DOI:
10.1039/D2BM01263D
(Paper)
Biomater. Sci., 2023,
11, 307-321
Calcofluor white-cholesteryl hydrogen succinate conjugate mediated liposomes for enhanced targeted delivery of voriconazole into Candida albicans†
Received
8th August 2022
, Accepted 10th November 2022
First published on 30th November 2022
Abstract
Fungal infections gradually lead to a high mortality rate due to difficulties in diagnosis, the limited number of antifungal drugs available, and the appearance of resistant isolates. Here, we developed a calcofluor white-cholesteryl hydrogen succinate conjugate (CFW–CHSc) as a novel nanomaterial that specifically binds to chitin chains in the cell wall. We showed that fluorescent-dye loaded CFW–CHSc-liposomes entered the cytoplasm of Candida albicans cells with increased efficacy. Voriconazole-loaded CFW–CHSc-liposomes displayed an increased antifungal activity against C. albicans yeast cells in an in vitro assay. Animal infection models and animal imaging analysis showed that fluorescent-dye loaded CFW–CHSc-liposomes maintained prolonged residence in rodent tissues. In mouse liver and kidney tissue, voriconazole-loaded CFW–CHSc-liposomes showed significantly enhanced antifungal activity when administered intravenously. Taken together, our studies confirm that CFW–CHSc increases the drug delivery efficacy of nanoparticles in vitro by interacting with chitin chains in the C. albicans cell wall. The fungi-targeting nanoparticles improve the drug delivery efficacy in vivo by enriching the nanoparticles at the site of fungal infection via the blood circulation system. Fungi-targeting nanomaterials have a promising future in the treatment of nosomycosis.
Introduction
COVID-19 can cause acute respiratory distress syndrome (ARDS) and increase the incidence of fungal super-infections in critically ill patients.1 Fungi are increasingly considered the cause of superficial, invasive, and disseminated infections. There are difficulties in diagnosing fungal infections and a limited number of antifungal drugs. There are also resistant isolates leading to challenging treatment and high mortality rates.2,3Candida is a yeast that colonizes the surface epithelium of the alimentary canal and the urogenital system of healthy human beings as normal flora.4 An impaired local or systemic immune system can cause candida overgrowth leading to candida infection such as candidiasis and candidemia. Candida albicans is the most commonly identified Candida species in clinical contexts leading to approximately 50% of all cases of candidiasis with an overall mortality rate of 43%.5
A remarkable feature of C. albicans is that it can grow either as a unicellular budding yeast or in filamentous pseudohyphal and hyphal forms by regulation of morphogenesis in response to a host environment. Switching from yeast to hyphal growth is highly affected by its virulence.6 In Candida infections, pathogenic yeast cells can adhere to host surfaces and medical devices, and thus form biofilms containing polysaccharide matrix-enclosed microcolonies of yeasts and hyphae arranged in a bilayer structure.7 Biofilms can protect the pathogen from host defenses and antibiotics, and sequester conventional antifungal agents. C. albicans cells in biofilms are more resistant to the current antifungal drugs versus cells under non-biofilm conditions (planktonic cells).8 However, long-term administration of antifungal drugs can also cause serious side effects such as hepatotoxicity, hormone-related effects and kidney damage.9,10 Multidrug-resistant Candida species such as Candida auris and Candida glabrata cause therapeutic failures.11 Therefore, new antifungal drugs with novel mechanisms of action are urgently needed as new drug delivery vehicles for improved efficacy.
Fungal cell walls have a versatile role in cell viability, morphogenesis, and pathogenesis. As a dynamic organelle, its composition is precisely regulated in response to environmental stresses and growth conditions.12 Most fungal cell walls contain an inner layer and outer layer. The skeletal structure in the inner layer is relatively conserved in fungi whereas the heterogeneous outer layer is various.13 In C. albicans, the inner layer is composed of β-(1,3) glucan with 3 to 4% interchain and chitin. The formation of β-(1,3) glucan and chitin by hydrogen bonds can assemble into fibrous microfibrils that form a basket-like scaffold around fungal cell.14 The outer layer coats the inner layer with highly mannosylated glycoproteins. β-(1,6) glucan as a linker molecule connects cell wall proteins to the β-(1,3) glucan-chitin skeleton by a glycosylphosphatidyl inositol (GPI) remnant.15 These structural elements on the fungal cell wall not only provide mechanical support to maintain cell shape but also protect cells from degradative enzymes in the environment and serve as adhesins to anchor the cell to the substrate.16 Moreover, rearrangement in the C. albicans cell wall produces elliptical and tubular shapes, greatly increasing C. albicans environmental adaption and host colonization.17,18
Calcofluor white (CFW) is widely used for the detection of many yeasts, pathogenic fungi (such as C. albicans), mycelial fungi (such as Aspergillus niger, Aspergillus nidulans, and Aspergillus awamori) and the basidiomycetous fungus Cryptococcus neoformans due to its specific binding with fungi.19–22 There are two sulfonic-acid groups in its chemical structure. CFW is solubilized due to its two negatively charged sulfonic-acid groups under certain conditions such as slight acid.23 CFW binds with many kinds of β-linked glucans in vitro24 and binds intensely with nascent chitin chains in vivo in Poterioochromonas stipitata.25 CFW also shows antifungal activity against fungi. Chitin assembly is interrupted by competition for hydrogen bonding sites between CFW and natural chitin. As a result, the integrity of the fungal cell wall is compromised and fungal growth is inhibited.26–29
In this study (Scheme 1), we designed and synthesized a CFW–cholesteryl hydrogen succinate conjugate (CFW–CHSc) as an antifungal nanomaterial. The structure of CFW–CHSc was determined using a nuclear magnetic resonance spectrometer. The mechanism by which CFW–CHSc interacts with its substrates is determined. The distribution rate of CFW–CHSc–Cy5.5-liposomes in a mouse is characterized by small animal imaging. Fungi-targeting nanodrug delivery systems based on CFW–CHSc were prepared by constructing voriconazole-loaded CFW–CHSc liposomes (CFW–CHSc–VRC-liposomes). Physicochemical characteristics such as particle size, ζ-potential, and the entrapment efficiency of CFW–CHSc–VRC-liposomes were also investigated. The minimum inhibitory concentration (MIC) was used to characterize the antifungal activity of CFW–CHSc, CFW–CHSc–VRC-liposomes, and voriconazole-loaded liposomes (VRC-liposomes) against C. albicans yeast cells in vitro. The drug delivery efficacy of CFW–CHSc-liposomes was determined with VRC-liposomes in vivo and in vivo. The antifungal activity of CFW–CHSc–VRC-liposomes and VRC-liposomes was then determined in vivo. Our data demonstrate that this novel biomaterial CFW–CHSc is active against C. albicans and binds specifically with cell walls in C. albicans. The drug delivery efficacy of fungi-targeting nanodrug delivery systems is dramatically improved versus untargeted liposomes in vitro and vivo. CFW–CHSc–VRC-liposomes greatly reduce the fungal burden in the mouse liver and kidneys suggesting excellent prospects in the treatment of fungal peritonitis in mice by CFW–CHSc.
