DOI:
10.1039/D2AN01693A
(Paper)
Analyst, 2023,
148, 38-46
A dual-response mitochondria-targeted NIR fluorescent probe with large Stokes shift for monitoring viscosity and HOCl in living cells and zebrafish†
Received
15th October 2022
, Accepted 16th November 2022
First published on 17th November 2022
Abstract
Mitochondria are important subcellular organelles involved in many cellular activities. Therefore, it is very important to monitor the concentration of various substances in mitochondria. In this work, we constructed a dual-response mitochondria-targeted fluorescent probe HBTN for the detection of viscosity and HOCl in vitro and in vivo. HBTN not only has a long emission wavelength with an emission peak of 680 nm, but also has a large Stokes shift of 278 nm. The fluorescence intensity of probe HBTN at 680 nm has a good linear relationship with solution viscosity, which can be used for quantitative detection of viscosity. In addition, the probe HBTN enables ratiometric detection of HOCl with a low detection limit (DL = 24.5 nM) and rapid response (<20 min). More importantly, the probe HBTN has been successfully applied to the detection of viscosity and exogenous/endogenous HOCl in the mitochondria of MCF-7 cells. Furthermore, the probe HBTN has been implemented in imaging viscosity and HOCl in zebrafish. HBTN is expected to be a practical tool for monitoring viscosity and HOCl in vivo.
1. Introduction
Mitochondria are vital subcellular organelles that are not only cells’ energy manufacturers, capable of producing ATP, but also play a role in a lot of cellular processes, such as cell differentiation and death.1–4 According to related research, the physiological activities of mitochondria are significantly influenced by viscosity and the amount of ROS.5–7 Intracellular viscosity is a crucial microenvironmental factor that affects the diffusion rate of different biological molecules.8,9 A balance of cellular viscosity is necessary to sustain several diffusion-mediated functions, including signaling, enzyme catalysis, and diffusion of metabolites.10–13 However, many diseases, including hypertension, diabetes, atherosclerosis, cell cancer, and Alzheimer's disease, are brought about by abnormal intracellular viscosity.14–19
On the other hand, hypochlorous acid (HOCl) is one of the important reactive oxygen species in living organisms. HOCl is a typical ROS created by the reaction between chloride ions and hydrogen peroxide, which is catalyzed by MPO enzymes.20–23 In the biological environment, HOCl is essential for defending against outside pathogens and preserving redox balance.24–27 However, excessive HOCl will destroy important substances in living organisms and lead to a lot of diseases including atherosclerosis, cancer, neurodegenerative diseases, and inflammation.28–33 Moreover, it has been reported in the literature that the diffusion rate of hypochlorous acid is closely related to viscosity under conditions such as oxidative stress or inflammation.34,35 Monitoring the relationship between hypochlorous acid and viscosity will contribute to the study of related physiological activities and disease mechanisms. For the early identification and treatment of associated disorders, it is crucial to develop a strong method for measuring mitochondrial viscosity and HOCl.
Fluorescence imaging detection has been widely employed for substance detection in biological environments due to the benefits of non-invasive in situ detection, rapid real-time analysis, and high sensitivity.36–42 So far, many fluorescent probes have been reported for the detection of viscosity or hypochlorous acid, respectively.43–50 Although some fluorescent probes have been reported for the detection of viscosity and HOCl (Table S2†), few fluorescent probes have been reported for the detection of mitochondrial viscosity and HOCl.34,51–54 In addition, near-infrared fluorescent probes are more suitable for biological imaging due to their advantages such as strong tissue penetration, less interference from biological autofluorescence, and less damage to biological samples.55–57 Fluorescent probes with large Stokes shifts can avoid spectral crosstalk, thereby reducing self-absorption during imaging and improving the signal-to-noise ratio of detection.58–60 However, there are currently very few fluorophores with both near-infrared emission and large Stokes shift. Thus, it is of great significance to develop a NIR fluorescent probe with large Stokes shift for the detection of mitochondrial viscosity and HOCl.
Therefore, we designed and synthesized a novel dual-response NIR fluorescent probe (HBTN) with large Stokes shift to monitor mitochondrial viscosity and HOCl, and its response mechanism is described in Scheme 1. HBTN consists of two moieties, 2-(2′-hydroxyphenyl)benzothiazole (HBT) and 1-methylpyridin-1-ium, linked by a conjugated structure. HBTN has near-infrared emission at 680 nm and a large Stokes shift of 278 nm. The probe HBTN can monitor viscosity by the change of fluorescence intensity at 680 nm, and its fluorescence intensity increases with the increase of viscosity. The fluorescence intensity (log
I680) has a good linear relationship with solution viscosity (log viscosity), which can be used for quantitative detection of viscosity. Moreover, HBTN can rapidly (<20 min) and specifically detect HOCl with high sensitivity (DL = 24.5 nM) accompanied by ratiometric fluorescence changes. More importantly, the probe HBTN has been successfully applied to the monitoring of viscosity and exogenous/endogenous HOCl in the mitochondria of MCF-7 cells. Besides, the probe HBTN exhibits the ability to image viscosity and HOCl in zebrafish.
