DOI:
10.1039/D2BM01515C
(Paper)
Biomater. Sci., 2023,
11, 322-338
NO released via both a Cu-MOF-based donor and surface-catalyzed generation enhances anticoagulation and antibacterial surface effects
Received
20th September 2022
, Accepted 13th November 2022
First published on 18th November 2022
Abstract
The anticoagulation and antibacterial functions of implant and interventional catheters during indwelling will determine their success or failure. Here, an amino-containing copper-based metal–organic framework (Cu-MOF) coating was prepared on the thermoplastic polyurethane substrate (TPU) surface by spin coating for anti-thrombotic and anti-infection effects. The adhesion properties of the polyurethane prepolymer coating (PC) enhanced the binding force of Cu-MOF particles and TPU surface and improved stability. Due to the coordination affinity of Cu2+ with nitric oxide (NO) and the NO loading capacity of the amino group, it showed that a large amount of NO was loaded in the coating. Meanwhile, the coordinated Cu2+ in the coating also catalyzed endogenous NO donors to generate NO, which prolonged the NO release for up to 30 days. The results of antibacterial experiments showed that the NO released from the coating had good antibacterial effects on both E. coli and S. epidermidis. An obvious antibacterial ring can be seen and the antibacterial rate was higher than 96%. It also showed inhibiting platelet adhesion and activation, prolonged in vitro clotting time and inhibited thrombus formation. In summary, for the first time, NO release from the coating was realized by the combined ways of NO donor and catalytic endogenous NO donor. It can not only meet the high NO release rate required for early anticoagulation and antibacterial of the catheter but also maintain normal anticoagulation requirements in the later period.
1. Introduction
Implanted and interventional catheters such as central venous catheters (CVCs) are commonly used intravascular catheters in cardiovascular system catheters and devices. It plays an important role in long-term infusion, blood transfusion, hemodialysis, blood collection, and plasma exchange for patients, particularly since the market demand for CVCs is enormous in the clinic.1–4 However, due to the special physicochemical properties of the catheter surface, they are easily activated by protein and platelet adhesion, thereby inducing coagulation and related thrombosis. Severe cases will result in device failure, increasing patient morbidity, mortality, and healthcare costs.5–8 More seriously, the bacterial colonization and the formation of biofilms during catheter implantation lead to catheter-associated bacterial infections, which can cause severe complications.9,10 Currently, to solve these problems, injections of anticoagulants and antibiotics are common clinical treatments. However, these methods have the disadvantages of high doses, low efficacy, and many side effects.11 For example, heparin and antibiotics are used clinically to seal tubes to treat complications associated with thrombosis and bacterial infections. However, the overuse of heparin and antibiotics not only leads to side effects (increased risk of bleeding and thrombocytopenia) but also creates drug-resistant bacteria that can have a more serious impact on patient care.12,13 In addition, studies have shown that the construction of anti-adhesive property hydrophilic polymers on the catheter surface can inhibit the adhesion of blood cells, but still cannot completely avoid the adhesion of plasma proteins and blood cells. During catheter implantation, platelets and the coagulation system are still activated, causing thrombosis formation. Therefore, it is important to develop anticoagulation and antimicrobial modification technology suitable for implant/interventional catheter surfaces to address the problems with existing blood contact catheters.
Nitric oxide (NO) is an important biological signaling molecule, which plays a critical role in the cardiovascular system, anticoagulant, and antibacterial functions such as vasodilatation and inhibition of platelet aggregation and activation.14,15 Hence, researchers have developed NO loading or NO generating materials for blood-contacting devices. These materials include metal-NO complexes, diazeniumdiolates (NONOates), and NO donors, which were further encapsulated or conjugated onto various organic and inorganic biomaterial vectors to develop NO delivery systems.16,17 Among NO donors, RSNOs and NONOates are the two most widely studied categories due to their ability to release NO when exposed to different stimuli, such as physiological solutions, metal ions, and heat and light irradiation. For example, NONOates spontaneously decompose to generate two moles of NO per mole of donor with proton-initiation under physiological solution.18,19 NO-releasing biomaterials are beneficial in killing microbial strains and anti-thrombotic. Although NO gas has some satisfactory properties, its low NO payload, short-release half-life, thermal/photochemical instability, and insufficient specificity NO have greatly limited its application.20 Therefore, the prerequisite for its further application is to increase the loading capacity and the long-term sustainable and controlled release of NO.
In order to increase the loading capacity of NO and prolong its release time, researchers have used porous inorganic materials such as silica particles, zeolites, and metal–organic frameworks (MOFs) as vectors for NO loading.21,22 Among them, MOFs are widely used for gas adsorption, storage, and release due to their chemical tunability, high number of active sites, high porosity, good thermal stability, and easy functionalization.23 Among these functions, due to the presence of coordination unsaturated metal sites (CUS) inside the Cu based metal organic frameworks (Cu-MOFs) that can form strong bonds with NO, and the ability of Cu-MOFs to post modification amino groups to bind NO to achieve the quantitatively loading. All of these advantages make Cu-MOFs a promising carrier for stable and long shelf-life NO storage applications.24 In addition, not only the coordinated Cu2+ can catalyze the release of NO from endogenous NO donors in situ, but also the release of Cu2+ may kill bacteria by binding to the bacterial surface and destroying the cell membrane.25 However, due to the instability of Cu-MOFs in water environments and the difficulty of immobilizing them on inert materials, their applications are severely limited. Meanwhile, achieving Cu-MOF catalyzed NO release from endogenous NO donors requires tedious pretreatment of the material, and few studies have reported the anticoagulant and antimicrobial applications of the Cu-MOF catalyzed NO release on the medical catheter surface.
Herein, a simple spin-coating method was used to prepare a Cu-MOF@polyurethane prepolymer coating (Cu-MOFs@PC) on the TPU surface. Due to the strong tackiness of PC, it can enhance the adhesion of the Cu-MOFs particles and the TPU substrate, forming a hierarchical structure consisting of multiple layers of Cu-MOFs particles. Then, the nitric oxide@Cu-MOFs@PC (NO@Cu-MOFs@PC) coating was obtained after high-pressure loading of NO. This coating can spontaneously release NO in an aqueous environment. Meanwhile, it can also continue to catalyze the in situ release of NO gas from the NO donor due to the presence of coordinated Cu2+. This way of combining NO release from NO donor and catalytic release of NO enables the long-term stable release of NO on the surface of the implanted catheter, with a release period of up to 30 days. The anticoagulant and antibacterial properties of the coating were further evaluated by in vitro and ex vivo blood tests as well as in vitro bacterial tests. The experimental results showed that the NO@Cu-MOFs@PC coating can effectively resist platelet adhesion and activation and displayed an excellent anti-thrombogenic ability. The results of the antibacterial experiments showed that NO@Cu-MOFs@PC coating could effectively inhibit the growth of E. coli and S. epidermidis, and disrupts the cell walls of E. coli and S. epidermidis, causing them to release their contents, demonstrating its excellent antibacterial ability.
2. Experimental
2.1. Materials
1,3,5-Benzenetricarboxylic acid (98%), 2-aminoterephthalic acid (98%), copper(II) nitrate hydrate (Cu(NO3)2·3H2O), S-nitrosoglutathoine (GSNO) and L-glutathione (GSH) were all purchased directly from Aladdin Chemicals Reagent Co (Shanghai, China). Ethanol (C2H5OH, 99.7%) was purchased from Jinshan Chemical Reagent Co. Ltd (Chengdu, China). Butanone was purchased from Kelong Chemical Co., Ltd (Chengdu, China). The phosphate-buffered saline (PBS, 0.01 M, pH = 7.4) was purchased from Yanyu Biotechnology Co (Chengdu, China). Nitric oxide (99.9%) was purchased from Chengdu Shimao Gas Co. The polyurethane substrate (TPU) was purchased from Foshan Lidali Plastic New Material Co., Ltd. Polyurethane prepolymer (PC) was provided by Huntsman Chemical Trading (Shanghai) Limited. Total Nitric Oxide Assay Kit (Beyotime Biotechnology, Shanghai, China) was used to detect NO release in vitro. All the reagents and solvents were commercially available and used as received without further purification.
2.2 Synthesis of Cu-MOF nanoparticles
The detailed preparation process for Cu-MOF nanoparticles using the hydrothermal method was reported in our previous work.26 A brief illustration of the method is given below. In a typical process, H3BTC (1 g, 4.7 mmol) and 2-aminoterephthalic acid (1 g, 5.5 mmol) were dissolved in a mixture of water (14 mL) and anhydrous ethanol (50 mL). After sonication at room temperature for 20 min, Cu(NO3)2·3H2O (1.7 g, 9.0 mmol) was added to continue ultrasonic dissolution. Finally, the mixture was placed into a 50 mL Teflon-lined stainless steel autoclave for hydrothermal growth at 140 °C for 1 h. Afterward, the precipitates were centrifuged and washed with ethanol and deionized water 2 times. After drying at 80 °C for 8 h, the sample was thoroughly ground with a mortar.
2.3 Preparation of Cu-MOFs@PC coating
The Cu-MOFs@PC coating was fabricated using spin-coating methods. The detailed preparation process is illustrated in Fig. 1. First, 90 mg Cu-MOFs nanoparticles were added to a 20 mL polyurethane prepolymer coating (PC) solution under magnetic stirring at room temperature (≤24 °C) for 48 h until all the nanoparticles were uniformly dispersed. Second, after the thermoplastic polyurethanes (TPU, 30 mm × 30 mm) substrate was degreased in ethanol and distilled water, TPU was firmly attached to the surface of the rigid plastic substrate using double-sided adhesive in order to prepare the Cu-MOFs@PC coating with an even thickness by the spin-coating method. Third, the well-stirred Cu-MOFs@PC nanoparticle precursor solution with a concentration of 4.5 mg mL−1 was dropped on the pretreated TPU substrate with 5 droplets (750 μL) per layer and spin-coated under the ambient conditions at 800 rpm for 40 s. After the spin coating was completed, the coating was placed in a 40 °C drying box and fully dried, then the spin coating was performed once again. After repeating the spin coating several times, the coating was kept at room temperature to naturally evaporate the solvent for 48 h to allow the coating to solidify.