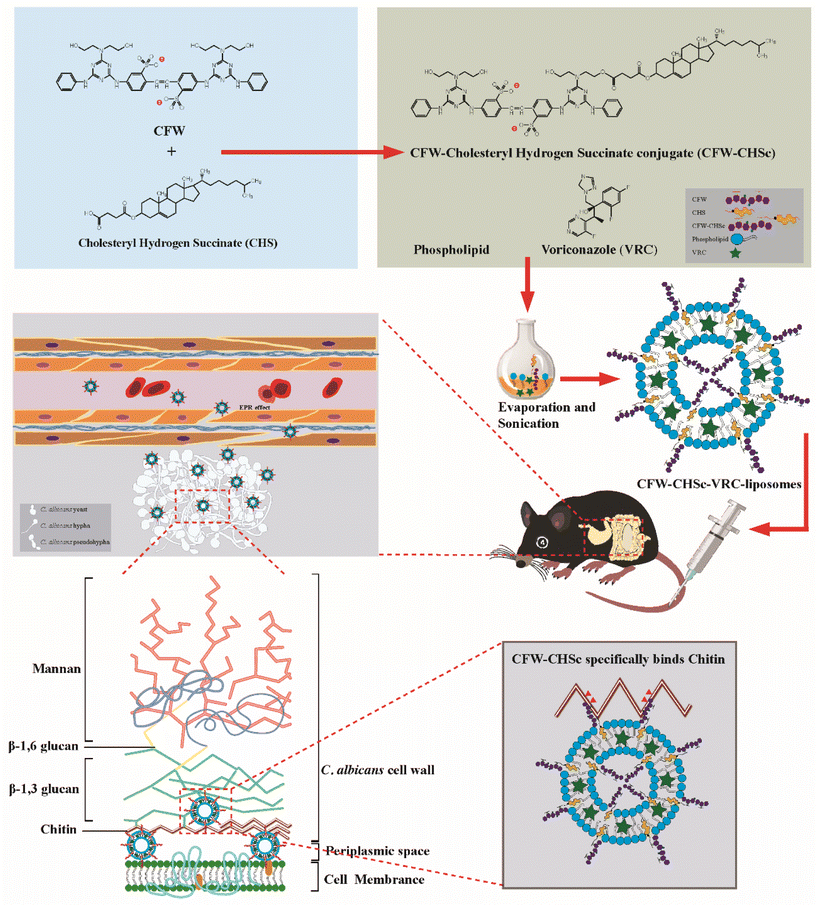 |
| Scheme 1 Schematic illustration of CFW–CHSc–VRC-liposomes in the treatment of fungal peritonitis in mice. CFW–CHSc is synthesized from CHS and CFW. VRC-loaded CFW–CHSc-liposomes are prepared and injected intravenously into infected mice. C. albicans's morphological development promotes biofilm growth at the infection sites where the extracellular matrix has a looser structure composed of glucans, chitin, and other polymers. The specific targeting ability of CFW–CHSc to chitin chains in the C. albicans cell wall promotes the enrichment of VRC-loaded nanoparticles at the infected sites via blood circulation. | |
Methods
Materials
CFW, N-(3-dimethylaminopropyl)-N′-ethylcarbodiimide hydrochloride, crystalline, (EDC·HCl), cholesteryl hydrogen succinate (CHS), N-hydroxysuccinimide (NHS), voriconazole (VRC), N-acetylglucosamine, polyxomycin D, coumarin 6 (C6), cyanine 5.5 (Cy5.5) and phosphate buffered saline (PBS) were all purchased from Sigma-Aldrich (St Louis, MO, USA). Anhydrous dimethyl sulfoxide (DMSO) was purchased from Aladdin (Shanghai, China). High-performance liquid chromatography (HPLC)-grade acetonitrile was purchased from DikmaPure (Beijing, China). Purified water was used throughout the experiment and was produced with a Merck Millipore Ultrapure Water System (Darmstadt, Germany). All other chemicals and reagents were of analytical grade quality or higher.
Strains and media
The strain used here was the sequenced reference strain C. albicans SC5314. C. albicans was cultured on yeast peptone dextrose (YPD) plates (1% yeast extract, 2% peptone, 2% dextrose, and 2% agar) or broth (1% yeast extract, 2% peptone, and 2% dextrose). A standard cell suspension was prepared by inoculating a single colony into YPD broth and incubating overnight at 200 rpm agitation and 30 °C. For antifungal activity testing and cellular uptake efficacy testing in vitro, the C. albicans cells were harvested using centrifugation, washed twice with PBS (0.1 M, pH 7.4), and re-suspended in YPD media at 1 × 105 CFU mL−1. In the animal infection model, C. albicans cells were re-suspended in YPD media at 1 × 108 CFU mL−1.
Preparation of CFW–CHSc–VRC-liposomes and VRC-liposomes
CFW–CHSc–VRC-liposomes were prepared using an ethanol injection method. Briefly, phospholipids, cholesterol, and VRC were dissolved in absolute ethanol. CFW–CHSc liposomes (from 1 μM to 0.01 μM) were solubilized in DMSO followed by dropping them into the above ethanol solution. The mixture was then added dropwise into deionized water at room temperature. This solution was sonicated with a Scientz-II D probe sonicator (Ningbo Scientz Biotechnology Co., Ltd, Zhejiang, China) at 150 W for 2 min in an ice bath. Finally, the sample was dialyzed (MWCO 8–10 kDa) against deionized water for two days to remove organic solvent and free VRC. VRC-liposomes were prepared as described above without the addition of CFW–CHSc.
Characterization of CFW–CHSc–VRC-liposomes and VRC-liposomes
The particle size and zeta potential of both nanoparticles were determined by dynamic light scattering (DLS) on a Zetasizer Nano-ZS ZEN3600 (Malvern Instruments Ltd, Worcestershire, UK). The measurements were performed at 25 °C after diluting the nanoparticles with deionized water. The entrapment efficiency (EE) and drug loading capacity (DL) of the VRC were determined by indirect calculations: 1 mL of liposomes was centrifuged using an ultrafiltration tube (Millipore, USA, MWCO 10 kDa) for 10 min to separate the free drug (Wfree) and encapsulated VRC (W). The filtrate was collected and determined for free VRC content by HPLC. For this, a C18 reversed-phase column (4.6 × 250 mm internal diameter, 5 μm) was used, with methanol
:
0.1% acetic acid = 70
:
30 as the mobile phase at a flow rate of 1.0 mL min−1, detection at 256 nm, the column temperature was 30 °C, and the volume of injection was 20 μL. The total VRC (Wtotal) in liposomes was obtained by mixing 900 μL of methanol with 100 μL of nanoparticles, thus destroying the nanoparticles and releasing all VRC. The EE (%) and DL (%) were calculated according to the following formulas. | 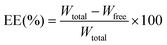 | (1) |
| 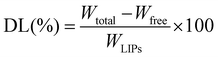 | (2) |
CFW–CHSc synthesis
CFW–CHSc was synthesized by a previously described method using EDC·HCl and NHS as catalysts. In brief, EDC·HCl (0.1 mM) and NHS (0.2 mM) were dissolved in 1 mL DMSO separately and were added in sequence to a round-bottom flask with CHS in 1 mL DMSO. The carboxylic acid moieties of CHS were activated for 2 h at 45 °C followed by adding 0.1 mM CFW in 1 mL DMSO. The mixture was then stirred at 45 °C for 12 h and dialyzed (dialysis membrane's Mw cutoff was 3.5 kDa) against deionized water for two days in the dark at room temperature to remove the unreacted components. The resulting solution was centrifuged at 5000 rpm for 10 min and a white powder was observed through lyophilization of the supernatants. The 1H nuclear magnetic resonance (NMR) spectrum of CHS in CDCl3 and CFW–CHSc in DMSO was determined using a NMR spectrometer (BrukerAM-400). 1H NMR spectra were recorded at 400 MHz in DMSO-d6 with TMS as an internal standard. 13C NMR spectra were recorded on a 100 MHz spectrometer. Chemical shifts (δ) were reported in parts per million (ppm) relative to the residual solvent DMSO-d6 signal at 2.50 ppm for 1H NMR and at 39.52 ppm for 13C NMR. Mass spectra were obtained using a QTOF-HRMS spectrometer using electron spray ionization.
CFW–CHSc binding test in C. albicans
To determine the binding ability of CFW–CHSc, C. albicans cells were grown in YPD media under various stress conditions. The cells were grown overnight in YPD media and then transferred to YPD media supplemented with different agents: 23 mM N-acetylglucosamine or 0.18 mM polyxomycin D. After 6 h, the cell suspensions were diluted to 1 × 107 CFU ml−1. The same amount of CFW–CHSc was added into YPD media, and the cells were incubated at 30 °C for 10 minutes. Finally, the cells were washed twice with PBS and re-suspended in 200 μL PBS for microscopy imaging. Fluorescence imaging was performed using a confocal laser scanning microscope (Leica TCS SPE) fitted with a 63×oil immersion objective lens. The cells were excited using UV light illuminating at less than 365 nm. Emission profiles were collected at 435 ± 10 nm.
In vitro antifungal activity
The antifungal activity of the tested compounds was determined in YPD media following the guidelines of the National Committee for Clinical Laboratory Standards. Serial dilutions of CFW–CHSc (0.1 μM)–VRC-liposomes and VRC-liposomes were performed on 0.03–16 μg mL−1. The compounds were dispensed into the wells of the first ten rows of a 96-well plate (100 μL each) using a multichannel pipette. 100 μL of YPD media containing 1 × 104 CFU C. albicans cells was added into each well. The control group contained 105 CFU mL−1C. albicans cells in 200 μL YPD media. All plates were incubated for 12 h at 30 °C with agitation at 200 rpm. For each well, the optical density at 600 nm was determined by microplate spectrophotometry (Thermo-Fisher Scientific, Waltham, MA, USA). The inhibition rate of the tested compounds was calculated as a percentage of inhibition in C. albicans cell growth compared to the control (no drug). All assays were performed in triplicate and repeated three times for reproducibility.