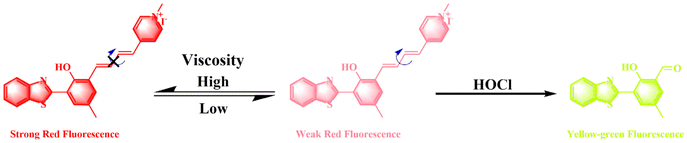 |
| Scheme 1 Detection of viscosity and HOCl with fluorescent probe HBTN. | |
2. Experimental section
2.1 General
Unless otherwise stated, all reagents and solvents mentioned in this work were purchased and used without purification. All instruments used in the experiments are listed in the ESI.† Samples were mounted on 10 mm wide quartz cuvettes for recording all spectra. Fluorescence spectra were recorded using 405 nm as the excitation wavelength. When measuring viscosity, the excitation/emission slits were 10 nm and 8 nm, respectively. When measuring HOCl, the excitation/emission slits were 10 nm and 20 nm, respectively. Frontier molecular orbitals were calculated using the software Orca 5.0.3.61–63 Energy-optimized geometries were plotted with the software Multiwfn.64
2.2 Synthesis
Synthesis details of the intermediate compounds HBTOH, HBTOF and HBTDF are provided in the ESI.†
Synthesis of probe HBTN.
HBTDF (100 mg, 0.339 mmol) and 1,4-dimethylpyridinium iodide (96.0 mg, 0.407 mmol) were dissolved in dichloromethane (20 mL) followed by the addition of piperidine (100 μL). In a nitrogen atmosphere, the reaction was carried out at room temperature for 24 h. Excess hydriodic acid was added after removal of the solvent by reduced pressure. After ethyl acetate was added and reflux was carried out, there was significant solid formation. After stopping the reflux and cooling to room temperature, we obtained the probe HBTN (46 mg, 26%) as a black-red solid by suction filtration. 1H NMR (400 MHz, DMSO-d6) δ 12.93 (s, 1H), 8.80 (d, J = 6.5 Hz, 2H), 8.22 (d, J = 8.0 Hz, 1H), 8.14 (dd, J = 12.3, 7.2 Hz, 3H), 7.92 (dd, J = 15.5, 8.4 Hz, 1H), 7.71 (d, J = 6.4 Hz, 2H), 7.61 (t, J = 7.7 Hz, 1H), 7.53 (t, J = 7.5 Hz, 1H), 7.41 (d, J = 8.6 Hz, 2H), 6.91 (d, J = 15.4 Hz, 1H), 4.23 (s, 3H), 2.38 (s, 3H). 13C NMR (100 MHz, DMSO-d6) δ 168.70, 153.23, 152.30, 150.91, 144.88, 142.42, 134.23, 132.38, 131.15, 129.54, 129.14, 129.05, 127.18, 126.56, 126.08, 124.59, 123.23, 122.45, 121.96, 116.77, 46.79, 20.00. HRMS (ESI): calcd for C24H21N2OS+ [M − I−]+m/z, 385.1369; found, 385.1370.
2.3 Cytotoxicity assay
Cytotoxicity of probe HBTN was evaluated by MTT assay. We calculated the cell viability using the formula (As − Ab)/(Ac − Ab) × 100%, where As, Ac and Ab correspond to the absorbance of the sample, blank and control at 490 nm, respectively.
2.4 Confocal fluorescence bioimaging
MCF-7 cells were cultured in RPMI 1640 culture medium containing 10% FBS in an incubator at 37 °C and 5% CO2. We used the probe HBTN to detect mitochondrial viscosity and HOCl in MCF-7 cells. First, we co-incubated MCF-7 cells with the probe HBTN and a commercial mitochondrial dye (Mito-Tracker Green) for colocalization experiments. MCF-7 cells were incubated with Mito-Tracker Green (0.5 μM) for 30 min and then incubated with HBTN (10 μM) for another 30 min. Next, the probe HBTN was used to test the viscosity in mitochondria in MCF-7 cells. After treatment with nystatin (10 μM) for 30 min, the MCF-7 cells were incubated with probe HBTN for 30 min. In addition, we also conducted experiments on the detection effect of probe HBTN on exogenous and endogenous HOCl in mitochondria. In the detection experiment of exogenous HOCl, MCF-7 cells were first treated with different concentrations of HOCl (20 and 40 μM) for 30 min, and then incubated with the probe HBTN (10 μM) for 30 min. For the imaging of exogenous HOCl, MCF-7 cells were first treated with LPS (5 μg mL−1) + PMA (5 μg mL−1) or LPS (5 μg mL−1) + PMA (5 μg mL−1) + NAC (1 mM) for 3 h, and then incubated with the probe HBTN (10 μM) for 30 min. All MCF-7 cells were washed three times with PBS before imaging.
We used four-day-old zebrafish for bioimaging experiments. All animal procedures were performed in accordance with the Guidelines for Care and Use of Laboratory Animals of Nanjing University and approved by the Animal Ethics Committee of Nanjing University. First, the effect of the probe HBTN on imaging viscosity in vivo was explored. Zebrafish were incubated with nystatin (10 μM) for 30 min and then with probe HBTN (10 μM) for 30 min. Zebrafish cultured only with the probe HBTN (10 μM) for 30 min was used as the control group. In addition, the response of probe HBTN to HOCl in vivo was also tested. Zebrafish were first treated with HOCl (20 μM) for 30 min, LPS (5 μg mL−1) for 4 h or LPS (5 μg mL−1) + NAC (1 mM) for 4 h, and then incubated with HBTN (10 μM) for 30 min. Similarly, zebrafish were cultured only with HBTN for 30 min as a control group. Before imaging, we washed all zebrafish three times with PBS.
3. Results and discussion
3.1 Design and synthesis of probe HBTN
The probe HBTN is composed of two parts, namely 2-(2′-hydroxyphenyl)benzothiazole and 1-methylpyridin-1-ium linked by a flexible conjugated linker. HBTN exhibits viscosity-sensitive properties due to the twisted intramolecular charge transfer (TICT) mechanism. Under high viscosity conditions, the intramolecular rotation of HBTN is suppressed, resulting in higher fluorescence intensity. The quaternary ammonium salt group makes HBTN very water-soluble and gives HBTN the ability to target mitochondria. On the other hand, due to the oxidative property of HOCl, the C
C of HBTN can be destroyed, resulting in a shift of the fluorescence emission peak. The probe HBTN was synthesized through a four-step reaction as shown in Scheme 2. HBTN and intermediate compounds were characterized by NMR and mass spectrometry (Fig. S1–S12†).