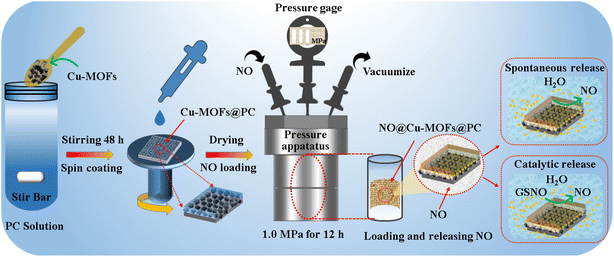 |
| Fig. 1 Schematic illustration of the preparation of the Cu-MOFs@PC coating, and schematic illustration of NO loading and release of the Cu-MOFs@PC coating. | |
2.4 NO loading and release
Before NO loading, the as-prepared Cu-MOFs@PC coating (30 mm × 30 mm) was packed into a quartz glass tube, and then transferred into a pressure apparatus, as shown in Fig. 1. Firstly, the chamber of the pressure apparatus was evacuated with a vacuum pump (2XZ-4 type rotary vane vacuum pump) for 60 min to maintain the pressure at a constant value of 0.1 MPa. Then, the samples were exposed to the NO environment under 1.0 MPa for 12 h to load NO on the Cu-MOFs@PC coating surface. The obtained coating was named NO@Cu-MOFs@PC and stored in a dry environment to prevent undesired release. The NO release from the NO@Cu-MOFs@PC coating surface was detected using the total NO assay kit according to the methods described in the previous studies. Apart from that, the rates of NO production on the NO@Cu-MOFs@PC coating without soaking and after PBS soaking (0 day, 1 day, 3 days, 5 days) were also measured using a Sievers 280i chemiluminescence NO analyzer (NOA 280i).
In addition, NO release by catalyzing endogenous NO donors to degrade the samples was tested as shown in Fig. 1. It was recorded using NOA 280i. The process was as follows. PBS (5 mL) with 20 μM GSNO and 20 μM GSH was added into the reaction vessel. Nitrogen was used as the carrier gas, the reaction vessel was protected from light at 37 °C, and the oxygen supply was 6.0 ± 0.2 psig. After the baseline stabilization, the coating (10 mm × 10 mm) was immersed in the solution and the NO flux was measured and recorded using a computer. In addition, the coating also was incubated in PBS for a period of time (5 days, 10 days, 15 days, 20 days, 25 days, 30 days), and the catalytic activity of the coating was continuously measured.
2.5 Characterization
The surface morphology of the Cu-MOFs@PC coating on the TPU substrate was characterized using a JSM-7800F field emission scanning electron microscope (SEM). The composition and content of the coating surface element were carefully detected by energy-dispersive spectroscopy (EDS). The morphology of the Cu-MOF nanoparticles was characterized in detail using a transmission electron microscope (TEM), and the corresponding element mapping of C, O, Cu, and N was performed synchronously. The chemical composition and crystal structure of Cu-MOFs@PC coating were analyzed by X-ray diffraction (XRD) and Fourier transform infrared (FTIR) spectroscopy. X-ray photoelectron spectroscopy (XPS) was used to study the surface chemical compositions. The water contact angle (WCA) was measured at room temperature by using an LSA100 system with a 5 μL water droplet.
2.6
In vitro evaluation of platelet adhesion and activation
In order to evaluate the blood compatibility of Cu-MOFs@PC coating with and without NO addition on the TPU sample, preliminary in vitro dynamic blood clotting time and platelet adhesion tests were conducted. Fresh whole blood was obtained from a New Zealand White rabbit. During the dynamic blood clotting test, 100 μL the fresh whole blood without anticoagulant was dropped on the Cu-MOFs@PC sample surface with and without loading of NO, and then placed in the wells of a 24-well cell culture plate, and the timing was started. After incubation at 37 °C for 5, 10, 20, and 30 min, 1 mL of distilled water was added to soak for 5 min. The absorbance (OD) of the mixed solution at 540 nm was detected using a microplate reader (Variosska LUX, Thermo). In the platelet adhesion test, the platelet-rich plasma (PRP) was obtained by centrifugation (1500 rpm, 15 min) of fresh anticoagulant rabbit blood. The four parallel samples for each group were placed into 24 well-cell culture plates and immersed in the 1 mL PRP. After incubation at 37 °C for 60 min, PRP was sucked up, and the samples were washed with PBS (pH = 7.4) and immersed in 2.5% glutaraldehyde overnight. Following further dehydration and de-alcoholization, the samples were gold-sputtered and examined by SEM. In addition, in this experiment, dendritic platelets were considered to be activated and the activation ratio of platelets was evaluated using statistical analysis.
2.7 Antibacterial test
The antibacterial activity of the surface of the sample was evaluated by the inhibition zone method and the colony counting method. Gram-positive staphylococcus epidermidis (S. epidermidis) and Gram-negative Escherichia coli (E. coli) were selected as model bacterial strains. The TPU substrate, PC, Cu-MOFs@PC, and NO@Cu-MOFs@PC coating samples were each cut into a square (10 mm × 10 mm), then irradiated with UV light for 30 min for sterilization. A 30 μL of bacterial suspension (106 CFU mL−1) was uniformly spread on the surface of Luria–Bertani (LB) agar, followed by placing the sterilized square samples on them. The agar plates were incubated at 37 °C for 24 h, and the zones of inhibition were observed. The plate colony counting method was used to investigate the antimicrobial ability of TPU, PC, Cu-MOFs@PC, and NO@Cu-MOFs@PC coating surfaces. The samples (10 mm × 10 mm) were placed onto 1 mL diluted bacterial suspension with a concentration of 106 CFU mL−1 and 10 mL of agar medium and cultured using a shaking table at 37 °C for 24 h. Then, 30 μL bacterial solution of each above samples was taken and coated on agar to count the colonies after 24 h culture.
3. Results and discussion
3.1 The morphologies, composition, and wettability of PC and Cu-MOFs@PC coatings
Usually, the number of spin-coating layers was also an important technical parameter to control the coating structure formation. Fig. 2A shows the low and high-resolution SEM images of the as-prepared pure PC (10 layers) coating and the Cu-MOFs@PC coating corresponding to 5, 10, and 15 spin-coating layers. The SEM analysis demonstrated that the surface of pure PC is very flat and smooth (seen from the SEM and its inset), and nothing is observed in the large area of the surface. With the introduction of Cu-MOF nanoparticles, the surface morphology changed significantly. As shown from low and high-resolution SEM images, the Cu-MOFs@PC coating surface at 5 layers has some sparsely dispersed protrusion, which can be attributed to the aggregation caused by the absence of uniformly dispersed Cu-MOFs nanoparticles. As the spin-coating layers increase to 10 layers, it is seen that the area of the surface protrusion becomes larger and the protrusion number increases significantly. It was observed from the high-magnification SEM images that the protrusions were made up of a large number of Cu-MOF nanoparticles. When the spin-coating layers are further increased to 15 layers, all protrusions with Cu-MOF nanoparticles accumulated to form a closely packed granular film. In summary, the obtained coating with spin-coating 10 layers displays a relatively well-distributed structure and high compactness. Notably, with the increase in the spin-coating layers, the latter layers of Cu-MOF nanoparticles are more evenly and fully covered on the previous layer of Cu-MOF nanoparticles, while these nanoparticles are regular and overlap each other in the gaps. It will be more favorable for the loading and release of NO, further affecting the anticoagulant and antibacterial effect of the coating surface.
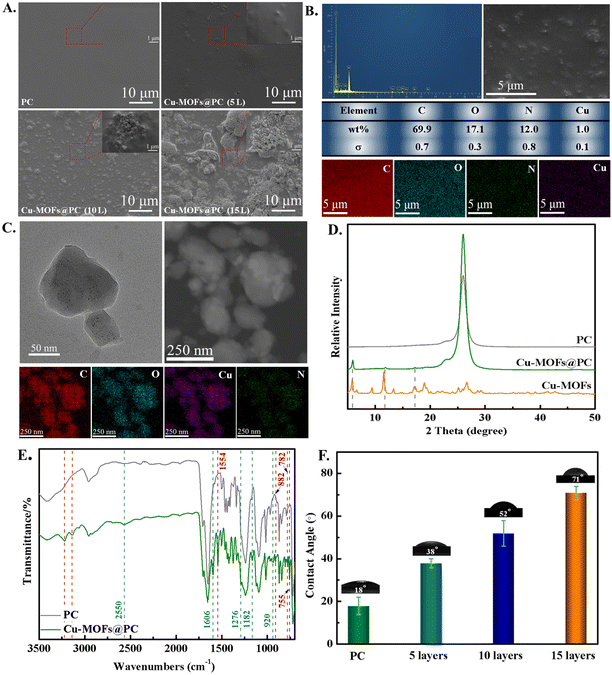 |
| Fig. 2 (A) SEM images of the prepared pure PC and Cu-MOFs@PC coatings under the different spin-coating layer numbers of 5 layers (5 L), 10 layers (10 L) and 15 layers (15 L). The inset on the top right is the higher magnification image. (B) The SEM image and energy dispersive spectra (EDS) of Cu-MOFs@PC coating on the TPU substrate, and the surface element mapping of the carbon, oxygen, copper, and nitrogen elements, respectively. (C) TEM and corresponding EDX elemental (C, O, N, and Cu) mapping analysis of Cu-MOFs@PC. XRD patterns (D) and FTIR spectra (E) of the Cu-MOFs@PC (10 L) composite coating surface on the TPU substrate. (F) The WCA of the PC coating and Cu-MOFs@PC coating surface at the 5 layers (5 L), 10 layers (10 L) and 15 layers (15 L). | |
Furthermore, in order to further investigate whether the Cu-MOFs nanoparticles entered the blank location, the energy dispersive spectra (EDS) analysis of the Cu-MOFs@PC coating was performed, as shown in Fig. 2B. It was obvious that carbon, oxygen, nitrogen, and copper elements existed in the Cu-MOFs@PC coating. Based on the results, the element mapping was performed on the entire area of Cu-MOFs@PC coating. As can be clearly seen, the uniform distributions of the main elements (C (red), O (blue), N (green), and Cu (purple) in the area determined for elemental analysis indicate that the Cu-MOFs particles are uniformly dispersed in the Cu-MOFs@PC coating. The uniform distribution of Cu and N verifies that the Cu-MOFs particles were mainly buried in the PC coating. The results further indicated that the Cu-MOFs@PC coating was successfully fabricated on the TPU surface. Transmission electron microscopy (TEM) imaging of the Cu-MOFs nanoparticles showed a polyhedral shape, and most of them had lateral dimensions between 50 nm and 150 nm (Fig. 2C). The TEM images have small white spots over the nanoparticles, meaning that the electrons can go through the particles, confirming their porous structure. In order to verify the composition of Cu-MOF nanoparticles, the element mapping of C, O, Cu and N is shown in Fig. 2C. The element mapping of Cu-MOF nanoparticles demonstrated that the signals of the typical elements C, O, Cu, and N were dispersed homogeneously across the selected area of the Cu-MOF nanoparticles, further confirming that the Cu-MOFs@PC coating was successfully prepared.