Cytotoxicity assay of CFW–CHSc in vitro
After trypsin digestion of 293T cells at the logarithmic growth stage, the cell density was adjusted to 5 × 104 cells per mL and the cells were inoculated into 96-well cell culture plates with 100 μL per well. The culture medium containing CFW–CHSc solution with different concentrations (0.01–0.25 μg mL−1) in each well was incubated at 37 °C for 24 h. The method of reduction of the tetrazolium salt, WST-8, was used to evaluate the cytotoxicity of CFW–CHSc in 293T cells grown in a 96-well plate. Absorbance was measured at 465 nm. Cell viability was calculated as a percentage of the vehicle control using GraphPad Prism v.9.0 (GraphPad, San Diego, CA). All assays were performed in triplicate and repeated three times for reproducibility.
Cellular uptake efficacy of CFW–CHSc-liposomes
The cellular uptake efficacy of CFW–CHSc-liposomes was determined using coumarin-based fluorescent liposomes. C6 was packed into liposomes and CFW–CHSc-liposomes forming C6-liposomes and CFW–CHSc (from 1 μM to 0.01 μM)–C6-liposomes. This was diluted with a stock solution to 0.2 μg mL−1. Next, 20 μL stock solution was loaded into all wells of a 24-well plate loaded with 1 × 105 CFU mL−1C. albicans cells in 1.98 ml YPD media. Controls contained an identical number of cells and only C6. All plates were wrapped with aluminum paper to remove light interference and were incubated at 30 °C. At three post-treatment time points (3 h, 6 h, and 9 h), 400 μL fungal cells were harvested by centrifugation, washed twice with PBS, and then re-suspended into 10 μL PBS for microscopy imaging. All samples were observed and photographed using a Leica TCS SPE. For C6 excitation, the cells were excited using UV light at less than 470 nm. Emission profiles were collected at 490 ± 10 nm. For CFW–CHSc excitation, the cells were excited using UV light illuminating at less than 365 nm. Emission profiles were collected at 435 ± 10 nm. All assays were performed in quintuplicate and repeated five times for reproducibility.
In vivo animal imaging analysis
All animal experiments following the instructions (NIH Publications No. 8023, revised 1978) were reviewed and approved by the Research Ethics Committees of Binzhou Medical University (No. 2021-301). Female Balb/c mice (4–5 weeks, 14–16 g) were purchased from Jinan Pengyue Experimental Animal Breeding Co., LTD (Jinan, Shandong, China) and used for in vivo imaging. Intraperitoneal infection was performed as described elsewhere.30 Here, fifteen mice were randomly chosen and divided evenly (n = 5) into three groups. Next, two groups of mice were anesthetized and infected with 2 × 107 fungal cells suspended in 200 μL sterile PBS via an intraperitoneal route. One group of mice was injected with 100 μL sterile PBS and used as a control uninfected model. Cy5.5-loaded liposomes (Cy5.5-liposomes) and Cy5.5-loaded CFW–CHSc (0.1 μM)-liposomes (CFW–CHSc–Cy5.5-liposomes) were prepared. At 7 days post-infection, 50 μL of CFW–CHSc–Cy5.5-liposomes or Cy5.5-liposomes were injected into the infected mice via the tail vein. CFW–CHSc–Cy5.5-liposomes were also injected into the uninfected mice. All fluorescence images were captured and analyzed using an IVIS Spectrum In Vivo Imaging System (PerkinElmer, USA) at seven post-treatment time points: 1 h, 2 h, 4 h, 6 h, 8 h, 12 h and 24 h.
In vivo antifungal activity
For the CFU test, CFW–CHSc (from 1 μM to 0.01 μM)–VRC-liposomes and VRC-liposomes were prepared and dissolved in sterile saline. Female C57BL/6 mice (4–5 weeks, 18–22 g) were purchased from Jinan Pengyue Experimental Animal Breeding Co., Ltd. Thirty-five mice were chosen randomly and divided evenly (n = 5) into seven groups. Intraperitoneal infection was performed as described elsewhere above. At one day post-infection, the infected mice were intravenously administered 2 mg kg−1 CFW–CHSc–VRC-liposomes or VRC-liposomes. The infected mice in control groups were administered CFW–CHSc-liposomes or liposomes (VRC free) at the same dose. Seven groups of mice were sacrificed at 8 days post-infection. Their liver and kidney tissues were isolated, weighed, and placed in 10 mL PBS. The tissues were then homogenized, and the suspension was plated on YPD agar at 30 °C for 2 days. The number of colony-forming units was then determined. For histopathological evaluation, the mice were treated as described above. At one day post-infection, the infected mice were intravenously administered 2 mg kg−1 CFW–CHSc (0.1 μM)–VRC-liposomes or VRC-liposomes. The liver and kidneys obtained at 8 days post-infection were stained with H&E. For the survival rate of the mice by intraperitoneal infection with C. albicans, forty mice were chosen randomly and divided evenly (n = 10) into four groups. At one day post-infection, the infected mice were intravenously administered 2 mg kg−1 CFW–CHSc (0.1 μM)–VRC-liposomes or VRC-liposomes. The survival rate of mice was recorded daily until day 20.
Image analysis
Images were compiled and analyzed using ImageJ (National Institutes of Health). In a study of the cellular uptake efficacy of CFW–CHSc-liposomes, the total intensity among cells was divided by cell area to achieve a mean gray value of the nanoparticle fluorescence intensity. In each group, five images were measured for statistical significance. In a study of in vivo animal imaging analysis, integrated density measurements of the nanoparticle fluorescence intensity were taken from the peritoneal cavity of mice by ROI analysis. All mice in each group were measured individually. The standard deviation of intensity of a monomer was found to be less than 10% of the total intensity.
Statistical analysis
Experiments were independently repeated at least three times for reproducibility. Data are expressed as the mean ± SD. Statistical analyses were performed using one-way analysis of variance followed by post hoc comparisons with Tukey's honest significant difference test (SPSS 19.0; IBM, Armonk, NY, USA). Statistically significant differences were set at P < 0.05.
Results and discussion
Characterization of the CFW–CHSc structure
The structure of the final product was determined through a NMR spectrometer and HR-MS. Fig. 1A and B show the 1H NMR spectrum of CHS in CDCl3 and the final product in DMSO separately. Compared to Fig. 1A, there are two additional sets of peaks in Fig. 1B. The signals at δ 3.6–3.7 ppm were assigned to the CH2 protons of CFW. The peaks at δ 7.0–8.0 ppm demonstrated an anomeric hydrogen of CFW. Fig. 1C displays the 13C NMR spectrum of the final product in DMSO. The signals at δ 58.9, 59.2, 121.7, and 124.5–170.5 ppm were assigned to the carbons of CFW and the signals at δ 12.1–56.6, 73.7, 122.6, 172.0, and 173.7 ppm were assigned to the carbons of CHS. In Fig. 1D, HR-MS shows the signal peak of the final product appearing at 691.3103 which corresponds to the molecular ion mass of 1384.6336 and the molecular formula of C17H92N12O13S2. These results suggest that CFW is completely linked to CHS, and CFW–CHSc was successfully synthesized.