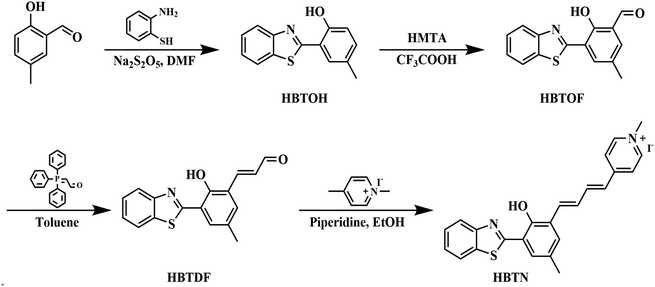 |
| Scheme 2 Synthetic route to probe HBTN. | |
3.2 Optical response of HBTN to viscosity
With the probe HBTN in hand, we first investigated the optical response of probe HBTN to different viscosity systems (water–glycerol mixed solvents) at 25 °C. As shown in Fig. 1a, the fluorescence intensity of probe HBTN was weak in the low-viscosity water solution. With the increase of glycerol ratio, the viscosity of the solution increased and the fluorescence intensity enhanced significantly. This phenomenon was further demonstrated by the high quantum yield of HBTN in glycerol (ΦF = 19.43%, Table S1†). Fluorescence enhancement can be directly observed with the naked eye under 365 nm UV light (Fig. S15†). It was found that the fluorescence intensity (log
I680) at 680 nm had a good linear relationship with the solution viscosity (log viscosity) in the range of 10.08–1412 cP (linear equation: y = 0.17141x + 2.24606, R2 = 0.99626) (Fig. 1b). In addition, the increase of viscosity will increase the fluorescence lifetime of the probe HBTN, and there is a good linear relationship between them (linear equation: y = 0.20041x + 0.66320, R2 = 0.97252, 1.01–10.08 cP, Fig. 1c and d). The above results reveal that the probe HBTN can be applied to quantitatively detect viscosity.
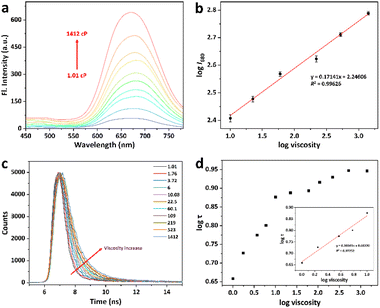 |
| Fig. 1 (a) Fluorescence emission spectra of HBTN (10 μM) in different viscosity systems (water–glycerol mixed solvents). (b) Linear relationship of log I680 and log viscosity. (c) Fluorescence lifetime curves of probe HBTN obtained in solutions of different viscosities (λex = 405 nm). (d) Corresponding fluorescence lifetimes at different viscosities, inset: linear relationship of log τ and log viscosity. | |
3.3 Optical response of HBTN toward HOCl
On the other hand, we performed the response tests of HBTN toward HOCl in PBS (10 mM, pH = 7.4, 20% CH3CN) at 25 °C. There were three absorption peaks at 309 nm, 402 nm and 510 nm for the probe HBTN solution (Fig. 2b). The absorption intensity of the three absorption peaks gradually decreased in the presence of HOCl. It can be obviously found that the color of the probe HBTN solution changed from light yellow to light red with the increase of HOCl concentration. The absorption intensity (A402) had a good linear relationship with the concentration of HOCl in the range of 0–5 μM (linear equation: y = −0.02275x + 0.22789, R2 = 0.98918) (Fig. S16 and S17†). As depicted in Fig. 2a, the probe HBTN solution had NIR fluorescence emission at 680 nm and a large Stokes shift of 278 nm. When hypochlorous acid was added, the fluorescence emission peak at 680 nm gradually decreased and the fluorescence emission peak at 530 nm gradually increased (Fig. 2c and d). Fluorescence emission exhibited a ratiometric change and the emission peak was shifted by 150 nm. As the amount of HOCl increased, we could clearly see that the probe solution changed from red fluorescence to yellow-green fluorescence under 365 nm UV light (Fig. 2e). The CIE chromaticity in Fig. 2f further demonstrates the change in fluorescence. It is surprising that an excellent linear relationship was found between the ratio of fluorescence intensities of the two emission peaks (I530/I680) and HOCl concentration (6–14 μM) (linear equation: y = 0.61099x − 1.77308, R2 = 0.99948). By using the calculation formula DL = 3σ/k, we calculated the detection limit to be 24.5 nM. The above experimental results show that the probe HBTN has high sensitivity to hypochlorous acid.