In order to verify the phase composition of pure PC and Cu-MOFs@PC coating, the XRD patterns of the as-prepared Cu-MOFs@PC coating and only the PC as sol were obtained and are shown in Fig. 2D. The X-ray diffraction (XRD) results showed significant diffraction peaks within 5–20° of 2θ and the position and relative intensity of the diffraction peaks of Cu-MOFs@PC were mainly consistent with the results obtained from our previous works.26 However, pure PC coating did not show a peak corresponding to Cu-MOFs at 0–20°, further indicating the successful preparation of Cu-MOFs@PC coating.
Furthermore, in order to further explore the functional groups on the pure PC and the Cu-MOFs@PC coating surface, the FTIR spectra of the pure PC and Cu-MOFs@PC coatings were recorded, as shown in Fig. 2E. The characteristic peaks shown by FTIR are fully consistent with those of Cu-MOFs synthesized in our previous works.26 But the peak does not appear in the spectrum of the pristine PC. In addition, for the Cu-MOFs@PC coating, the double peaks at 3137 cm−1 and 3224 cm−1 could be considered as –NH2. The absorption peak at 1606 cm−1 was assigned to –NH2 bending vibrations. It is well known that the surface structure is an important factor affecting surface wettability, and the change of wettability in turn affects the biocompatibility of materials, especially blood compatibility. Therefore, it is particularly important to test the wettability of material surfaces. Fig. 2F shows the WCA photos of the pure PC and different spin-coating layers. It can be seen that the WCA of pure PC coating surface is only 18°. After Cu-MOF material was added, WCA increased slightly and increased with the increase in spin-coating layers, which were 38°, 52° and 71° respectively. Although the 15 layer-coating has the largest WCA, we conjecture that too many nanoparticles exposed on the surfaces of 15 layers will be degraded by water to produce toxicity. Therefore, Cu-MOFs material with 10 layers was selected for subsequent studies.
3.2 Mechanical stability of Cu-MOFs@PC coating
Many medical coatings are easily destroyed or removed from the TPU surface due to the weak bonding of the coating to the substrate and poor mechanical stability. In order to verify the mechanical stability of Cu-MOFs@PC coating in various physically damaging environments,27 two qualitative tests of curling test and an ultrasound experiment were completed. Firstly, the curling test was taken by curling 180° on the Cu-MOFs@PC coating surface by the inward and outward curling cycles. From the SEM images shown in Fig. 3A for the coating curled for a different number of cycles, it can be seen that the coating has excellent mechanical stability under 300 curling cycles and the surface morphology was almost unchanged. When the number of curling cycles was increased to 400, some cracks appeared on the coating surface. This is mainly due to the fracture and extrusion of agglomerated large particles during the curling cycle, but the surface morphology of the coating remained unchanged over a wide range. It was proved that the Cu-MOFs@PC coating had excellent mechanical stability on the TPU surface. The mechanical stability of the coating was further demonstrated by testing the variation of the WCA of the coating surface under different curling cycles, as shown in Fig. 3B. It can be seen that the WCA did not change significantly with the increase of curling cycles, even after 400 cycles of curling cycles, WCA was still at about 53°. Obviously, the results prove that the prepared Cu-MOFs@PC coating has excellent mechanical stability and durability. The insets are the optical photos of the measured WCA on the coating surface. For the ultrasound treatment, the Cu-MOFs@PC coating was placed into a small bottle filled with deionized water, then the bottle was transferred into an ultrasonic pool and the Cu-MOFs@PC coating was ultrasonically treated for 60 min. It is noteworthy that the surface morphology of the coating did not change after 60 min of ultrasonic treatment, and C, O, N, and Cu elements were uniformly distributed on the coating surface, as shown in Fig. 3C. Obviously, the more intense tests of the curling test and ultrasound treatment validated the mechanical robustness of the Cu-MOFs@PC coating on the TPU substrate.
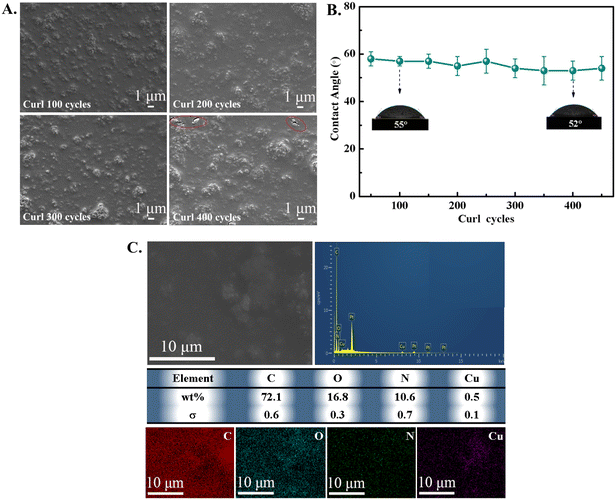 |
| Fig. 3 (A) The SEM photographs of the coating at different curling cycles. (B) The WCA variation with different curling cycles. (C) The SEM photographs of the coating surface after 60 min of ultrasonic treatment and the energy dispersion spectrum (EDS). | |
3.3 NO loading and releasing detection
Further, we used FTIR to study whether NO was successfully loaded, as shown in Fig. 4A. It is evident that several new peaks at 1628 and 1275 cm−1 appeared in the spectrum of NO@Cu-MOFs@PC coating compared to that of the Cu-MOFs@PC coating, and these are ascribed to the stretching vibrations of the N–O, N–H, N–O, N–O, and N
N bonds, respectively.28,29 Furthermore, upon loading with NO, peaks at 1040 and 949 cm−1 appeared in the NO@Cu-MOFs@PC coating, and the stretching frequencies at 1040–1043 cm−1 (N–O) are characteristic of NONOates.30 These peaks may be attributed to the interaction of NO with the Cu-MOFs.31–33 Meanwhile, the shoulder at 700 cm−1 disappeared after NO loading, this signal is typical of –N–H deformation vibrations in secondary amines and would also suggest NONOates formation.34 It is worth noting that there is also an additional stretching band at 2292 cm−1 present in all NO-modified compounds, which is most likely due to the N–N stretch.35 This leads to the conclusion that NO is coordinated to the Cu centers on one side and reacts with the amine groups to form NONOates groups at the same time. The FTIR results indicated that Cu-MOFs@PC coating can load NO via NONOates formation.
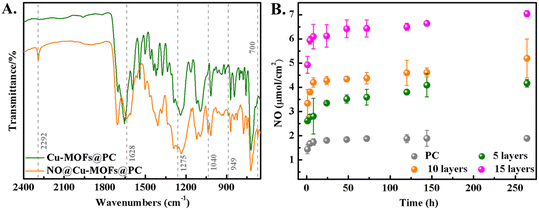 |
| Fig. 4 Characterization of NO loading and releasing of Cu-MOFs@PC coating surface. (A) The FTIR patterns of Cu-MOFs@PC coating before and after loading NO. (B) Cumulative release of NO from pure PC coating, and the Cu-MOFs@PC coating under the different spin-coating layer number of 5 layers, 10 layers and 15 layers in PBS (pH = 7.4) NO detected by the Griess assay. Values are expressed as mean ± standard deviation (SD) (n = 3). | |
The NO release behavior of NO-loaded PC and NO@Cu-MOFs@PC coating are shown in Fig. 4B, which is the total cumulative amount of NO released per square centimeter of coating versus time. It can be seen that the NO release from pure PC reached more than 1.6 μmol cm−2 after soaking for 8 h, and little NO within 268 h afterward was released and nearly not released. The NO@Cu-MOFs@PC coating significantly enhanced and prolonged the release of NO compared to the release of NO loaded in the pure PC coating. The total release and the release time of NO@Cu-MOFs@PC coating increased with the increase of the spin coating layer, both of which could sustain the release of NO for up to 268 h. It was further demonstrated that the number of Cu-MOF particles in the coating increased with the increase of the spin coating layer. Since the NO cumulative release of 15 layers was beyond the safe range for humans, and the NO release of 5 layers was smaller, we chose 10 layers of NO@Cu-MOFs@PC coating in the follow-up study. The cumulative release of this coating was 5.19 μmol cm−2. These results suggest that NO can be released controllably in the NO@Cu-MOFs@PC coating at low concentrations, which will greatly improve the efficacy of NO as a therapeutic agent.