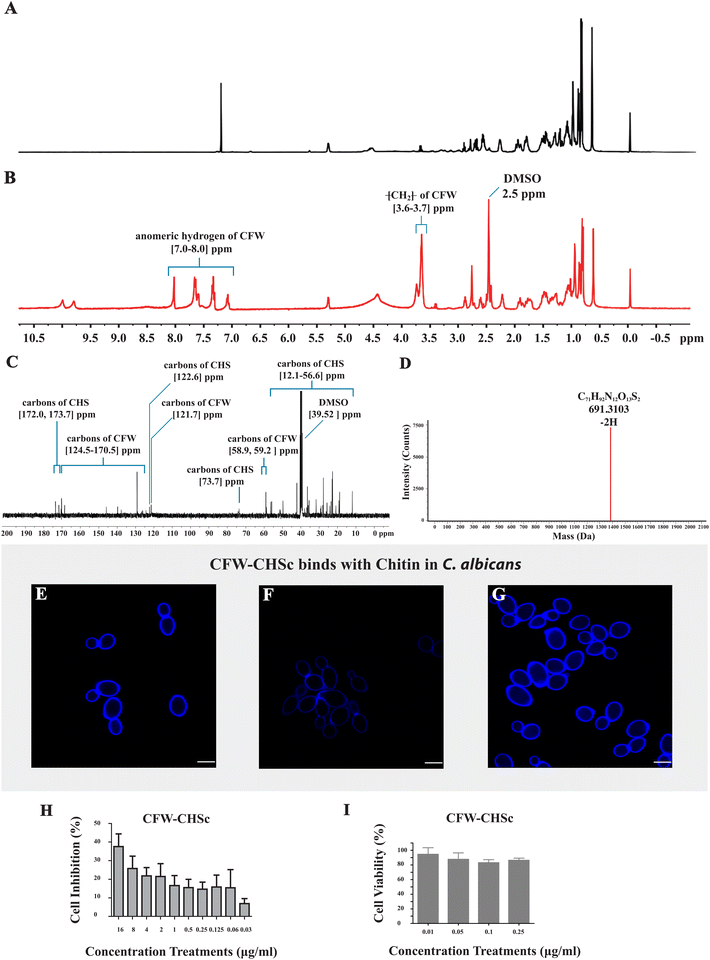 |
| Fig. 1 (A) 1H NMR spectrum of CHS in CDCl3. (B) 1H NMR spectrum of the final product from CHS and CFW as determined by 1H NMR. (C) 13C NMR spectrum of the final product. (D) Molecular ion mass of the final product was determined by HR-MS. (E–G) The C. albicans SC5314 strain was grown overnight and transferred to YPD supplemented with the different agents listed below for 6 h of culture at 30 °C. Fluorescence images of C. albicans yeast cells stained with CFW–CHSc (blue) were obtained using a CLSM (Leica TCS SPE). All scale bars are 5 μm. (E) H2O as the control, (F) 0.18 mM polyxomycin D, (G) 23 mM N-acetylglucosamine. (H) Inhibition rates of CFW–CHSc against C. albicans yeast cells in vitro. Vertical bars represent mean ± SD obtained from three experiments for each concentration. (I) Viability rates of the 293T cells treated with CFW–CHSc in vitro. Vertical bars represent mean ± SD obtained from three experiments for each concentration. | |
CFW–CHSc specifically binds to chitin in the cell walls of C. albicans
As a typically diaminostilbene derivative compound, CFW fluoresces upon exposure to UV light and binds specifically to various β-linked fibrillar polymers such as cellulose and chitin through hydrogen bonding.31 Specific binding tests of CFW–CHSc with chitin were performed to determine whether or not CFW–CHSc would mimic the ability of CFW to interact with chitin in the cell wall of fungi. C. albicans cells grown in YPD with CFW–CHSc treatment generated a typical CFW fluorescence in the cell wall (Fig. 1E). The intensity of CFW–CHSc fluorescence of the cells grown in YPD supplemented with 0.18 mM polyoxin D (Fig. 1F) decreased considerably versus control cells (Fig. 1E); cells grown in YPD supplemented with 23 mM N-acetylglucosamine (Fig. 1G) increased dramatically. Polyoxin D affects septum formation, cell wall maturation, and bud ring formation, thus damaging cell growth in fungi due to its inhibition in chitin polymerization.32 Conversely, the growth of C. albicans yeast cells on N-acetylglucosamine-supplemented media stimulates chitin synthesis causing an increased chitin content in the cell wall.33,34 Our results suggest that the intensity of CFW–CHSc fluorescence of C. albicans yeast cells is highly related to the changes in chitin synthesis. The intensity of CFW fluorescence of C. albicans yeast cells is an accurate reflection of the relative chitin content in the cell wall. Therefore, it is possible that CFW–CHSc inherits the ability of binding to chitin chains in the cell wall from CFW.
CFW–CHSc antifungal activity and cytotoxicity in vitro
An in vitro antifungal activity test was performed to characterize the antifungal activity of CFW–CHSc under normal fungal growth conditions (YPD). The results (Fig. 1H) show that the inhibition rate of CFW–CHSc is dependent on its concentration. The maximum cell inhibition rate of CFW–CHSc was 37.58% in 16 μg mL−1. A disk-diffusion assay was conducted to differentially measure the antifungal activity of CFW–CHSc against C. albicans. There was no significant difference between CFW–CHSc and CFW treatment (in 4 μg mL−1 and 16 μg mL−1 concentrations) in terms of the diameter of the inhibition zone (data not shown). In fungi, the antifungal activity of CFW is achieved from its binding to chitin chains. It is not surprising that CFW–CHSc can bind to chitin and preserve its antifungal activity even when it is being synthesized from CFW.
An in vitro assay of cytotoxicity was performed to assess the comparative safety of CFW–CHSc in mammalian cells. We compared the cell viability of the 293T cells treated with CFW–CHSc with that treated with PBS solution (Fig. 1I). Interestingly, the cell viability was approximately 95% in 293T cells treated with CFW–CHSc at 0.01 μg mL−1, whose concentration was used in the construction of targeted liposomes. These results indicated that CFW–CHSc would be safe in vitro.
Characterization of CFW–CHSc–VRC-liposomes and VRC-liposomes
Nanoparticles can reduce the unfavorable drug properties and improve drug penetration through biological membrane barriers, thus benefitting the treatment of deep fungal infections.35 Nanoparticles’ properties, such as particle size and zeta potential, are critical for adhesion to and interaction with biological cells, drug release, brain infiltration and cell-uptake efficiency.36–38 The liposomes were characterized by particle size and distribution and the zeta potential. The particle size distributions of CFW–CHSc–VRC-liposomes and VRC-liposomes are shown in Fig. 2A. The PDI of CFW–CHSc–VRC-liposomes was smaller than that of VRC-liposomes, which indicated that the particle size distribution is more centralized. Additionally, the particle sizes of VRC-liposomes and CFW–CHSc–VRC-liposomes were 74.24 ± 1.16 nm and 92.76 ± 1.23 nm, respectively. The zeta potentials of VRC-liposomes and CFW–CHSc–VRC-liposomes were −16.7 ± 0.96 mV and −8.57 ± 2.14 mV, respectively. Notably, this slightly negative zeta potential of CFW–CHSc–VRC-liposomes contributed to the steric stabilization of the nanoparticles at the particle/water interface.39 Drug EE and DL are important indices for drug delivery systems.40Fig. 2A shows that the EE (%) of VRC-liposomes was 53.67 ± 0.84%, and the DL (%) was 6.20 ± 0.061%; the EE of CFW–VRC-liposomes was 45.70 ± 0.78%, and the DL was 5.05 ± 0.058%. Moreover, the CFW–CHSc–VRC-liposomes demonstrated good stability over two weeks (ESI Table S1†).