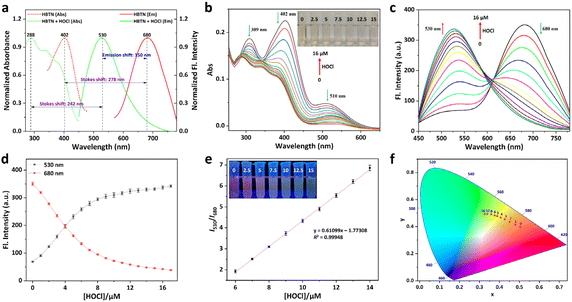 |
| Fig. 2 (a) Normalized absorption and fluorescence emission spectra of HBTN (10 μM) and HBTN (10 μM) + HOCl (16 μM). (b) Absorption spectra of HBTN in the presence of HOCl (0–16 μM), inset: photograph of HBTN (10 μM) under visible light in the presence of HOCl (0, 2.5, 5, 7.5, 10, 12.5, and 15 μM). (c) Fluorescence emission spectra of HBTN (10 μM) in the presence of HOCl (0–16 μM). (d) Fluorescence intensities of HBTN (10 μM) at 530 nm and 680 nm in the presence of HOCl (0–16 μM). (e) Linear relationship between the ratio of fluorescence intensities (I530/I680) and concentration of HOCl (6–14 μM), inset: photograph of HBTN (10 μM) under 365 nm UV light in the presence of HOCl (0, 2.5, 5, 7.5, 10, 12.5, and 15 μM). (f) CIE chromaticity diagram of HBTN (10 μM) in the presence of HOCl (0–16 μM). | |
Furthermore, the stability and response speed of the probe HBTN were investigated. As shown in Fig. 3a, the fluorescence intensities of HBTN at 530 nm and 680 nm varied little under excitation light. When HOCl was added, the fluorescence changed rapidly and stabilized after 20 min. We then explored the effect of pH on the response of HBTN to HOCl. We found that the fluorescence of HBTN remained stable at different pH in the range of 4–10 (Fig. 3b). When the pH was in the range of 6–10, the probe HBTN had the ability to respond to hypochlorous acid. The above experimental results show that the probe HBTN can respond quickly and it can respond at physiological pH, which will help the application of HBTN in the physiological environment.
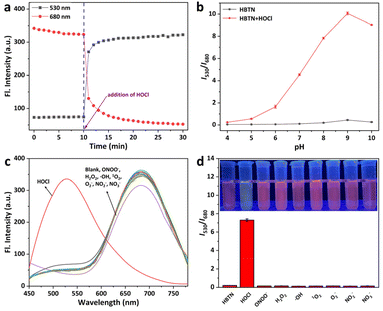 |
| Fig. 3 (a) Time profile of fluorescence intensities at 530 nm and 680 nm of HBTN (10 μM) before and after the addition of HOCl (20 μM). (b) Ratio of fluorescence intensities (I530/I680) of HBTN (10 μM) at different pH in the range of 4–10. (c) Fluorescence spectra of HBTN (10 μM) in the presence of HOCl (20 μM) or other ROS (200 μM). (d) Ratio of fluorescence intensities (I530/I680) and the photograph under 365 nm UV light of HBTN (10 μM) in the presence of HOCl (20 μM) or other ROS (200 μM). | |
In order to further confirm the application value of probe HBTN in the living environment, we need to test the selectivity of the probe HBTN. As shown in Fig. S18–S21,† when common biomolecules such as amino acids, metal cations, and acid anions were added, the fluorescence of the probe HBTN solution hardly changed. In addition, other active substances (ONOO−, H2O2, ˙OH, 1O2, O2−, NO2−, and NO3−) with similar chemical properties to HOCl will not cause fluorescence changes in the probe solution as well (Fig. 3c and d). However, the fluorescence of the probe HBTN solution changed significantly in the presence of HOCl. We can visually observe a clear color change in the probe solution after adding HOCl under visible light or 365 nm UV light, but this color change does not appear when other ROS are added (Fig. 3d and S22†). After performing the above selectivity studies, we can confirm that the probe HBTN has excellent selectivity for HOCl.
3.4 Response mechanism study
To explain the response of HBTN to viscosity, we performed theoretical calculations. In a low-viscosity environment, the intramolecular rotation enables the energy to be converted to a non-radiative form, so the probe HBTN has relatively low fluorescence intensity. Conversely, when the viscosity of the environment increases, the intramolecular rotation is suppressed and the fluorescence emission is enhanced. Furthermore, according to theoretical calculations, it can be seen that the highest occupied molecular orbital (HOMO) of HBTN is mainly located in the HBT component. However, the lowest unoccupied molecular orbital (LUMO) and LUMO+1 are mainly concentrated in the 1-methylpyridin-1-ium group and vinyl group (Fig. 4a and S23†). The frontier molecular orbital diagram shows that there is a strong electron transfer process from HBT to the 1-methylpyridin-1-ium group in the excited state, which is a typical TICT process. Therefore, according to the TICT mechanism, the probe HBTN is sensitive to solution viscosity. According to relevant literature reports,46,65 our proposed mechanism of the probe HBTN responding to HOCl is shown in Fig. 4b. The energy level difference between the LUMO and HOMO of the probe HBTN is significantly smaller than that of HBTOF, which confirms that the fluorescence emission of HBTN is red-shifted compared to that of HBTOF (Fig. 4a). To verify the response mechanism, we analyzed the reaction product between HBTN and HOCl by HRMS. The mass spectrum peak appeared at 268.8007, which is consistent with the generation of HBTOF (Fig. S24†). Through the above experiments, we verified the proposed response mechanism.