3.4 Chemical stability of NO@CuMOFs@PC coating
Furthermore, for the sustained NO release, the stability of Cu-MOFs@PC coating in PBS is critical, as shown in Fig. 5A. The NO@Cu-MOFs@PC coating was incubated in PBS for preset times, and Cu2+ concentration was measured. The experimental results showed a rapid release of Cu2+ during the initial 3 days, it is possible that the unstable Cu-MOFs was shed and degraded on the coating surface, resulting in a rapid loss of these Cu2+. Then, the release of Cu2+ became slow and stable as the immersion time increases, and the release amount was about 0.138 mg L−1 even after 30 days. This might be due to the layer-by-layer decomposition of the nano Cu-MOFs inside the coating. These results indicate that PC coating can effectively improve the stability of Cu-MOFs and slow down the release rate of Cu2+ in the aqueous environment.
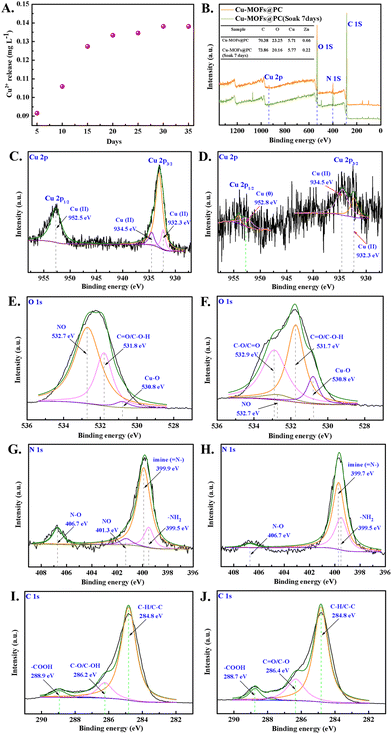 |
| Fig. 5 (A) Cumulative release of Cu2+ from the Cu-MOFs@PC coating in PBS (pH = 7.4, 37 °C). (B–J) XPS survey spectra and high-resolution spectra of the Cu-MOFs@PC coating before and after 7 days of immersion in PBS (pH = 7.4, 37 °C). | |
Moreover, due to the instability of Cu-MOFs in the water environment, the Cu-MOFs exposed on the surface are easy to degrade and disappear, thus affecting the release behavior of NO. Therefore, XPS analyses were performed to investigate the surface composition of Cu-MOFs@PC coating before and after 7 days of immersion. The C, O, N, and Cu elements were detected in the NO@Cu-MOFs@PC coating, shown in the survey spectra of Fig. 5B. According to their corresponding atomic concentration, it can be seen that the Cu2+ on the coating surface were still present despite a partial loss after 7 days of immersion. Therefore, it can be boldly assumed that the loaded NO still exists on the surface of the coating, and this conjecture is further verified in the previous NO-release behavior and the subsequent NO-release rate results. Further from the high-resolution XPS spectra of Cu 2p, it can be seen that the separation peaks at 932.3 eV, 934.5 eV, and 952.5 eV for the NO@Cu-MOFs@PC coating before immersion represent divalent copper (Cu(II)) as shown in Fig. 5C. The separation peaks at 932.3 eV and 934.5 eV after immersion represent divalent copper (Cu(II)),36,37 while the separation peak at 952.8 eV represents the zerovalent copper (Cu(0)), as shown in Fig. 5D.38 The results showed that the valence state of elemental copper has partially changed before and after soaking, but the main valence state is still (Cu(II)), which will help the later Cu-MOFs@PC coating to further catalyze the release of NO from NO donors. As shown in Fig. 5E, the high-resolution XPS spectra of O 1s before and after immersion, it can be seen that the separation peaks at 530.8 eV, 531.8 eV, and 532.7 eV for the coating before immersion represent the Cu–O bond, C
O/C–O–H bond and NO, respectively. These binding energies are in good agreement with the literature,39 indicating that the Cu-MOFs@PC coating was successfully loaded with NO. Further from the O 1s spectra after immersion, as shown in Fig. 5F, the NO peak at 532.7 eV of the NO@Cu-MOFs@PC coating was significantly weakened after immersion. It is proved that NO loaded in the coating will be replaced by the aqueous environment replacing after 7 days of immersion in PBS solution, thus reducing the NO content. The two peaks at binding energies of 399.5 eV and 399.7 eV in the high-resolution N 1s spectrum are shown in Fig. 5G, corresponding to –NH2 and imine (
N–), respectively, further demonstrating that the Cu-MOFs@PC coating was successfully prepared on the medical catheter surface. In addition, a fitted peak of NO with a binding energy of 401.3 eV was found on the sample surface before immersion, whereas no fitted peak of NO was found in the sample after the immersion. It was further shown that NO was successfully loaded on the surface of the Cu-MOFs@PC coating and that the PBS solution easily displaced NO from the coating surface, as shown in Fig. 5H. Although there were significant differences in the composition of other chemical elements on the surface before and after immersion, they did not affect the high-resolution XPS spectra of C 1s, as shown in Fig. 5I–J.
3.5 NO-release rate detection
As a gaseous drug with concentration-dependent therapeutic effects, appropriate and stable NO generation is an important determinant of the good anticoagulant and antibacterial ability of Cu-MOFs@PC coating. A real-time chemiluminescent assay (Sievers NOA280i) was used to monitor the NO flux from the NO@Cu-MOFs@PC coating surface in PBS solution (pH = 7.4). A steady and continuous release of NO was observed when the NO@Cu-MOFs@PC coating was submerged into the PBS solution (Fig. 6A). The results showed that the interposition of pure PC did not increase the NO flux, a steady and continuous release of NO was observed when NO@Cu-MOFs@PC was submerged into the PBS solution. The NO release induced by the NO@Cu-MOFs@PC appeared stable and the release rate was considered suitable, being in the range of the optimal physiological value (0.5–4 × 10−10 mol cm−2 min−1).40 In addition, the release rate of NO on the surface of NO@Cu-MOFs@PC coating decreased with the increase of soaking days, but the samples exhibited stable and good long-term NO catalytic activity even upon exposure to PBS 7 days, and all values were in the range of NO release by healthy ECs, as shown in Table 1. Our results are consistent with the results obtained using the total nitric oxide kit assay, indicating that the NO loaded in the coating can be released slowly, which greatly prolongs the release time. The stable and appropriate NO generation is crucial to achieve good anticoagulant and antimicrobial properties.
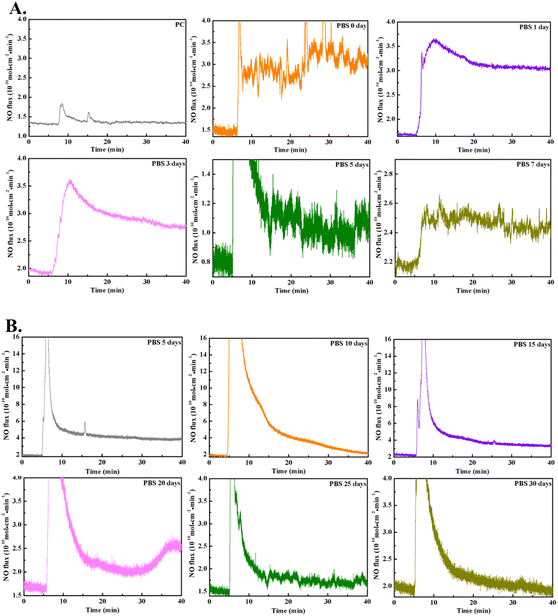 |
| Fig. 6 (A) Variation of NO release rate with time of pure PC coating, and variation of the NO release rate with time after NO@Cu-MOFs@PC coating immersed in PBS for 0, 1, 3, 5 and 7 days. (B) A plot of the rate of NO release from endogenous donor GSNO catalyzed by Cu-MOFs@PC after immersion in PBS for 5, 10, 15, 20, 25, and 30 days. | |
Table 1 NO-release rates of NO@Cu-MOFs@PC coating soaked in PBS for different days
Soaking days |
NO release rate (mol cm−2 min−1) |
0 |
(1.75 ± 0.4) × 10−10 |
1 |
(1.4 ± 0.05) × 10−10 |
3 |
(1.0 ± 0.05) × 10−10 |
5 |
(0.4 ± 0.25) × 10−10 |
7 |
(0.2 ± 0.06) × 10−10 |
In addition, due to Cu2+ can catalyze endogenous donor GSNO to release NO, based on the results of the Cu2+ release, the Cu2+ in the coating can be released stably for 35 days, so even if the loaded NO is completely released, it is still possible to use the catalytic effect of coordinated Cu2+ itself to decompose the endogenous NO donor GSNO to generate NO for the purpose of long-term NO release. The reaction is 2GSNO = 2NO + GSSG. The rate of NO release in this model is subject to the endogenous donor and the coordinated Cu2+ in the materials. Therefore, we further explored the NO release catalyzed of NO@Cu-MOFs@PC coating in real-time by using a Sievers NOA280i chemiluminescence analyzer. The NO release was monitored by recording the NO flux with time. An initial burst of NO release from the coating was observed when the NO@Cu-MOFs@PC coating soaked in PBS for 5 days was immersed in the donor. However, soon the NO release induced by the NO@Cu-MOFs@PC appeared stable and the release rate was considered suitable (2.8 × 10−10 mol cm−2 min−1), being in the range of the optimal physiological value. In addition, it was also observed that the NO flux decreased with an increasing number of days of immersion, which may be due to the decrease in coordinated Cu2+ due to the continuous degradation of Cu-MOFs in the coating. However, NO@Cu-MOFs@PC exhibited good long-term NO catalytic activity even upon exposure to PBS for 30 days and retained an NO release rate of 0.3 × 10−10 mol cm−2 min−1, as shown in Fig. 6B. This way of combining NO release from NO donor and catalytic release of NO enables the long-term stable release of NO on the coating surface, which is beneficial to the long-term anticoagulant and antibacterial effects of the implanted interventional catheters.