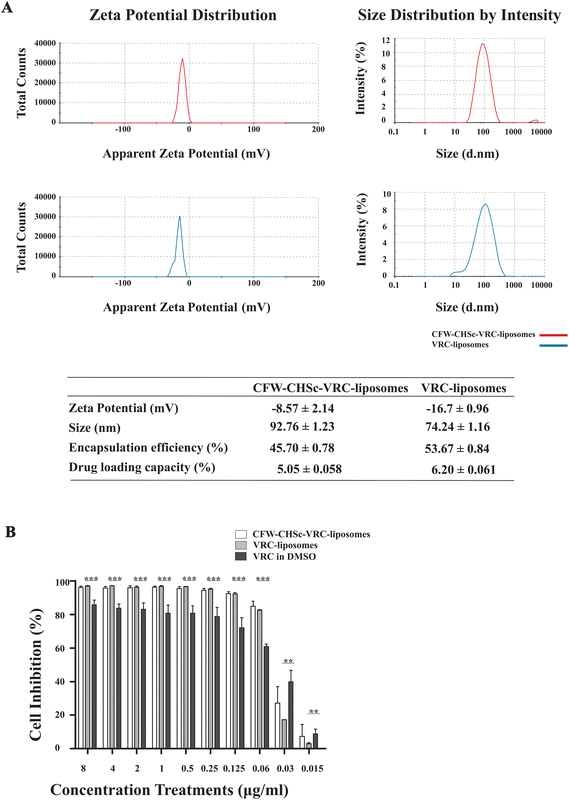 |
| Fig. 2 (A) Characterization of CFW–CHSc–VRC-liposomes (red) and VRC-liposomes (blue): ζ-potential, the particle-size distribution spectrum, and the encapsulation percentage. (B) Inhibition rates of both nanoparticles against C. albicans yeast cells in vitro. C. albicans yeast cells were incubated with different concentrations of both nanoparticles for 12 h. Values were given in reference to the control group that was cultured with CFW–CHSc-liposomes (no VRC) or liposomes (no VRC). These materials were considered to have 100% viability in the control group. Vertical bars represent mean ± SD obtained from three experiments for each concentration (***P ≤ 0.001; **P ≤ 0.01). | |
Antifungal activity of CFW–CHSc–VRC-liposomes in vitro
Next, to determine the antifungal activity of CFW–CHSc–VRC-liposomes, an in vitro antifungal activity test was performed under normal fungal growth conditions (YPD) among CFW–CHSc–VRC-liposomes, VRC-liposomes, and VRC in DMSO. The results are summarized in Fig. 2B. The inhibition rate of the three test components was dependent on the concentration. CFW–CHSc–VRC-liposomes and VRC-liposomes showed significant growth inhibition effects at concentrations greater than 0.125 μg mL−1. The cell inhibition rate was approximately 92% compared to 72% in groups treated with VRC. At drug concentrations higher than 0.06 μg mL−1, no significant difference in the cell inhibition rate was detected between CFW–CHSc–VRC-liposomes and VRC-liposomes. Interestingly, the inhibition rate of CFW–CHSc–VRC-liposomes at 0.03 μg mL−1 was approximately 1.56-fold higher than that of VRC-liposomes. The VRC-free liposomes and CFW–CHSc-liposomes negatively impacted cell growth in C. albicans (data not shown). These results indicate that liposomes might significantly improve the drug delivery efficacy by accelerating cell uptake and drug sustainability versus the free drug.41 Compared to regular liposomes, CFW–CHSc-liposomes moderately increase the antifungal activity by their targeted drug delivery in vitro.
Cellular uptake of C6-liposomes and CFW–CHSc–C6-liposomes in vitro
The photoluminescence of C6 has been widely used to study the cellular uptake of non-fluorescent drug molecules by fluorescence/confocal microscopy.42–44 To determine the effect of CFW–CHSc on the nanoparticle drug delivery efficacy, the intracellular uptake of C6-liposomes and CFW–CHSc–C6-liposomes was quantitatively examined in C. albicans yeast cells by confocal microscopy. Fig. 3A–D show the confocal micrographs of C6 loaded liposome uptake into C. albicans. The maximum fluorescence intensity of C6-liposome uptake into C. albicans was observed at 6 h. Fig. 3A–C show the role of the amount of CFW–CHSc (from 1 μM to 0.01 μM) in CFW–CHSc-liposome uptake into C. albicans yeast cells. The maximum fluorescence intensity of three distinctive CFW–CHSc–C6-liposomes was observed at 9 h. However, the fluorescence intensity of CFW–CHSc (0.01 μM)–C6-liposomes was obviously higher than that of the other CFW–CHSc–C6-liposomes at 6 h. Besides, the fluorescence intensity of CFW–CHSc (1 μM) was higher on the cell surface at 6 h whereas that of CFW–CHSc (0.1 μM) and CFW–CHSc (0.01 μM) was more in the cytoplasm (Fig. 3E). These results suggest that the uptake rate of nanoparticles varies from CFW–CHSc–C6-liposomes to C6-liposomes. The content of CFW–CHSc obviously affects the uptake of liposomes into C. albicans. CFW–CHSc might prolong the nanoparticle uptake into C. albicans cells via ATP-dependent endocytosis, due to the positive interaction between CFW–CHSc and chitin in the cell wall. The content of CFW–CHSc in nanoparticles possibly correlates interaction strengths with its substrate.
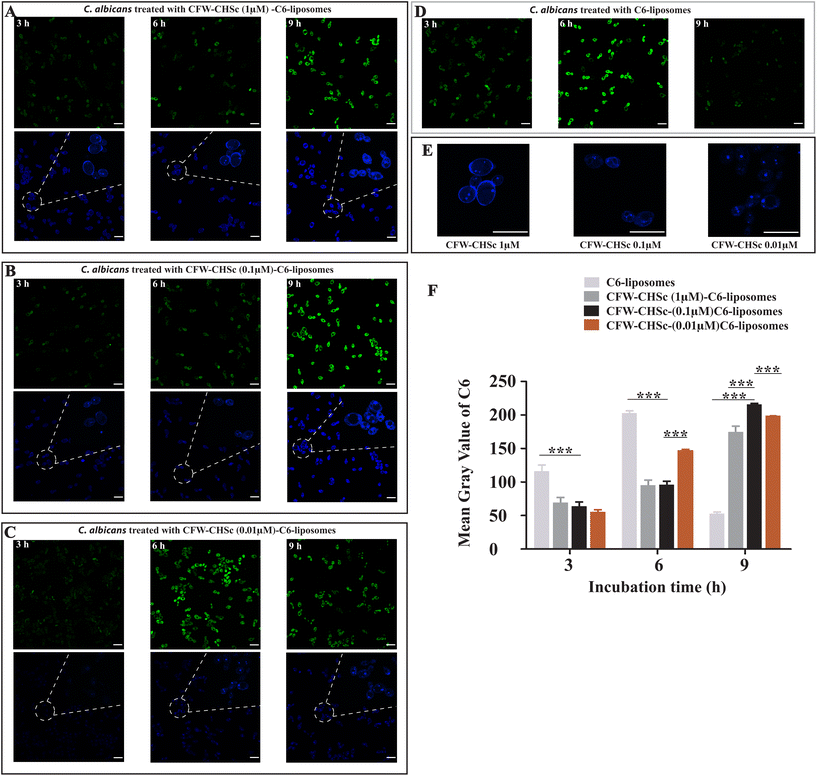 |
| Fig. 3 (A–D) The C. albicans SC5314 strain was incubated with CFW–CHSc (from 1 μM to 0.01 μM)–C6-liposomes or C6-liposomes for 3 h, 6 h, and 9 h. Fluorescence images of C. albicans yeast cells were obtained using a Leica TCS SPE. The green fluorescent channel was from C6 excitation and the blue fluorescent channel was from CFW–CHSc excitation. All scale bars are 10 μm. (E) The magnification of interaction between CFW–CHSc–C6-liposomes and C. albicans in the blue fluorescent channel at 6 h. (F) Quantitative measurements of both fluorescent nanoparticles in C. albicans yeast cells using ImageJ at three post-treatment time points (3 h, 6 h, and 9 h). Vertical bars represent mean ± SD obtained from three experiments for each post-treatment time point (***P ≤ 0.001). | |
Furthermore, the fluorescence signals in the cellular uptake of dye-loaded nanoparticles were quantified as the mean gray value using ImageJ (Fig. 3F). We found that the mean gray value in the cellular uptake of CFW–CHSc (0.1 μM)–C6-liposomes at 9 h was approximately 4-fold higher than the value of C6-liposomes at 9 h. This value was also slightly higher than the maximum mean gray value in the cellular uptake of C6-liposomes at 6 h. The higher mean gray value of CFW–CHSc–C6-liposomes versus C6-liposomes could be explained on the basis of the increased cellular accumulation of the encapsulated dyes. The target nanoparticle formulation (CFW–CHSc-liposomes) might act as an intracellular depository to maintain sustained dye release. Meanwhile, the mean gray value in the cellular uptake of CFW–CHSc (0.1 μM)–C6-liposomes at 9 h was significantly higher than that in the cellular uptake of other CFW–CHSc–C6-liposomes. These results indicate that 0.1 μM CFW–CHSc might increase the cellular uptake of CFW–CHSc–C6-liposomes into C. albicans yeast cells, compared with 1 μM and 0.01 μM CFW–CHSc. Therefore, the quantity of CFW–CHSc in targeted liposome construction was 0.1 μM in this study. Compared to traditional liposomes, our results show the improved drug delivery efficacy of CFW–CHSc-liposomes in vitro due to their greater intracellular uptake in C. albicans yeast cells.