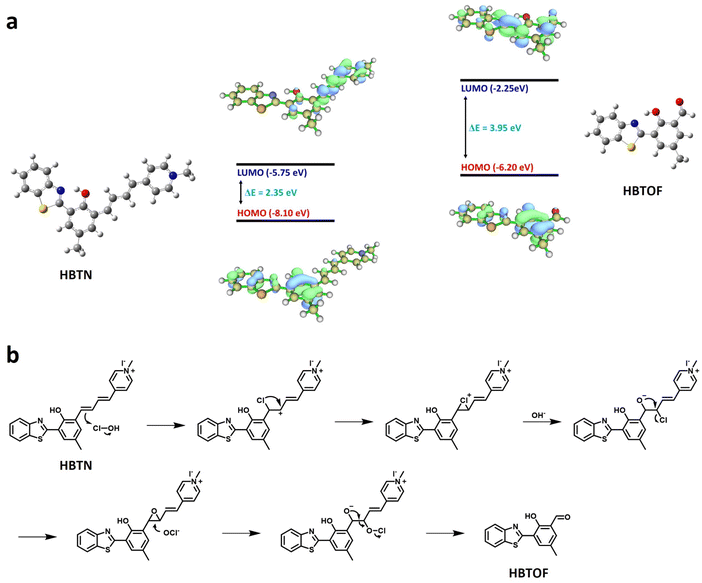 |
| Fig. 4 (a) Frontier molecular orbitals of HBTN and HBTOF. (b) Proposed response mechanism of HBTN toward HOCl. | |
3.5 Bioimaging experiments
Encouraged by the finding that the probe HBTN can detect viscosity and HOCl well in vitro, we attempted to apply the probe HBTN to bioimaging. Before performing imaging experiments, we first tested the cytotoxicity of the probe HBTN by MTT assay. As shown in Fig. S25,† HBTN has low cytotoxicity in the concentration range of 0–30 μM, and the cell viability can be maintained above 80% within HBTN concentration of 20 μM. In addition, we explored the phototoxicity of 405 nm laser irradiation in MCF-7 cells, and found that MCF-7 cells were not severely damaged after being continuously irradiated with a 405 nm laser for 40 min, so 405 nm laser irradiation is suitable for bioimaging using probe HBTN (Fig. S26†). Therefore, the probe HBTN can be used for detection in the biological environment.
The probe HBTN contains a 1-methylpyridin-1-ium group as a quaternary ammonium ion group, which can help the probe target the subcellular organelle mitochondria. We performed a colocalization experiment by co-incubating MCF-7 cells with the probe HBTN and Mito-Tracker Green, a commercially available mitochondria-targeting green dye. We conclude from Fig. 5 that the two channels have a good degree of overlap, with a Pearson's coefficient of 82%. The fluorescence intensity changes of the two channels in the linear region tend to be consistent (Fig. 5g). The above experimental results indicate that the probe HBTN has the ability to target mitochondria.
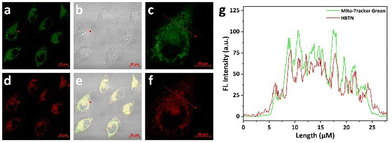 |
| Fig. 5 Co-incubation of MCF-7 cells with the probe HBTN and Mito-Tracker Green. (a) Mito-Tracker Green (λex = 488 nm, green channel: λem = 510–550 nm). (d) HBTN (λex = 405 nm, red channel: λem = 680–740 nm). (b) Bright field. (e) Overlap of (a), (d) and (b). Scale bar = 20 μm. (c and f) Magnified images of the red and green channels, respectively. Scale bar = 10 μm. (g) Fluorescence intensity profile of the linear region in MCF-7 cells. | |
Next, we further explored the ability of the probe HBTN to monitor mitochondrial viscosity. According to literature reports, nystatin can cause swelling of mitochondria, leading to increased mitochondrial viscosity.66 As shown in Fig. 6a, MCF-7 cells exhibited no fluorescence without any treatment. MCF-7 cells exhibited red fluorescence when incubated with the probe HBTN (Fig. 6b). In addition, the fluorescence intensity of MCF-7 cells incubated with HBTN after treatment with nystatin was greater than that without treatment with nystatin (Fig. 6b–d). Therefore, we can conclude that the probe HBTN can be used for the detection of mitochondrial viscosity.
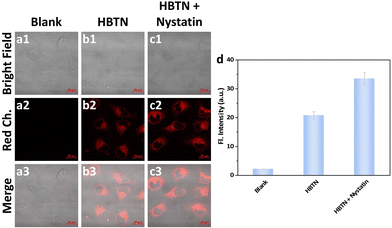 |
| Fig. 6 Fluorescence imaging of mitochondrial viscosity in MCF-7 cells. (a) MCF-cells without any treatment. (b) MCF-7 cells were incubated with HBTN (10 μM) for 30 min. (c) MCF-7 cells were first treated with nystatin (10 μM) for 30 min, and then incubated with probe HBTN (10 μM) for 30 min. λex = 405 nm, red channel: λem = 680–740 nm, scale bar = 20 μm. (d) Comparison of red channel fluorescence intensities. | |
Then, we investigated the ability of the probe HBTN to detect HOCl in mitochondria. As shown in Fig. 7a, when MCF-7 cells were incubated with HBTN only, obvious fluorescence was seen in the red channel, while only weak fluorescence was seen in the green channel. When exogenous hypochlorous acid was added, the fluorescence intensity of the red channel decreased and the fluorescence intensity of the green channel increased (Fig. 7b and c). The ratio of the fluorescence intensity of the two channels (Igreen/Ired) was enhanced with the increase of the concentration of HOCl (Fig. 7f). When using LPS (lipopolysaccharide, an endotoxin that induces HOCl production) and PMA (phorbol myristate acetate) to induce cells to produce endogenous HOCl, the same fluorescence phenomenon as that in the case of adding exogenous HOCl was observed. In order to avoid the interference of other factors, we adopted NAC (acetylcysteine, a scavenger of HOCl) to remove the generated HOCl. We found that the fluorescence intensities of the red and green channel of MCF-7 cells did not change much after treatment with NAC compared to the fluorescence intensities of cells incubated with HBTN alone, indicating that the probe HBTN can respond to endogenous HOCl (Fig. 7a and e). Hence, the probe HBTN can realize the monitoring of exogenous and endogenous HOCl in mitochondria.