3.6
In vitro evaluation of hemocompatibility
Hemocompatibility of implantable or interventional catheters is the most important property of the blood-contacting materials, and the potential inhibition of NO@Cu-MOFs@PC coating on hemolysis and platelet activation was further evaluated. We first tested blood compatibility using a dynamic clotting time assay, which is particularly important for the implantable catheter. Fig. 7A presents the clotting time curves for Cu-MOFs@PC and NO@Cu-MOFs@PC coatings at different times of incubation with fresh blood (5 min, 10 min, 20 min, and 30 min); 1 mL of distilled water was added and the absorbance was detected at 545 nm. The higher the value of absorbance, the lower the degree of blood coagulation after contact between the material surface and blood. It can be seen that the absorbance value of NO@Cu-MOFs@PC coating was always higher than that of Cu-MOFs@PC coating. This means that NO loading enhances the absorbance of the coating, extends the dynamic coagulation time, and improves anti-clotting ability.
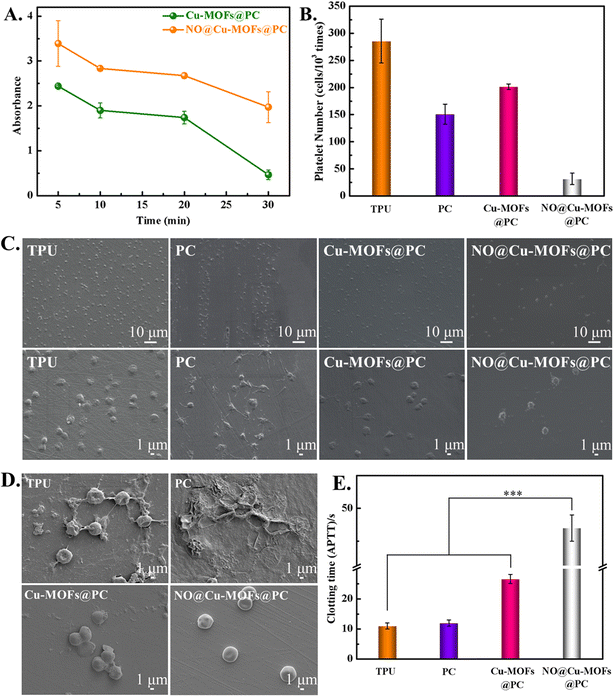 |
| Fig. 7
In vitro anticoagulation evaluation of samples surface. (A) The dynamic coagulation time of the Cu-MOFs@PC and NO@Cu-MOFs@PC coating surface. (B) Number of platelet adhesion statistics. (C) SEM images of platelet adhesion and activation. (D) SEM image of erythrocyte adhesion and activation. (E) In vitro APTT coagulation time test. | |
In order to further verify the anticoagulation property of the as-synthesized coatings on platelets, the adhesion and activation of platelets were evaluated in vitro. The anticoagulation property of the coating was confirmed by analyzing platelet morphologies and adhesion with the use of SEM (Fig. 7C). The platelets in both the TPU and the PC surface were severely activated, presented in a spreading and fully-spreading state, and the pseudopodia could be clearly seen. On the Cu-MOFs@PC coating surface, the number of platelets with a fully-spreading state and the pseudopodia decreased due to the presence of Cu2+. In contrast, the number of platelets adhering to the NO@Cu-MOFs@PC coating surface was significantly lower, and the platelet morphology remained round in shape with little deformation or pseudopodia when NO gas was loaded. The results showed that NO release was an important reason for inhibiting platelet adhesion and activation. This was further demonstrated by the statistics of the activation rate (Fig. 7B). In order to further examine the effects of TPU, PC, Cu-MOFs@PC, and NO@Cu-MOFs@PC on the morphology and number of erythrocytes, in vitro whole blood static culture experiment was performed on each sample, as shown in Fig. 7D. A large number of erythrocytes and fibrinogen adhered to the surfaces of TPU and PC, and the erythrocytes were deformed, indicating that the TPU and PC surfaces were highly susceptible to coagulation. The Cu-MOFs@PC coating had some anti-adhesive properties due to the introduction of Cu2+, thus the surface coagulation was reduced, but some fibrinogen and erythrocytes were still attached. In contrast, the NO@Cu-MOFs@PC coating showed only a small number of erythrocytes and no deformation of erythrocytes, indicating that the coating had good anti-biocontamination properties. This is mainly due to the release of NO from the coating to inhibit the adhesion and deformation of erythrocytes and fibrinogen. This is basically consistent with the results of platelet adhesion and activation experiments.
Further in vitro APTT assays were performed on samples TPU, PC, Cu-MOFs@PC, and NO@Cu-MOFs@PC, and the results are shown in Fig. 7E. The clotting times of TPU and PC were about 10 s, indicating that the hemocompatibility of these two samples was poor. The clotting time of Cu-MOFs@PC coating was significantly longer than that of TPU and PC, indicating that the introduction of Cu-MOFs nanoparticles would improve their hemocompatibility. The clotting time of NO@Cu-MOFs@PC coating was significantly higher than that of Cu-MOFs@PC coating, indicating that NO@Cu-MOFs@PC coating has good hemocompatibility. This was because NO released from the NO@Cu-MOFs@PC coating can bind to endogenous coagulation factors, thereby inhibiting coagulation and prolonging in vitro clotting time.
To further test the anti-thrombogenic capability of the samples, an ex vivo test on New Zealand rabbits without any systemic heparin anticoagulation was used to simulate the interaction of the coating with dynamic whole blood in vivo. All animal procedures were performed in accordance with the Guidelines for Care and Use of Laboratory Animals of “Southwest Jiaotong” University and approved by the Animal Ethics Committee of “The Ethics Committee of Southwest Jiaotong University”. We assembled the TPU, PC, Cu-MOFs@PC, and NO@Cu-MOFs@PC coatings into a Y-shaped tube, as shown in Fig. 8A. After 60 min of ex vivo circulation, the formation of severe thrombosis on the TPU and PC samples, and visible occlusion were found on the cross-section surfaces. Although a small amount of thrombus was detected in the Cu-MOFs@PC coating surface, the vast majority of the area was free of blood clots, suggesting that it also possessed excellent anti-thrombogenic properties. In contrast, no obvious thrombosis or occlusion was observed on the NO@Cu-MOFs@PC coating surface (Fig. 8A). We further evaluated the flow capacity of the circuit to support blood circulation. After the arteriovenous shunt experiment, the flow rate of simulated body fluid with NO@Cu-MOFs@PC coating in the circuit was still greater than 80% (Fig. 8B), significantly higher than that of the TPU and PC samples (25.2 ± 5.0% and 2.3 ± 1.2%, respectively). In addition, the weights of the thrombi on TPU, PC, and Cu-MOFs@PC were apparently higher than those in the NO@Cu-MOFs@PC coating samples (Fig. 8C). Statistical analysis of the occlusions showed 58.0 ± 3.2% lumen loss of TPU, 95.1 ± 1.2% lumen loss of PC coating, and only 10.9 ± 2.1% lumen loss in the NO@Cu-MOFs@PC coating (Fig. 8D). The above results indicate that the NO-loaded biomaterials have good anticoagulant properties. Further observation by SEM indicated that many red blood cells, platelets, and fibrin networks were formed on the sample TPU and PC surfaces, resulting in thrombus formation on the TPU and PC surfaces. Only a few red blood cells and unactivated platelets adhered on the NO@Cu-MOFs@PC coating surface (Fig. 8E). The results of the ex vivo experiment indicated that the NO@Cu-MOFs@PC coating had a dramatic inhibitory effect on thrombosis.
