Muriana and B. Bermudez, J. Nutr. Biochem., 2017, 39, 40–47 CrossRef PubMed.
- Y. Li, G. Yang, X. Yang, W. Wang, J. Zhang, Y. He, W. Zhang, T. Jing and R. Lin, Int. Immunopharmacol., 2016, 40, 211–218 CrossRef PubMed.
- H. Huang, P. Koelle, M. Fendler, A. Schroettle, M. Czihal, U. Hoffmann and P. J. Kuhlencordt, PLoS One, 2014, 9, e114643 CrossRef PubMed.
- S. H. Ganji, S. Qin, L. Zhang, V. S. Kamanna and M. L. Kashyap, Atherosclerosis, 2009, 202, 68–75 CrossRef.
- S. H. Ganji, V. S. Kamanna and M. L. Kashyap, Atherosclerosis, 2014, 235, 554–561 CrossRef.
- K. Zhang, Y. Lv, J. Meng, J. Wang, A. Peng, X. Wang and Z. Tian, Anal. Chim. Acta, 2019, 1061, 142–151 CrossRef.
|
This journal is © The Royal Society of Chemistry 2023 |
Click here to see how this site uses Cookies. View our privacy policy here.