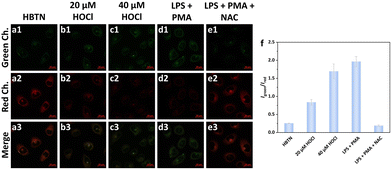 |
| Fig. 7 Fluorescence imaging of mitochondrial HOCl in MCF-7 cells. (a) MCF-7 cells were incubated with HBTN (10 μM) for 30 min. (b and c) MCF-7 cells were first incubated with the probe HBTN (10 μM) for 30 min, and then treated with different concentrations of HOCl (20 and 40 μM) for 30 min. (d and e) MCF-7 cells were first treated with LPS (5 μg mL−1) + PMA (5 μg mL−1) or LPS (5 μg mL−1) + PMA (5 μg mL−1) + NAC (1 mM) for 3 h, and then incubated with the probe HBTN (10 μM) for 30 min. λex = 405 nm, green channel: λem = 450–530 nm, red channel: λem = 680–740 nm, scale bar = 20 μm. (f) Comparison of the fluorescence intensity ratios of the green and red channels. | |
To further explore the imaging performance of the probe HBTN in vivo, we performed experiments using zebrafish as a model. We can find from Fig. 8 that the fluorescence intensity of the red channel of zebrafish treated with nystatin was stronger than that of zebrafish not treated with nystatin. The above result demonstrates that the probe HBTN can be used to monitor viscosity in zebrafish.
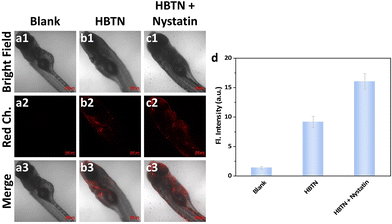 |
| Fig. 8 Fluorescence imaging of viscosity in zebrafish. (a) Zebrafish without any treatment. (b) Zebrafish were incubated with HBTN (10 μM) for 30 min. (c) Zebrafish were first treated with nystatin (10 μM) for 30 min, and then incubated with probe HBTN (10 μM) for 30 min. λex = 405 nm, red channel: λem = 680–740 nm, scale bar = 200 μm. (d) Comparison of red channel fluorescence intensities. | |
Furthermore, we tried to use the probe HBTN for HOCl detection in vivo. As shown in Fig. 9, when zebrafish were treated with exogenous hypochlorous acid, it could be clearly observed that the fluorescence intensity of the red channel decreased and the fluorescence intensity of the green channel enhanced. On the other hand, we treated zebrafish with LPS to generate endogenous HOCl. The fluorescence in the red channel was decreased and the fluorescence in the green channel was enhanced in LPS-treated zebrafish. However, zebrafish did not show the above fluorescence changes when NAC was used to remove HOCl. Therefore, the probe HBTN enables the monitoring of endogenous and exogenous HOCl in zebrafish.
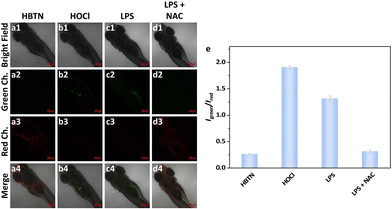 |
| Fig. 9 Fluorescence imaging of HOCl in zebrafish. (a) Zebrafish were incubated with HBTN (10 μM) for 30 min. (b–d) Zebrafish were first treated with HOCl (20 μM) for 30 min, LPS (5 μg mL−1) for 4 h or LPS (5 μg mL−1) + NAC (1 mM) for 4 h, and then incubated with the probe HBTN (10 μM) for 30 min. λex = 405 nm, green channel: λem = 450–530 nm, red channel: λem = 680–740 nm, scale bar = 200 μm. (e) Comparison of the fluorescence intensity ratios of the green and red channels. | |
4. Conclusions
In summary, we constructed a novel mitochondria-targeted fluorescent probe HBTN for the detection of viscosity and HOCl in vitro and in vivo. HBTN has superior properties such as long wavelength emission and large Stokes shift. Probe HBTN can quantitatively detect viscosity and HOCl with high selectivity and sensitivity. Colocalization experiments confirmed that the probe HBTN can localize in the subcellular organelle mitochondria. Furthermore, the probe HBTN was able to detect viscosity and exogenous/endogenous HOCl in living cells and zebrafish. The excellent performance of HBTN leads us to believe that it can be an effective tool to detect viscosity and HOCl in vivo in the future, which will facilitate further studies on the physiological significance of viscosity and HOCl.
Author contributions
Wangbo Qu: Conceptualization, methodology, software, writing – original draft; Bin Yang: Software, validation; Taiyu Guo: Data curation, investigation; Ruowei Tian: Software, formal analysis; Shuang Qiu: Investigation, visualization; Xinyue Chen: Visualization, formal analysis; Zhirong Geng: Supervision, resources, writing – review and editing; Zhilin Wang: Project administration, resources, funding acquisition, writing – review and editing.
Conflicts of interest
There are no conflicts to declare.
Acknowledgements
This work was supported by the National Natural Science Foundation of China (21976080).
References
- S. E. Weinberg and N. S. Chandel, Nat. Chem. Biol., 2015, 11, 9–15 CrossRef CAS.
- S. Nesci, Mitochondrion, 2017, 35, 11–12 CrossRef CAS.
- S. Vyas, E. Zaganjor and M. C. Haigis, Cell, 2016, 166, 555–566 CrossRef CAS PubMed.
- N. Pfanner, B. Warscheid and N. Wiedemann, Nat. Rev. Mol. Cell Biol., 2019, 20, 267–284 CrossRef CAS.
- K. Luby-Phelps, Curr. Opin. Cell Biol., 1994, 6, 3–9 CrossRef CAS PubMed.
- S. Raha and B. H. Robinson, Trends Biochem. Sci., 2000, 25, 502–508 CrossRef CAS.
- P. S. Brookes, Y. S. Yoon, J. L. Robotham, M. W. Anders and S. S. Sheu, Am. J. Physiol.: Cell Physiol., 2004, 287, C817–C833 CrossRef CAS PubMed.
- K. Luby-Phelps, Int. Rev. Cytol., 1999, 192, 189–221 Search PubMed.