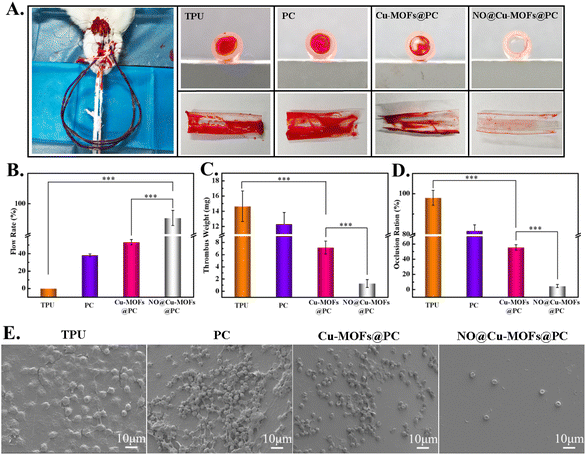 |
| Fig. 8 Thrombogenicity of the samples in an arteriovenous shunt model of the New Zealand rabbit. (A) The ex vivo circulation of the heparinized extracorporeal circulation catheter with the TPU, PC, Cu-MOFs@PC and NO@Cu-MOFs@PC, and photographs of the cross sections of the samples in the catheters after 60 min circulation and the inner wall of the samples. (B) Relative flow rate of simulated body fluid with samples in the catheter at the end of the circulation. (C) Quantitative analysis of the thrombus weight on the surfaces. (D) Percentage of tubing occlusions determined by calculating the loss cross-section diameter. (E) SEM image of thrombi. Data are presented as mean ± SD (n ≥ 4) and analyzed using one-way ANOVA, *p < 0.05, **p < 0.01, ***p < 0.001. | |
3.7 Antibacterial properties of the coatings
As an open indwelling device, the catheter is susceptible to bacterial adhesion and infection. Therefore, the catheter surface must have antibacterial properties. An increased NO-release rate will be needed for obtaining an antibacterial function. The antibacterial activities of pure TPU, PC, Cu-MOFs@PC, and NO@Cu-MOFs@PC coating surfaces were evaluated against E. coli and S. epidermidis using the inhibition zone test, as shown in Fig. 9A. As expected, no inhibition zone was observed for the TPU and PC coating surfaces, verifying that they did not have any antibacterial activity toward either bacteria. However, Cu-MOFs@PC also had no obvious antibacterial effect, which might be due to the wrapping of PC affecting the release of Cu2+ in a short period of time and did not play a bactericidal role. Because similar literature has reported that only when the copper ion concentration reaches 10.1 mg L−1, it has the antibacterial ability, and when it reaches 28.0 mg L−1, it is the minimum inhibitory concentration of copper ions.41,42 In contrast, the NO@Cu-MOFs@PC coating displayed a clear and observable inhibition region without the surrounding bacteria colony growth, and the average diameters of the inhibition zones against E. coli and S. epidermidis were 26 mm and 21 mm, respectively. Likewise, these results can be further confirmed from the area of the inhibition zone on the sample surface, as shown in Fig. 9B. This was mainly because when the NO@Cu-MOFs@PC coating was exposed to the water environment, the unsaturated metal sites and the amino group binding NO can spontaneously release NO into the medium and inhibit the growth of bacteria.43 Because NO is a free radical with a very short half-life (less than 1s half-life), its range of action can only be a very short distance from the surrounding cellular bacteria. Moreover, it can be found that the diameter of inhibition zones against E. coli was larger than that against S. epidermidis. This finding was attributed to the structural difference in the cell wall of Gram-negative bacteria compared to Gram-positive bacteria, that the thick peptidoglycan layer of the S. epidermidis cell wall prevents the penetration of the Cu2+ inside the cytoplasm.44
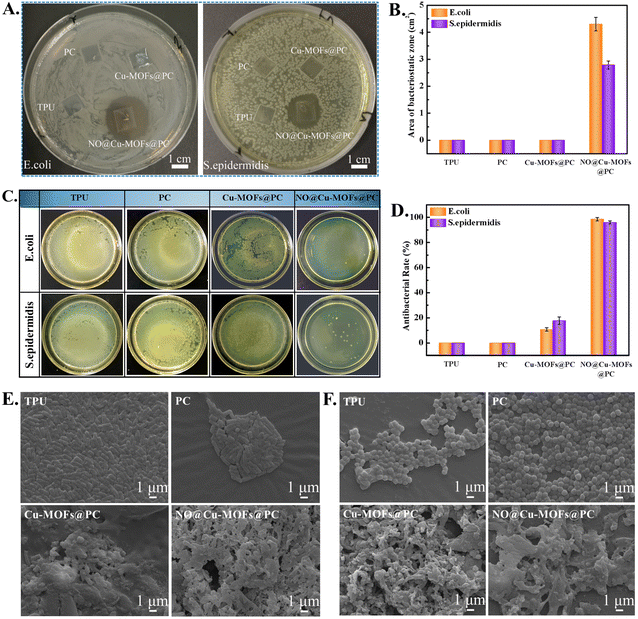 |
| Fig. 9 (A) Inhibition zone of different samples against E. coli and S. epidermidis. (B) The measured the area of the bacteriostatic zone on the sample surfaces. (C) The colony count of the adherent alive bacteria on the samples surface. (D) Antibacterial rate of the samples against E. coli and S. epidermidis. Data are presented as the means ± SD (n ≥ 4). (E) SEM image of E. coli on the surface of each sample. (F) SEM image of S. epidermidis on the surface of each sample. | |
Besides, to further investigate the viability of E. coli and S. epidermidis, the antibacterial ability of the samples was studied by an agar plate colony counting assay. Fig. 9C shows the digital photographs of viable colonies left after incubation with TPU, PC, Cu-MOFs@PC, and NO@Cu-MOFs@PC coatings surface for 8 h. It can be observed that the same results were obtained as those in the inhibition zone test. There was still a large number of colonies on the surfaces of the TPU surface and PC coating surface, and the Cu-MOFs@PC surface only showed weak antibacterial efficacy. On the contrary, the number of bacteria colonies that adhered to the surface of NO@Cu-MOFs@PC was significantly less than that of the TPU, PC, and Cu-MOFs@PC surface, suggesting that the coating had excellent antibacterial activities against both E. coli and S. epidermidis. Moreover, the antibacterial activity rates of NO@Cu-MOFs@PC coating against E. coli and S. epidermidis were about 96%, displaying a high bacteriostasis efficiency. In comparison, the TPU and PC coatings gave an antibacterial rate of 0%, displaying a low bacteriostasis level against E. coli and S. epidermidis, while the Cu-MOFs@PC gave an antibacterial rate of 18–22%, showing a moderate bacteriostasis level against E. coli and S. epidermidis, as shown in Fig. 9D. This may be because copper ions released in the coating will cause the difference in ion concentrations, inside and outside the bacterial cell membrane, destroying the nutrients needed by the cell, and causing cell death. However, due to the small concentration of copper ions released by the coating, it cannot reach the bactericidal concentration (10 mg L−1), and can only have a weak bactericidal effect.41,42
Importantly, in order to further verify the influence of Cu-MOFs and NO on the morphology of bacteria, SEM characterization of bacteria was performed. As shown in Fig. 9E and F, many rod-shaped live E. coli bacteria and spherical-shaped live S. epidermidis with a smooth surface adhered to the surface of the TPU and PC. Compared with the bacterial morphology of the TPU and PC surfaces, the cell membranes of some E. coli and S. epidermidis were partially destroyed and showed apoptosis of bacteria on the surface of the Cu-MOFs@PC coating. Especially, the cell membranes of the attached bacteria in the NO@Cu-MOFs@PC coating surface were partially destroyed and the cytoplasm leaked. The bacterial morphology has visible deformation and collapse, demonstrating that the in situ NO release can severely destroy the captured bacteria.45,46 The results showed that the synergistic action of Cu-MOFs and NO could completely kill bacteria and prolong the service life of the catheter.
3.8 Mechanism analysis
In conclusion, we successfully fabricated Cu-MOFs@PC coating containing amino groups on the medical TPU surface by spin coating. Due to the strong tackiness of the PC solution, it can enhance the adhesion of the Cu-MOFs particles and the TPU substrate, forming a hierarchical structure consisting of multiple layers of Cu-MOFs particles. The further drying process also gives the coating a strong bonding energy with the substrate, which makes the Cu-MOFs@PC coating have excellent stability. In addition, since the coating is a layered structure formed by stacking multilayer Cu-MOF particles, sufficient unsaturated metal sites Cu2+ and amino groups are present. It was easy to load a large amount of NO into the Cu-MOFs@PC coating in a high-pressure environment, as shown in Fig. 10A. In our strategy, NO loading was combined with catalytic NO release for the first time. This is due to the ligand affinity of Cu2+ with NO and the unsaturated metal sites (CUS) of Cu-MOFs to load NO,47,48 as well as the presence of amino groups capable of reacting with NO to form [N(O)NO] functional groups, resulting in the spontaneous release of NO gas in physiological buffers.49,50 Further, we placed the NO@Cu-MOFs@PC coating in PBS solution, and the coating could spontaneously release NO gas in the presence of H+ and water molecules, as shown in Fig. 10B. Since the coating is a dense and uniform layered composite structure inlaid with Cu-MOFs particles. In the water environment, H+ and water molecules preferentially interacted with the exposed NO@Cu-MOFs@PC. After the consumption of Cu-MOFs in the upper layer, the generated vacancies lead to the continuing entry of water molecules and their interaction with the NO@Cu-MOFs particles in the lower layer. Thus, the period of NO-spontaneous release can be greatly extended. In addition, the presence of coordinated Cu2+ can also catalyze the release of NO gas from the endogenous NO donor GSNO in situ by the reaction 2GSNO → 2NO + GSSG,45 thus enabling the coating to release NO effectively in the long term, as shown in Fig. 10C. Compared with similar literature on NO loading by Cu-MOFs,51,52 this initial-release behavior of using the loaded NO to produce a large amount of NO in the aqueous environment first and the sequential-release behavior of using coordinated Cu2+ catalyzed donors to produce NO later could realize the long-term stable release of NO from medical catheters in the implanted body. This method greatly extends the release time of NO on the material surface, with a release period of up to 30 days. The blood compatibility properties of NO@Cu-MOFs@PC coating surface and their effects on the number and morphology of E. coli and S. epidermidis were further analyzed by in vitro and in vivo blood experiments as well as in vitro bacterial experiments. The results of blood compatibility experiments showed that NO released from NO@Cu-MOFs@PC enabled the coating to have excellent anti-platelet adhesion and activation properties, as well as excellent resistance to biological contamination, as shown in Fig. 10D. The results of antibacterial experiments showed that the NO released from NO@Cu-MOFs@PC as well as Cu2+ could not only effectively inhibit the growth of E. coli and S. epidermidis, but also disrupt the cell walls of E. coli and S. epidermidis to release their contents, with efficient antibacterial ability, as shown in Fig. 10E. The reason for the obvious antibacterial effect mainly depends on the sudden release of NO in the first few hours of the coating, which can change the membrane structure of bacteria to achieve the killing effect on the bacteria adhering to the coating. More importantly, the NO released from the loaded NO; the amounts of NO released from the free Cu2+ catalytic donor, and the Cu2+ released are both within the safe physiological range of the human body, which enables the long-term effective use of the implanted device surface.41,48,52 Therefore, the NO@Cu-MOFs@PC coating is a promising material for achieving long-term effective use of a catheter in clinical settings.