Targeted in vivo fluorescence imaging
Cyanine-based systems provide important information required for biomedical research in living animal imaging due to their attractive optical properties with appropriate bio-functionality.45–47 Cy5.5-liposomes and CFW–CHSc (0.1 μM)–Cy5.5-liposomes were prepared to determine the accumulation behavior of CFW–CHSc-liposomes in vivo. An intraperitoneal infection model was structured in Balb/c mice infected with C. albicans yeast cells. A live animal imaging assay was then performed to monitor the fluorescence signals of CFW–CHSc–Cy5.5-liposomes and Cy5.5-liposomes injected intravenously in real-time. Infected mice treated with CFW–CHSc–Cy5.5-liposomes exhibited steady and saturated signals in the peritoneal cavity after 1 h (Fig. 4B). The fluorescence signal declined with time and remained moderate in the peritoneal cavity after 24 h. Although the trend in fluorescence in infected mice treated with Cy5.5-liposomes was similar to mice treated with CFW–CHSc–Cy5.5-liposomes (Fig. 4C), the fluorescence signals were significantly lower than similarly injected CFW–CHSc–Cy5.5-liposome mice. The fluorescence signals almost disappeared after 24 h. These results indicate that two nanoparticle formulations can be delivered into the peritoneal cavity via intravenous injection, but the CFW–CHSc–Cy5.5-liposome half-lives are dramatically longer in infected tissues in mice.
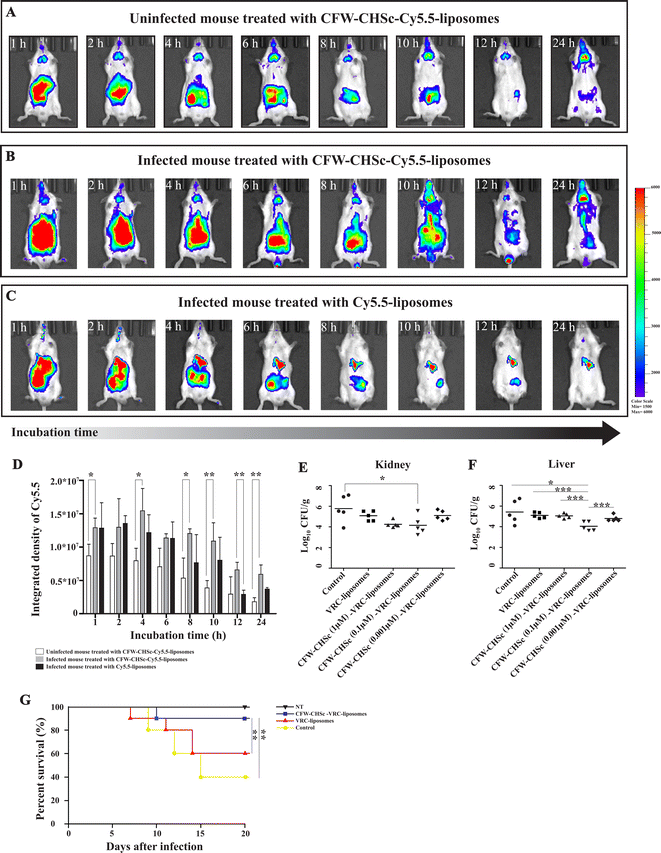 |
| Fig. 4 (A–C) Fluorescence imaging was performed at different times using an IVIS Spectrum. Balb/c mice were divided into three groups of n = 5 and treated intravenously with different fluorescent nanoparticles. Each mouse was examined at seven post-treatment time points. (A) Uninfected mice were treated with CFW–CHSc–Cy5.5-liposomes. (B) Mice were infected intraperitoneally with C. albicans cells and then treated with CFW–CHSc–Cy5.5-liposomes. (C) Infected mice were treated with Cy5.5-liposomes. (D) Quantitative measurements of the integrated density of Cy5.5 were performed using ImageJ. Vertical error bars represent the mean ± SD obtained from five mice in each group at each post-treatment time point (**P ≤ 0.01; *P ≤ 0.05). (E and F) C57BL/6 mice were divided into five groups of five and infected intraperitoneally with C. albicans cells. Intravenous treatment of CFW–CHSc (0.1 μM)–VRC-liposomes significantly inhibited C. albicans proliferation in mouse liver and kidney tissues compared to VRC-liposome and CFW–CHSc (1 μM and 0.01 μM)–VRC-liposome treatment (***P ≤ 0.001; *P ≤ 0.05). (G) Survival curves were plotted for the following four groups (n = 10 for each group); the control without VRC treatment; CFW–CHSc (0.1 μM)–VRC-liposome treatment; VRC-liposome treatment and healthy mice (**P ≤ 0.01). | |
To exclude interference from the non-specific binding of CFW–CHSc–liposomes in the host, uninfected mice were treated with CFW–CHSc–Cy5.5-liposomes. Fig. 4A shows that fluorescence signals were observed in the peritoneal cavity in uninfected mice after 1 h. The signal of Cy5.5 lasted for a short time in uninfected mice. These results suggest that CFW–CHSc–Cy5.5-liposomes might be metabolized quickly in uninfected mice. Intriguingly, fluorescence signals were observed in mouse brain, which indicated that CFW–CHSc-liposomes might pass through the blood–brain barrier and could have value in fungal meningitis treatment.
ImageJ analysis (Fig. 4D) shows that infected mice treated with CFW–CHSc–Cy5.5-liposomes maintained an integrated density of Cy5.5 around 1.25 × 107 after 10 h. There was a significant decrease in the integrated density of Cy5.5 after 12 h but only 8 h in Cy5.5-liposomes. The integrated density of Cy5.5 in infected mice treated with CFW–CHSc–Cy5.5-liposomes was obviously higher (1-fold to 2.3-fold) than that of infected mice treated with Cy5.5-liposomes. The integrated density of Cy5.5 in uninfected mice treated with CFW–CHSc–Cy5.5-liposomes gradually decreased with time compared to that of infected mice (1.5-fold to 3.3-fold lower). Together, these results suggest that the amount of CFW–CHSc–Cy5.5-liposomes in infected tissues is significantly higher than that of Cy5.5-liposomes. This improved performance might be because of the active targeting ability of CFW–CHSc: it increases nanoparticle enrichment at fungal infection sites via blood circulation. The enhanced drug delivery efficacy greatly encourages subsequent therapy of antifungal drug-loaded CFW–CHSc-liposomes against C. albicans in vivo.
Antifungal activity of CFW–CHSc–VRC-liposomes in vivo
To evaluate the impact of CFW–CHSc on drug activity against C. albicans in vivo, five groups of C57BL/6 mice infected with C. albicans yeast cells by intraperitoneal injection were intravenously administered 2 mg kg−1 CFW–CHSc (from 1 μM to 0.01 μM)–VRC-liposomes or VRC-liposomes once daily for seven days. Mice in the control group were treated with appropriate CFW–CHSc-liposomes in saline solution. At 8 days post-infection, the liver and kidneys were homogenized, and colony-forming units were quantified. There was an obvious reduction in the fungal burden between groups treated with both VRC-loaded nanoparticle formulations and the control group (Fig. 4E and F). Strikingly, a significantly lower number of CFUs were detected in the livers and kidneys of the CFW–CHSc (0.1 μM)–VRC-liposomes treatment group compared to that of the VRC-liposome treatment group, e.g., an 11.5-fold difference in the livers and an 8.1-fold in the kidneys. Meanwhile, the number of CFUs in the CFW–CHSc (0.1 μM)–VRC-liposome treatment group was obviously lower than that in the CFW–CHSc (1 μM)–VRC-liposome and CFW–CHSc (0.01 μM)–VRC-liposome groups, which corresponded to the cellular uptake study. Besides, mice treated with CFW–CHSc (0.01 μM)–VRC-liposomes showed a significantly lower mortality rate than the VRC-liposome treatment group (Fig. 4G).
Hematoxylin and eosin (H&E) staining of the liver and kidneys (Fig. 5) shows that there were obvious pathological changes in the liver and kidneys due to C. albicans infection in the control group (Fig. 5A and E) such as small abscesses formed by the infiltration of numerous neutrophils and necrotic foci with relatively few inflammatory cells. There were also granulation tissues surrounding the site of small abscess. In the VRC-liposome treatment group (Fig. 5B and F), there were mild tissue necroses at the sites of small abscess. In the CFW–CHSc (0.1 μM)–VRC-liposome treatment group (Fig. 5C and G), there were only a few lymphocyte and monocyte infiltrations and no obvious tissue response. Besides, there was no tissue damage in mice treated with CFW–CHSc (0.1 μM)–VRC-liposomes. In the healthy group (Fig. 5D and H), there were normal liver and kidneys.
 |
| Fig. 5 Histopathological examination of the liver (A–D) and kidneys (E–H) with H&E staining. At one day post-infection, mice were intravenously treated with VRC-liposomes or CFW–CHSc (0.1 μM)–VRC-liposomes. Histopathological examination was performed at 8 days post-infection. Red arrows indicate the necrotic foci caused by C. albicans infection in tissues. All scale bars are 100 μm. | |
These results suggest that the antifungal activity of CFW–CHSc–VRC-liposomes is significantly improved in the treatment of fungal peritonitis in mice versus VRC-liposomes. The outstanding fungistatic effect of CFW–CHSc–VRC-liposomes might be due to the addition of CFW–CHSc. The specific targeting ability of CFW–CHSc to C. albicans cells promotes the accumulation of VRC-loaded nanoparticles at the infected sites via blood circulation, which in turn significantly improves the antifungal drug activity of CFW–CHSc–VRC-liposomes. Meanwhile the study of antifungal activity of CFW–CHSc–VRC-liposomes in vivo indicates a conclusive safety of the material. Nanoparticles used for drug therapy offer many advantages over conventional formulations such as increased solubility, prolonged half-life, etc. However, fungi-targeting nanoparticles offer better and intelligent drug therapy during nosomycosis treatment.