- Z. G. Yang, J. F. Cao, Y. X. He, J. H. Yang, T. Kim, X. J. Peng and J. S. Kim, Chem. Soc. Rev., 2014, 43, 4563–4601 RSC.
- K. Luby-Phelps, Mol. Biol. Cell, 2013, 24, 2593–2596 CrossRef CAS PubMed.
- J. L. Yin, M. Peng and W. Y. Lin, Anal. Chem., 2019, 91, 8415–8421 CrossRef CAS.
- M. Castellana, M. Z. Wilson, Y. F. Xu, P. Joshi, I. M. Cristea, J. D. Rabinowitz, Z. Gitai and N. S. Wingreen, Nat. Biotechnol., 2014, 32, 1011–1018 CrossRef CAS PubMed.
- Y. C. Miao, S. Bhattacharya, M. Edwards, H. Q. Cai, T. Inoue, P. A. Iglesias and P. N. Devreotes, Nat. Cell Biol., 2017, 19, 329–340 CrossRef CAS PubMed.
- D. C. Wallace, Nat. Rev. Cancer, 2012, 12, 685–698 CrossRef CAS.
- M. Morohoshi, K. Fujisawa, I. Uchimura and F. Numano, Diabetes, 1996, 45, 954–959 CrossRef PubMed.
- K. Eto, T. Asada, K. Arima, T. Makifuchi and H. Kimura, Biochem. Biophys. Res. Commun., 2002, 293, 1485–1488 CrossRef CAS.
- A. M. Aleardi, G. Benard, O. Augereau, M. Malgat, J. C. Talbot, J. P. Mazat, T. Letellier, J. Dachary-Prigent, G. C. Solaini and R. Rossignol, J. Bioenerg. Biomembr., 2005, 37, 207–225 CrossRef CAS.
- P. Mecocci, M. F. Beal, R. Cecchetti, M. C. Polidori, A. Cherubini, F. Chionne, L. Avellini, G. Romano and U. Senin, Mol. Chem. Neuropathol., 1997, 31, 53–64 CrossRef CAS.
- Y. Y. Zhang, Z. Li, W. Hu and Z. H. Liu, Anal. Chem., 2019, 91, 10302–10309 CrossRef CAS PubMed.
- M. D. Rees, S. E. Bottle, K. E. Fairfull-Smith, E. Malle, J. M. Whitelock and M. J. Davies, Biochem. J., 2009, 421, 79–86 CrossRef CAS PubMed.
- M. B. Hampton, A. J. Kettle and C. C. Winterbourn, Blood, 1998, 92, 3007–3017 CrossRef CAS.
- A. Hammer, G. Desoye, G. Dohr, W. G. Sattler and E. Malle, Lab. Invest., 2001, 81, 543–554 CrossRef CAS PubMed.
- N. H. Lu, Y. H. Sui, R. Tian and Y. Y. Peng, J. Agric. Food Chem., 2018, 66, 4933–4940 CrossRef CAS PubMed.
- J. C. Genereux, A. K. Boal and J. K. Barton, J. Am. Chem. Soc., 2010, 132, 891–905 CrossRef CAS.
- S. J. Klebanoff, J. Leukocyte Biol., 2005, 77, 598–625 CrossRef CAS.
- S. Sultana, A. Foti and J. U. Dahl, Infect. Immun., 2020, 88, e00964–e00919 CrossRef.
- C. Liu, Y. Shang, T. Zhao, L. J. Liang, S. He, L. C. Zhao, X. S. Zeng and T. H. Wang, Sens. Actuators, B, 2021, 348, 130632 CrossRef CAS.
- M. J. Steinbeck, L. J. Nesti, P. F. Sharkey and J. Parvizi, J. Orthop. Res., 2007, 25, 1128–1135 CrossRef CAS PubMed.
- A. Daugherty, J. L. Dunn, D. L. Rateri and J. W. Heinecke, J. Clin. Invest., 1994, 94, 437–444 CrossRef CAS.
- S. Waldbaum, L. P. Liang and M. Patel, J. Neurochem., 2010, 115, 1172–1182 CrossRef CAS PubMed.
- S. M. Wu and S. V. Pizzo, Arch. Biochem. Biophys., 2001, 391, 119–126 CrossRef CAS PubMed.
- E. A. Podrez, H. M. Abu-Soud and S. L. Hazen, Free Radical Biol. Med., 2000, 28, 1717–1725 CrossRef CAS.
- D. I. Pattison and M. J. Davies, Biochemistry, 2006, 45, 8152–8162 CrossRef CAS PubMed.
- X. H. Huang, T. C. Luo, C. Zhang, J. R. Li, Z. J. Jia, X. L. Chen, Y. J. Hu and H. Huang, Talanta, 2022, 241, 123235 CrossRef CAS PubMed.
- M. He, M. T. Ye, Z. G. Wang, P. Liu, H. Y. Li, C. F. Lu, Y. Y. Wang, T. Liang, H. Y. Li and C. Y. Li, Sens. Actuators, B, 2021, 343, 130063 CrossRef CAS.
- W. Li, Y. Shen, X. Y. Gong, X. B. Zhang and L. Yuan, Anal. Chem., 2021, 93, 16673–16682 CrossRef CAS.
- P. P. Lu, Y. Huang, C. Y. Zhang, L. L. Fu, X. Y. Wang and L. X. Chen, Talanta, 2022, 239, 123111 CrossRef CAS PubMed.
- Y. Jia, Y. Y. Wang, Y. L. Zhang, J. Y. Wang, Y. X. Pei, Z. Q. Wang, P. Li and K. L. Han, Anal. Chem., 2022, 94, 1203–1210 CrossRef CAS PubMed.