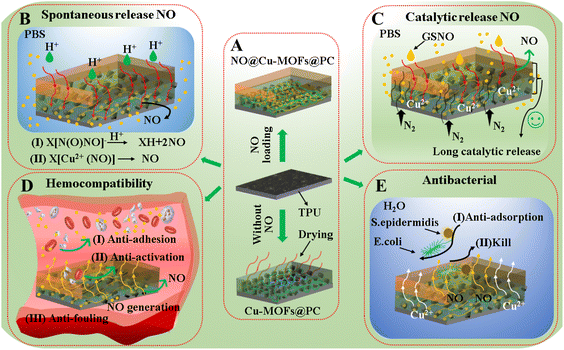 |
| Fig. 10 (A–E) Mechanistic description of mechanical stability, chemical stability, anticoagulation, and antibacterial of NO@Cu-MOFs@PC coating with the layered structure. | |
4. Conclusions
In summary, we successfully prepared a multifunctional robust NO@Cu-MOFs@PC coating with excellent mechanical stability, anti-coagulation, antibacterial, and good biocompatibility on the TPU substrate surface using a spin coating method. The coordination affinity of Cu2+ with NO and the NO loading capacity of the amino group present to easily load large amounts of NO gas in a high-pressure environment. The presence of coordinated Cu2+ in the coating also catalyzes the release of NO from endogenous NO donors in situ, and this sequential release of NO allows for the long-term stable release of NO from the implanted device in the implanted body, with a release cycle of up to 30 days. Furthermore, the results of in vitro and hemi-blood experiments, as well as in vitro antimicrobial assays, showed that the NO@Cu-MOFs@PC coating not only exhibited inhibition of platelet adhesion, activation, and antithrombotic effects but also demonstrated excellent antimicrobial properties against E. coli and S. epidermidis. In conclusion, these results indicate that coating has significant potential for application on the catheter surface and that the problems of poor anticoagulant properties and insufficient antimicrobial properties on the catheter surface can be solved by modifying the implanted device surface.
Author contributions
Jianwen Zhang: Conceptualization, methodology, formal analysis, data curation, writing – original draft, review & editing. Xianlan Ke: Investigation, data curation, methodology. Maotao Huang: Investigation, methodology. Xinyu Pei: Investigation, methodology. Shuai Gao: Methodology. Dimeng Wu: Methodology. Junying Chen: Conceptualization, formal analysis, data curation, writing – review & editing. Yajun Weng: Methodology, formal analysis, data curation, writing – review & editing.
Conflicts of interest
There are no conflicts to declare.
Acknowledgements
This work was financially supported by the National Key Research and Development Program of China (No. 2020YFC1107303), the National Natural Science Foundation of China (No. 31971256), the Natural Science Foundation of Sichuan Province (2022NSFSC0381) and the Fundamental Research Funds for the Central Universities (2682022ZTPY026).
References
- I. H. Jaffer and J. I. Weitz, The blood compatibility challenge. Part 1: blood-contacting medical devices: the scope of the problem, Acta Biomater., 2019, 94, 2–10 CrossRef CAS PubMed.
- L. Nisa, K. Nicoucar and R. Giger, Major bleeding of the upper aerodigestive tract due to oral anticoagulant/antibiotic interactions, Eur. Ann. Otorhinolaryngol. Head Neck Dis., 2013, 130, 153–156 CrossRef CAS.
- H. Yu, S. X. Yu, H. Qiu, P. Gao, Y. Z. Chen, X. Zhao, Q. F. Tu, M. G. Zhou, L. Cai, N. Huang, K. Q. Xiong and Z. L. Yang, Nitric oxide-generating compound and bio-clickable peptide mimic for synergistically tailoring surface anti-thrombogenic and anti-microbial dual-functions, Bioact. Mater., 2021, 6, 1618–1627 CrossRef CAS PubMed.
- Q. F. Tu, X. H. Shen, Y. W. Liu, Q. Zhang, X. Zhao, M. F. Maitz, T. Liu, H. Qiu, J. Wang, N. Huang and Z. L. Yang, A facile metal-phenolic-amine strategy for dual-functionalization of blood-contacting devices with antibacterial and anticoagulant properties, Mater. Chem. Front., 2019, 3, 265–275 RSC.
- Z. Q. Zhu, Q. Gao, Z. Y. Long, Q. Y. Huo, Y. F. Ge, N. Vianney, N. A. Daliko, Y. C. Meng, J. Qu, H. Chen and B. L. Wang, Polydopamine/poly (sulfobetaine methacrylate) Co-deposition coatings triggered by CuSO4 /H2O2 on implants for improved surface hemocompatibility and antibacterial activity, Bioact. Mater., 2021, 6, 2546–2556 CrossRef CAS.
- L. Liu, H. C. Shi, H. Yu, R. T. Zhou, J. H. Yin and S. F. Luan, One-step hydrophobization of tannic acid for antibacterial coating on catheters to prevent catheter-associated infections, Biomater. Sci., 2019, 7, 5035 RSC.
- F. Xie, X. E. Bian, Y. W. Lu, T. Xia, D. D. Xu, Y. F. Wang and J. Cai, Versatile antibacterial surface with amphiphilic quaternized chitin-based derivatives for catheter associated infection prevention, Carbohydr. Polym., 2022, 275, 118683 CrossRef CAS PubMed.
- S. Schulz, M. Maitz, S. Hänsel, L. D. Renner and C. Werner, Analyzing the antiseptic capacity of silver-functionalized poly (ethylene glycol)-heparin hydrogels after human whole blood exposure, Biomater. Sci., 2018, 6, 1129–1139 RSC.
- J. L. Zhou, Z. X. Hu, F. Zabihi, Z. G. Chen and M. F. Zhu, Progress and Perspective of Antiviral Protective Material, Adv. Fiber Mater., 2020, 2, 123–139 CrossRef CAS.
- S. L. Percival, L. Suleman, C. Vuotto and G. Donelli, Healthcare-associated infections, medical devices and biofifilms: risk, tolerance and control, J. Med. Microbiol., 2015, 64, 323–334 CrossRef.
- L. H. Li, C. Chen, C. L. Zhang, R. F. Luo, X. R. Lan, F. Guo, L. Ma, P. Fu and Y. B. Wang, A honokiol-mediated robust coating for blood-contacting devices with anti-inflammatory, antibacterial and antithrombotic properties, J. Mater. Chem. B, 2021, 9, 9770–9783 RSC.
- S. Schwarz, A. Loeffler and K. Kadlec, Bacterial resistance to antimicrobial agents and its impact on veterinary and human medicine, Vet. Dermatol., 2017, 28, 82–119 CrossRef PubMed.
- M. Fischer, M. Vahdatzadeh, R. Konradi, J. Friedrichs, M. F. Maitz, U. Freudenberg and C. Werner, Multilayer hydrogel coatings to combine hemocompatibility and antimicrobial activity, Biomaterials, 2015, 56, 198–205 CrossRef CAS.
- M. Wu, Z. H. Lu, K. K. Wu, C. W. Nam, L. Zhang and J. S. Guo, Recent advances in the development of nitric oxide-releasing biomaterials and their application potentials in chronic wound healing, J. Mater. Chem. B, 2021, 9, 7063–7075 RSC.
- J. Maggie, P. Malone, S. E. Maloney and M. H. Schoenfisch, Nitric Oxide Therapy for Diabetic Wound Healing, Adv. Healthcare Mater., 2019, 8, 1801210 CrossRef.
- S. T. Gregg, Q. C. Yuan, R. E. Morris and B. Xiao, Functionalised Solids Delivering Bioactive Nitric Oxide Gas for Therapeutic Applications, Mater. Today Commun., 2017, 12, 95–105 CrossRef CAS.
- Q. Zhao, Y. H. Fan, Y. Zhang, J. F. Liu, W. J. Li and Y. J. Weng, Copper-Based SURMOFs for Nitric Oxide Generation:Hemocompatibility, Vascular Cell Growth, and Tissue Response, ACS Appl. Mater. Interfaces, 2019, 11, 7872–7883 CrossRef CAS.
- M. J. R. Ahonen, D. J. Suchyta, H. Zhu and M. H. Schoenfisch, Nitric Oxide-Releasing Alginates, Biomacromolecules, 2018, 19, 1189–1197 CrossRef CAS PubMed.
- N. I. Kauser, M. Weisel, Y. L. Zhong, M. M. Lo and A. Ali, Calcium Dialkylamine Diazeniumdiolates: Synthesis, Stability, and Nitric Oxide Generation, J. Org. Chem., 2020, 85, 4807–4812 CrossRef CAS PubMed.
- P. H. Ling, X. P. Gao, X. Y. Sun, P. Yang and F. Gao, Versatile metal-organic frameworks as a catalyst and an indicator of nitric oxide, J. Mater. Chem. B, 2022, 10, 3817–3823 RSC.
- Y. Zhou, T. Yang, R. Namivandi-Zangeneh, C. Boyer, K. Liang and R. Chandrawati, Copper-doped metal-organic frameworks for the controlled generation of nitric oxide from endogenous S-nitrosothiols, J. Mater. Chem. B, 2021, 9, 1059–1068 RSC.
- M. Neidrauer, U. K. Ercan, A. Bhattacharyya, J. Samuels, J. Sedlak, R. Trikha, K. A. Barbee, M. S. Weingarten and S. G. Joshi, Antimicrobial efficacy and wound-healing property of a topical ointment containing nitric-oxide-loaded zeolites, J. Med. Microbiol., 2014, 63, 203–209 CrossRef CAS PubMed.