Conclusion
Here, we successfully developed CFW–CHSc as a novel nanomaterial for efficient drug delivery in C. albicans. CFW–CHSc showed its specific binding ability to chitin chains in the cell wall and antifungal activity against C. albicans yeast cells in vitro at higher concentrations. CFW–CHSc succeeded both properties from CFW. We prepared CFW–CHSc–VRC-liposomes to determine the function of CFW–CHSc in a nanoparticulate drug delivery system. The CFW–CHSc–VRC-liposomes showed an increased inhibition rate at lower concentrations in cell growth-based in vitro assays versus VRC-liposomes. The drug delivery efficacy of CFW–CHSc–liposomes was assessed using fluorescence-based approaches in C. albicans cells and mice. The fluorescence images demonstrated that the improved drug delivery efficacy of CFW–CHSc-liposomes was due to their greater intracellular uptake in C. albicans yeast cells. The half-lives of CFW–CHSc–Cy5.5-liposomes were dramatically prolonged in infected mice due to the increased enrichment of nanoparticles at the fungal infection sites via the blood circulation system. CFW–CHSc-liposomes benefited from specific binding between CFW–CHSc and chitin and showed increased drug delivery efficacy in vitro and in vivo versus untargeted liposomes. Fungal burden analysis demonstrated that CFW–CHSc–VRC-liposomes significantly reduced the fungal burden in the mouse liver and kidneys versus VRC-liposomes strongly suggesting improved therapeutic efficacy. Collectively, our studies demonstrated the significance of CFW–CHSc as a novel nanomaterial targeting fungi in nanoparticle drug delivery systems. Fungi-targeting nanoparticles can be used in the treatment of nosomycosis and must be studied further.
Author contributions
Wei Liu: investigation, methodology, and data curation. Mengshun Li: investigation, conceptualization, and methodology. Baocheng Tian: investigation and software. Xuesong Yang: investigation. Wei Du: investigation and formal analysis. Xiuwen Wang: investigation. Huihui Zhou: histopathological evaluation. Chen Ding: supervision, validation, project administration, and writing – reviewing and editing. Sixiang Sai: conceptualization, visualization, writing – original draft preparation, and funding acquisition.
Conflicts of interest
There are no conflicts to declare.
Acknowledgements
This work was financially supported by the National Natural Science Foundation of China (81501784, 81703717, and 31870140) and the Liaoning Revitalization Talents Program (XLYC1807001).
References
- A. Arastehfar, A. Carvalho, F. L. van de Veerdonk, J. D. Jenks, P. Koehler, R. Krause, O. A. Cornely, D. S. Perlin, C. Lass-Florl and M. Hoenigl, COVID-19 Associated Pulmonary Aspergillosis (CAPA)-From Immunology to Treatment, J. Fungi, 2020, 6(2), 1–17 CrossRef.
- I. D. Jacobsen, Fungal infection strategies, Virulence, 2019, 10(1), 835–838 CrossRef CAS.
- J. R. Kohler, B. Hube, R. Puccia, A. Casadevall and J. R. Perfect, Fungi that Infect Humans, Microbiol. Spectrum, 2017, 5(3), 1–29 CrossRef PubMed.
- P. G. Pappas, C. A. Kauffman, D. R. Andes, C. J. Clancy, K. A. Marr, L. Ostrosky-Zeichner, A. C. Reboli, M. G. Schuster, J. A. Vazquez and T. J. Walsh,
et al., Clinical Practice Guideline for the Management of Candidiasis: 2016 Update by the Infectious Diseases Society of America, Clin. Infect. Dis, 2016, 62(4), e1–50 CrossRef.
- L. Drgona, A. Khachatryan, J. Stephens, C. Charbonneau, M. Kantecki, S. Haider and R. Barnes, Clinical and economic burden of invasive fungal diseases in Europe: focus on pre-emptive and empirical treatment of Aspergillus and Candida species, Eur. J. Clin. Microbiol. Infect. Dis., 2014, 33(1), 7–21 CrossRef CAS PubMed.
- D. S. Thompson, P. L. Carlisle and D. Kadosh, Coevolution of morphology and virulence in Candida species, Eukaryotic Cell, 2011, 10(9), 1173–1182 CrossRef CAS PubMed.
- S. M. Noble, B. A. Gianetti and J. N. Witchley, Candida albicans cell-type switching and functional plasticity in the mammalian host, Nat. Rev. Microbiol., 2017, 15(2), 96–108 CrossRef CAS PubMed.
- M. B. Lohse, M. Gulati, A. D. Johnson and C. J. Nobile, Development and regulation of single- and multi-species Candida albicans biofilms, Nat. Rev. Microbiol., 2018, 16(1), 19–31 CrossRef CAS PubMed.
- L. L. Benitez and P. L. Carver, Adverse Effects Associated with Long-Term Administration of Azole Antifungal Agents, Drugs, 2019, 79(8), 833–853 CrossRef CAS PubMed.
- G. Cavell, The Problem with Amphotericin, Clin. Drug Invest., 2020, 40(8), 687–693 CrossRef PubMed.
- M. C. Arendrup and T. F. Patterson, Multidrug-Resistant Candida: Epidemiology, Molecular Mechanisms, and Treatment, J. Infect. Dis., 2017, 216(suppl_3), S445–S451 CrossRef CAS PubMed.
- N. A. R. Gow, J. P. Latge and C. A. Munro, The Fungal Cell Wall: Structure, Biosynthesis, and Function, Microbiol. Spectrum, 2017, 5(3), 1–25 Search PubMed.
- J. P. Latge, The cell wall: a carbohydrate armour for the fungal cell, Mol. Microbiol., 2007, 66(2), 279–290 CrossRef CAS PubMed.
- J. Nett, L. Lincoln, K. Marchillo, R. Massey, K. Holoyda, B. Hoff, M. VanHandel and D. Andes, Putative role of beta-1,3 glucans in Candida albicans biofilm resistance, Antimicrob. Agents Chemother., 2007, 51(2), 510–520 CrossRef CAS.
- E. Iorio, A. Torosantucci, C. Bromuro, P. Chiani, A. Ferretti, M. Giannini, A. Cassone and F. Podo, Candida albicans cell wall comprises a branched beta-D-(1–>6)-glucan with beta-D-(1–>3)-side chains, Carbohydr. Res., 2008, 343(6), 1050–1061 CrossRef CAS.
- J. Ruiz-Herrera, M. V. Elorza, E. Valentin and R. Sentandreu, Molecular organization of the cell wall of Candida albicans and its relation to pathogenicity, FEMS Yeast Res., 2006, 6(1), 14–29 CrossRef CAS.
- A. da Silva Dantas, K. K. Lee, I. Raziunaite, K. Schaefer, J. Wagener, B. Yadav and N. A. Gow, Cell biology of Candida albicans-host interactions, Curr. Opin. Microbiol., 2016, 34, 111–118 CrossRef CAS.
- M. D. Lenardon, P. Sood, H. C. Dorfmueller, A. J. P. Brown and N. A. R. Gow, Scalar nanostructure of the Candida albicans cell wall; a molecular, cellular and ultrastructural analysis and interpretation, Cell Surf., 2020, 6, 100047 CrossRef CAS.
- M. Hejtmanek, J. Dolezel and I. Holubova, Staining of fungal cell walls with fluorescent brighteners: flow-cytometric analysis, Folia Microbiol., 1990, 35(5), 437–442 CrossRef CAS.