- S. Wang, Y. C. Bu, X. Lu, D. D. Chen, Z. P. Yu, J. Zhang, L. K. Wang and H. P. Zhou, Sens. Actuators, B, 2022, 371, 132546 CrossRef CAS.
- C. F. Lai, Y. P. Zhao, Y. Liang, X. Zou and W. Y. Lin, Spectrochim. Acta, Part A, 2022, 268, 120637 CrossRef CAS PubMed.
- M. Oguz, S. Erdemir and S. Malkondu, Anal. Chim. Acta, 2022, 1227, 340320 CrossRef CAS PubMed.
- R. Q. Zhou, L. X. Niu, Y. F. Hu, Q. R. Qi, W. C. Huang and L. Yang, Spectrochim. Acta, Part A, 2021, 248, 119226 CrossRef CAS PubMed.
- J. T. Zhan, C. Geng, X. Y. Hao, W. H. Song, Z. H. Li and W. Y. Lin, Sens. Actuators, B, 2022, 371, 132506 CrossRef CAS.
- S. Q. Zhang, Y. Zhang, L. H. Zhao, L. L. Xu, H. Han, Y. B. Huang, Q. Fei, Y. Sun, P. Y. Ma and D. Q. Song, Talanta, 2021, 233, 122592 CrossRef CAS PubMed.
- C. Geng, J. T. Zhan, X. Y. Hao, W. H. Song and W. Y. Lin, Spectrochim. Acta, Part A, 2022, 264, 120271 CrossRef CAS PubMed.
- Y. F. Huang, Y. B. Zhang, F. J. Huo, Y. M. Liu and C. X. Yin, Dyes Pigm., 2020, 179, 108387 CrossRef CAS.
- J. L. Han, S. Yang, B. H. Wang and X. Z. Song, Anal. Chem., 2021, 93, 5194–5200 CrossRef CAS.
- A. S. Zheng, H. Liu, C. H. Peng, X. N. Gao, K. H. Xu and B. Tang, Talanta, 2021, 226, 122152 CrossRef CAS PubMed.
- M. Baruah, A. Jana, M. Ali, K. Mapa and A. Samanta, J. Mater. Chem. B, 2022, 10, 2230–2237 RSC.
- J. T. Hou, N. Kwon, S. Wang, B. Y. Wang, X. J. He, J. Yoon and J. L. Shen, Coord. Chem. Rev., 2022, 450, 214232 CrossRef CAS.
- X. Wang, F. L. Song and X. J. Peng, Dyes Pigm., 2016, 125, 89–94 CrossRef CAS.
- L. J. Liang, Y. M. Sun, C. Liu, X. J. Jiao, Y. Shang, X. S. Zeng, L. C. Zhao and J. L. Zhao, J. Mol. Struct., 2021, 1227, 129523 CrossRef CAS.
- H. Y. Li, Y. Liu, X. Y. Li, X. H. Li and H. M. Ma, Spectrochim. Acta, Part A, 2021, 246, 119059 CrossRef CAS.
- Z. X. Zhan, Q. Lei, Y. C. Dai, D. N. Wang, Q. W. Yu, Y. Lv and W. M. Li, Anal. Chem., 2022, 94, 12144–12151 CrossRef CAS PubMed.
- J. Ouyang, L. H. Sun, Z. Zeng, C. Zeng, F. Zeng and S. Z. Wu, Angew. Chem., Int. Ed., 2020, 59, 10111–10121 CrossRef CAS.
- X. Z. Luo, R. Wang, C. Z. Lv, G. Chen, J. M. You and F. B. Yu, Anal. Chem., 2020, 92, 1589–1597 CrossRef CAS PubMed.
- K. Y. Zhou, Y. T. Yang, T. T. Zhou, M. Jin and C. X. Yin, Dyes Pigm., 2021, 185, 108901 CrossRef CAS.
- L. Yan, Q. S. Gu, W. L. Jiang, M. Tan, Z. K. Tan, G. J. Mao, F. Xu and C. Y. Li, Anal. Chem., 2022, 94, 5514–5520 CrossRef CAS PubMed.
- F. Zhang, J. L. Han, J. P. Wang, X. Li, Y. G. Wang, B. H. Wang and X. Z. Song, Spectrochim. Acta, Part A, 2020, 242, 118755 CrossRef CAS PubMed.
- X. Z. Wei, M. J. Hao, X. X. Hu, Z. L. Song, Y. Wang, R. H. Sun, J. Zhang, M. Yan, B. Y. Ding and J. H. Yu, Sens. Actuators, B, 2021, 326, 128849 CrossRef CAS.
- F. Weigend and R. Ahlrichs, Phys. Chem. Chem. Phys., 2005, 7, 3297–3305 RSC.
- F. Weigend, Phys. Chem. Chem. Phys., 2006, 8, 1057–1065 RSC.
- A. Hellweg, C. Hättig, S. Höfener and W. Klopper, Theor. Chem. Acc., 2007, 117, 587–597 Search PubMed.
- T. Lu and F. W. Chen, J. Comput. Chem., 2012, 33, 580–592 CrossRef CAS PubMed.
- Y. H. Chen, T. W. Wei, Z. J. Zhang, W. Zhang, J. Lv, T. T. Chen, B. Chi, F. Wang and X. Q. Chen, Chin. Chem. Lett., 2017, 28, 1957–1960 CrossRef CAS.
- M. Z. Tang, Z. Huang, X. L. Luo, M. Q. Liu, L. Z. Wang, Z. H. Qi, S. F. Huang, J. C. Zhong, J. X. Chen, L. F. Li, D. Wu and L. X. Chen, Free Radical Biol. Med., 2019, 134, 445–457 CrossRef CAS PubMed.
|
This journal is © The Royal Society of Chemistry 2023 |
Click here to see how this site uses Cookies. View our privacy policy here.