- B. Xiao, P. S. Wheatley, X. B. Zhao, A. J. Fletcher, S. Fox, A. G. Rossi, I. L. Megson, S. Bordiga, L. Regli, K. Thomas and R. E. Morris, High-capacity hydrogen and nitric oxide adsorption and storage in a metal-organic framework, Chem. Soc., 2007, 129, 1203 CrossRef CAS.
- M. J. Ingleson, R. Heck, J. A. Gould and M. J. Rosseinsky, Nitric Oxide Chemisorption in A Postsynthetically Modified Metal-Organic Framework, Inorg. Chem., 2009, 48, 9986–9988 CrossRef CAS PubMed.
- L. S. Lin, T. Huang, J. Song, X. Y. Ou, Z. Wang, H. Deng, R. Tian, Y. Liu, J. F. Wang, Y. Liu, G. Yu, Z. Zhou, S. Wang, G. Niu, H. H. Yang and X. Chen, Synthesis of copper peroxide nanodots for H2O2 self-supplying chemodynamic therapy, J. Am. Chem. Soc., 2019, 141, 9937–9945 CrossRef CAS.
- M. T. Huang, J. W. Zhang, X. L. Ke, S. Gao, D. M. Wu, J. Y. Chen and Y. J. Weng, Hydrophobic Nano CuMOFs with Stearic Acid modification for NO Loading and Releasing, RSC Adv., 2022, 12, 2383–2390 RSC.
- J. Wang, J. W. Zhang, X. Y. Pei, S. Liu, Y. Li and C. W. Wang, Rapid dipping preparation of closely packed Zn(OH)2@STA nanosheets on cotton fabric and the oil-water separation in various harsh environments, Colloids Surf., A, 2020, 598, 124868 CrossRef CAS.
- J. Chen, D. D. Sheng, T. Ying, H. J. Zhao, J. Zhang, Y. X. Li, H. Xu and S. Y. Chen, MOFs-Based Nitric Oxide Therapy for Tendon Regeneration, Nano-Micro Lett., 2021, 23, 2–17 Search PubMed.
- X. Cui, X. Sun, L. Liu, Q. Huang, H. Yang, C. J. Chen, S. X. Nie and Z. X. Zhao, In-situ fabrication of cellulose foam HKUST-1 and surface modifcation with polysaccharides for enhanced selective adsorption of toluene and acidic dipeptides, Chem. Eng. J., 2019, 369, 898–907 CrossRef CAS.
- J. A. Hrabie and L. K. Keefer, Chemistry of the Nitric Oxide-Releasing Diazeniumdiolate (“Nitrosohydroxylamine”) Functional Group and Its Oxygen-Substituted Derivatives, Chem. Rev., 2002, 102, 1135–1154 CrossRef CAS.
- R. R. Haikal, C. Hua, J. J. Perry, D. O'Nolan, I. Syed, A. Kumar, A. H. Chester, M. J. Zaworotko, M. H. Yacoub and M. H. Alkordi, Controlling the Uptake and Regulating the Release of Nitric Oxide in Microporous Solids, ACS Appl. Mater. Interfaces, 2017, 9, 43520–43528 CrossRef CAS PubMed.
- E. D. Bloch, W. L. Queen, S. Chavan, P. S. Wheatley, J. M. Zadrozny, R. Morris, C. M. Brown, C. Lamberti, S. Bordiga and J. R. Long, Gradual Release of Strongly Bound Nitric Oxide from Fe2(NO)2(dobdc), J. Am. Chem. Soc., 2015, 137, 3466–3469 CrossRef CAS.
- B. Xiao, P. S. Wheatley, X. Zhao, A. J. Fletcher, S. Fox, A. G. Rossi, I. L. Megson, S. Bordiga, L. Regli, K. M. Thomas and R. E. Morris, High-Capacity Hydrogen and Nitric Oxide Adsorption and Storage in a Metal-Organic Framework, J. Am. Chem. Soc., 2007, 129, 1203–1209 CrossRef CAS.
- K. Peikert, L. J. McCormick, D. Cattaneo, M. J. Duncan, F. Hoffmann, A. H. Khan, M. Bertmer, R. E. Morris and M. Froba, Tuning the nitric oxide release behavior of amino functionalized HKUST-1, Microporous Mesoporous Mater., 2015, 216, 118–126 CrossRef CAS.
- J. G. Nguyen, K. K. Tanabe and S. M. Cohen, Postsynthetic diazeniumdiolate formation and NO release from MOFs, CrystEngComm, 2010, 12, 2335–2338 RSC.
- Y. Pan, S. S. Jiang, W. Xiong, D. R. Liu, M. Li, B. He, X. L. Fan and D. Luo, Supported CuO catalysts on metal-organic framework (Cu-UiO-66) for efficient catalytic wet peroxide oxidation of 4-chlorophenol in wastewater, Microporous Mesoporous Mater., 2020, 291, 109703 CrossRef CAS.
- P. Tian, D. Liu, K. X. Li, T. T. Yang, J. J. Wang, Y. Liu and S. Zhang, Porous metal-organic framework Cu3(BTC)2 as catalyst used in air-cathode for high performance of microbial fuel cell, Bioresour. Technol., 2017, 244, 206–212 CrossRef CAS.
- X. L. Gong, R. Zhao, J. Q. Qina, H. M. Wang and D. Wang, Ultra-efficient removal of NO in a MOFs-NTP synergistic process at ambient temperature, Chem. Eng. J., 2019, 358, 291–298 CrossRef CAS.
- B. H. Chen, Y. S. Ma, L. B. Ding, L. S. Xu, Z. F. Wu, Q. Yuan and W. X. Huang, XPS and TPD study of NO interaction with Cu (111): Role of different oxygen species, Chin. J. Catal., 2013, 34, 964–972 CrossRef CAS.
- M. W. Vaughn, L. Kuo and J. C. Liao, Estimation of nitric oxide production and reaction rates in tissue by use of a mathematical model, Am. J. Physiol.: Heart Circ. Physiol., 1998, 274, H2163–H2174 CrossRef CAS.
- Y. R. Yu, G. P. Chen, J. H. Guo, Y. X. Liu, J. A. Ren, T. T. Kong and Y. J. Zhao, Vitamin metal-organic framework-laden microfibers from microfluidics for wound healing, Mater. Horiz., 2018, 5, 1137–1142 RSC.
- D. Y. Fan, Z. Yi, X. Feng, W. Z. Tian, D. K. Xu, A. M. C. Valentino, Q. Wang and H. C. Sun, Antibacterial property of a gradient Cu-bearing titanium alloy by laser
additive manufacturing, Rare Met., 2022, 41, 580–593 CrossRef CAS.
- Y. P. Long, L. Li, T. Xu, X. Z. Wu, Y. Gao, J. B. Huang, C. He, T. Ma, L. Ma, C. Cheng and C. S. Zhao, Hedgehog artifificial macrophage with atomic catalytic centers to combat Drug-resistant bacteria, Nat. Commun., 2021, 12, 6143 CrossRef CAS.
- H. Li, Y. D. Luo, F. Y. Yu and H. M. Zhang, In-situ construction of MOFs-based superhydrophobic /superoleophilic coating on filter paper with self-cleaning and antibacterial activity for efficient oil/water separation, Colloids Surf., A, 2021, 625, 126976 CrossRef CAS.
- Y. Yang, X. Z. Wu, L. Ma, C. He, S. J. Cao, Y. P. Long, J. B. Huang, R. D. Rodriguez, C. Cheng, C. S. Zhao and L. Qiu, Bioinspired Spiky Peroxidase-Mimics for Localized Bacterial Capture and Synergistic Catalytic Sterilization, Adv. Mater., 2021, 33, 2005477 CrossRef CAS.
- W. L. Teo, J. W. Liu, W. Q. Zhou and Y. L. Zhao, Facile preparation of antibacterial MOF-fabric systems for functional protective wearables, SmartMat, 2021, 2, 567–578 CrossRef CAS.
- K. Fontana, L. Ventimiglia and B. Mutus, Nitric Oxide Generating Copper-Chitosan Particles for Wound Healing Applications, J. Chem. Technol. Biotechnol., 2018, 93, 2093–2101 CrossRef CAS.
- Y. H. Fan, Y. Zhang, Q. Zhao, Y. H. Xie, R. F. Luo, P. Yang and Y. J. Weng, Immobilization of nano Cu-MOFs with polydopamine coating for adaptable gasotransmitter generation and copper ion delivery on cardiovascular stents, Biomaterials, 2019, 204, 36–45 CrossRef CAS.
- R. S. Drago, R. O. Ragsdale and D. P. Eyman, A Mechanism for the Reaction of Diethylamine with Nitric Oxide, J. Am. Chem. Soc., 1961, 83, 4337–4339 CrossRef CAS.
- M. Davies, G. Fulton and P. Hagen, Clinical biology of nitric oxide, Br. J. Surg., 1995, 82, 1598–1610 CrossRef CAS.
- B. Xiao, P. S. Wheatley, X. Zhao, A. J. Fletcher, S. Fox, A. G. Rossi, I. L. Megson, S. Bordiga, L. Regli, K. M. Thomas and R. E. Morris, High-capacity hydrogen and nitric oxide adsorption and storage in a metal-organic framework, J. Am. Chem. Soc., 2007, 129, 1203–1209 CrossRef CAS.
- P. J. Zhang, Y. Li, Y. H. Tang, H. Shen, J. K. Li, Z. F. Yi, Q. F. Ke and H. Xu, Copper-Based Metal–Organic Framework as a Controllable Nitric Oxide-Releasing Vehicle for Enhanced Diabetic Wound Healing, ACS Appl. Mater. Interfaces, 2020, 12, 18319–18331 CrossRef CAS PubMed.
|
This journal is © The Royal Society of Chemistry 2023 |
Click here to see how this site uses Cookies. View our privacy policy here.