- R. Ruchel and S. Margraf, Rapid microscopical diagnosis of deep-seated mycoses following maceration of fresh specimens and staining with optical brighteners, Mycoses, 1993, 36(7–8), 239–242 CAS.
- J. E. Monheit, D. F. Cowan and D. G. Moore, Rapid detection of fungi in tissues using calcofluor white and fluorescence microscopy, Arch. Pathol. Lab. Med., 1984, 108(8), 616–618 CAS.
- H. M. Marines, M. S. Osato and R. L. Font, The value of calcofluor white in the diagnosis of mycotic and Acanthamoeba infections of the eye and ocular adnexa, Ophthalmology, 1987, 94(1), 23–26 CrossRef CAS PubMed.
- C. Roncero, M. H. Valdivieso, J. C. Ribas and A. Duran, Effect of calcofluor white on chitin synthases from Saccharomyces cerevisiae, J. Bacteriol., 1988, 170(4), 1945–1949 CrossRef CAS PubMed.
- A. F. Ram and F. M. Klis, Identification of fungal cell wall mutants using susceptibility assays based on Calcofluor white and Congo red, Nat. Protoc., 2006, 1(5), 2253–2256 CrossRef CAS PubMed.
- W. Herth, Calcofluor white and Congo red inhibit chitin microfibril assembly of Poterioochromonas: evidence for a gap between polymerization and microfibril formation, J. Cell Biol., 1980, 87(2 Pt 1), 442–450 CrossRef CAS PubMed.
- V. Chalupova, V. Raclavsky and R. Novotny, Rylux BSU stimulates spore germination in Trichophyton mentagrophytes and Aspergillus fumigatus and increases the survival rate after UV-irradiation, Folia Microbiol., 2002, 47(2), 152–156 CrossRef CAS PubMed.
- J. Brasch, I. Kreiselmaier and E. Christophers, Inhibition of dermatophytes by optical brighteners, Mycoses, 2003, 46(3–4), 120–125 CrossRef CAS PubMed.
- G. Sadeghi, A. A. Khaksar, S. Bassiri Jahromi, A. Amirkhani, A. Eslamifar, S. Ajdary, F. Katiraee, J. Taeb, F. A. Paskiabi and M. Sayyah, Fungistatic effects of optical brightener 220 against Trichophyton tonsurans, Aspergillus fumigatus and Candida albicans, J. Dermatol. Treat., 2009, 20(2), 120–123 CrossRef CAS PubMed.
- J. Haplova, V. Farkas, M. Hejtmanek, R. Kodousek and J. Malinsky, Effect of the new fluorescent brightener Rylux BSU on morphology and biosynthesis of cell walls in Saccharomyces cerevisiae, Arch. Microbiol., 1994, 161(4), 340–344 CrossRef CAS.
- C. Ding, R. A. Festa, T. S. Sun and Z. Y. Wang, Iron and copper as virulence modulators in human fungal pathogens, Mol. Microbiol., 2014, 93(1), 10–23 CrossRef CAS PubMed.
- H. Maeda and N. Ishida, Specificity of binding of hexopyranosyl polysaccharides with fluorescent brightener, J. Biochem., 1967, 62(2), 276–278 CrossRef CAS PubMed.
- B. Bowers, G. Levin and E. Cabib, Effect of polyoxin D on chitin synthesis and septum formation in Saccharomyces cerevisiae, J. Bacteriol., 1974, 119(2), 564–575 CrossRef CAS PubMed.
- X. Z. Wu, A. X. Cheng, L. M. Sun and H. X. Lou, Effect of plagiochin E, an antifungal macrocyclic bis(bibenzyl), on cell wall chitin synthesis in Candida albicans, Acta Pharmacol. Sin., 2008, 29(12), 1478–1485 CrossRef CAS.
- L. A. Walker, C. A. Munro, I. de Bruijn, M. D. Lenardon, A. McKinnon and N. A. Gow, Stimulation of chitin synthesis rescues Candida albicans from echinocandins, PLoS Pathog., 2008, 4(4), e1000040 CrossRef PubMed.
- G. M. Soliman, Nanoparticles as safe and effective delivery systems of antifungal agents: Achievements and challenges, Int. J. Pharm., 2017, 523(1), 15–32 CrossRef CAS PubMed.
- S. K. Singh, M. K. Hidau, S. Gautam, K. Gupta, K. P. Singh, S. K. Singh and S. Singh, Glycol chitosan functionalized asenapine nanostructured lipid carriers for targeted brain delivery: Pharmacokinetic and teratogenic assessment, Int. J. Biol. Macromol., 2018, 108, 1092–1100 CrossRef CAS PubMed.
- S. Hernando, E. Herran, J. Figueiro-Silva, J. L. Pedraz, M. Igartua, E. Carro and R. M. Hernandez, Intranasal Administration of TAT-Conjugated Lipid Nanocarriers Loading GDNF for Parkinson's Disease, Mol. Neurobiol., 2018, 55(1), 145–155 CrossRef CAS PubMed.
- A. Lyden, L. Lombardi, W. Sire, P. Li, J. C. Simpson, G. Butler and G. U. Lee, Characterization of carboxylate nanoparticle adhesion with the fungal pathogen Candida albicans, Nanoscale, 2017, 9(41), 15911–15922 RSC.
- F. A. de Sa, S. F. Taveira, G. M. Gelfuso, E. M. Lima and T. Gratieri, Liposomal voriconazole (VOR) formulation for improved ocular delivery, Colloids Surf., B, 2015, 133, 331–338 CrossRef CAS PubMed.
- Z. Zhang and S. S. Feng, The drug encapsulation efficiency, in vitro drug release, cellular uptake and cytotoxicity of paclitaxel-loaded poly(lactide)-tocopheryl polyethylene glycol succinate nanoparticles, Biomaterials, 2006, 27(21), 4025–4033 CrossRef CAS PubMed.
- B. Tian, Q. Yan, J. Wang, C. Ding and S. Sai, Enhanced antifungal activity of voriconazole-loaded nanostructured lipid carriers against Candida albicans with a dimorphic switching model, Int. J. Nanomed., 2017, 12, 7131–7141 CrossRef CAS PubMed.
- C. Yu, B. He, M. H. Xiong, H. Zhang, L. Yuan, L. Ma, W. B. Dai, J. Wang, X. L. Wang and X. Q. Wang,
et al., The effect of hydrophilic and hydrophobic structure of amphiphilic polymeric micelles on their transport in epithelial MDCK cells, Biomaterials, 2013, 34(26), 6284–6298 CrossRef CAS PubMed.
- Y. Dong and S. S. Feng, In vitro and in vivo evaluation of methoxy polyethylene glycol-polylactide (MPEG-PLA) nanoparticles for small-molecule drug chemotherapy, Biomaterials, 2007, 28(28), 4154–4160 CrossRef CAS PubMed.
- Y. Ma, Y. Zheng, K. Liu, G. Tian, Y. Tian, L. Xu, F. Yan, L. Huang and L. Mei, Nanoparticles of Poly(Lactide-Co-Glycolide)-d-a-Tocopheryl Polyethylene Glycol 1000 Succinate Random Copolymer for Cancer Treatment, Nanoscale Res. Lett., 2010, 5(7), 1161–1169 CrossRef CAS PubMed.
- A. P. Gorka, R. R. Nani and M. J. Schnermann, Harnessing Cyanine Reactivity for Optical Imaging and Drug Delivery, Acc. Chem. Res., 2018, 51(12), 3226–3235 CrossRef CAS PubMed.
- L. Stackova, P. Stacko and P. Klan, Approach to a Substituted Heptamethine Cyanine Chain by the Ring Opening of Zincke Salts, J. Am. Chem. Soc., 2019, 141(17), 7155–7162 CrossRef CAS PubMed.
- R. B. Altman, Q. Zheng, Z. Zhou, D. S. Terry, J. D. Warren and S. C. Blanchard, Enhanced photostability of cyanine fluorophores across the visible spectrum, Nat. Methods, 2012, 9(5), 428–429 CrossRef CAS.
Footnotes |
† Electronic supplementary information (ESI) available: Stability assay of CFW-CHSc-VRC-liposomes and VRC-liposomes. 13C NMR spectrum of CFW. See DOI: https://doi.org/10.1039/d2bm01263d |
‡ Co-first authorship. These authors contributed equally and share co-first authorship. |
|
This journal is © The Royal Society of Chemistry 2023 |
Click here to see how this site uses Cookies. View our privacy policy here.