DOI:
10.1039/D2CS00497F
(Review Article)
Chem. Soc. Rev., 2023,
52, 1672-1696
Emerging ultrasmall luminescent nanoprobes for in vivo bioimaging
Received
30th August 2022
First published on 13th February 2023
Abstract
Photoluminescence (PL) imaging has become a fundamental tool in disease diagnosis, therapeutic evaluation, and surgical navigation applications. However, it remains a big challenge to engineer nanoprobes for high-efficiency in vivo imaging and clinical translation. Recent years have witnessed increasing research efforts devoted into engineering sub-10 nm ultrasmall nanoprobes for in vivo PL imaging, which offer the advantages of efficient body clearance, desired clinical translation potential, and high imaging signal-to-noise ratio. In this review, we present a comprehensive summary and contrastive discussion of emerging ultrasmall luminescent nanoprobes towards in vivo PL bioimaging of diseases. We first summarize size-dependent nano-bio interactions and imaging features, illustrating the unique attributes and advantages/disadvantages of ultrasmall nanoprobes differentiating them from molecular and large-sized probes. We also discuss general design methodologies and PL properties of emerging ultrasmall luminescent nanoprobes, which are established based on quantum dots, metal nanoclusters, lanthanide-doped nanoparticles, and silicon nanoparticles. Then, recent advances of ultrasmall luminescent nanoprobes are highlighted by surveying their latest in vivo PL imaging applications. Finally, we discuss existing challenges in this exciting field and propose some strategies to improve in vivo PL bioimaging and further propel their clinical applications.
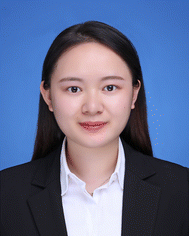
Shihua Li
| Shihua Li received her PhD degree from the College of Chemistry of Fuzhou University (China) in 2019 under the direction of Prof. Huanghao Yang. She is currently a postdoctoral researcher in collaboration with Prof. Yang. Her research interests focus on engineering nanoprobes for biomedical applications such as bioimaging, drug delivery, and theranostics. |
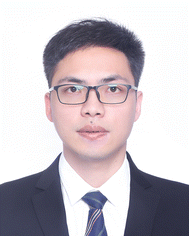
Xiaorong Song
| Xiaorong Song is currently an associate professor at College of Chemistry, Fuzhou University (China). He received his PhD in 2017 from Fuzhou University, and continued his visiting scholar study in National University of Singapore (NUS) and postdoctoral research at Fujian Institute of Research on the Structure of Matter, Chinese Academy of Sciences (China). His research interests focus on luminescent nanomaterials and their biomedical applications, especially on luminescent nanoprobe based disease diagnosis and bioimaging. |
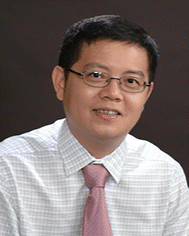
Jianping Xie
| Jianping Xie is currently a Dean's Chair Associate Professor in the Department of Chemical & Biomolecular Engineering, National University of Singapore (NUS). He received his BS and MS degrees in Chemical Engineering from Tsinghua University (China), and his PhD from the Singapore-MIT Alliance (SMA) program. His group is known for the work on engineering water-soluble metal nanoclusters for biomedical and catalytic applications. Dr Xie is a Fellow of the Royal Society of Chemistry (FRSC). |
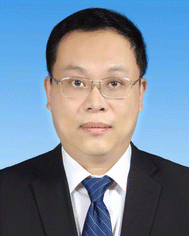
Huanghao Yang
| Huanghao Yang received his PhD from Xiamen University in 2002 and was engaged in postdoctoral research in Hong Kong University of Science and Technology (2002–2004). He joined Fuzhou University in 2008. He has been supported by National Science Foundation for Distinguished Young Scholars of China (2011) and the National Award for the Chang Jiang Scholar Program (2012). His research interests mostly focus on X-ray imaging, analytical chemistry, chemical biology, and nanomedicine. Prof. Yang is a Fellow of the Royal Society of Chemistry. |
1. Introduction
Medical imaging that utilizes imaging scanners to collect in vivo information has greatly advanced the development of diagnosis and therapy of human diseases.1 Among the current nondestructive imaging techniques, photoluminescence (PL) imaging is one of the most important imaging tools in scientific and clinical research, due to its high sensitivity and specificity, multiplexing analysis and real-time dynamic imaging abilities.2,3 As a result, PL imaging is promising for in vivo imaging, which enables the sensitive visualization of targets’ concentration, activity, and other parameters as well as the diverse pathophysiological processes in the living body.4–6
Note that luminescent probes are used in PL imaging and ideally can generate sensitive and stable PL signals upon the specific recognition of certain analytes.7 Organic fluorophores and fluorescent proteins have been widely applied in the design of luminescent probes. However, such conventional probes may face some problems, such as poor physicochemical stability and limited targeting efficacy to biomolecules and tissues.8,9 Currently, luminescent nanoparticles (NPs) have aroused considerable attention in PL imaging because of their enhanced physicochemical stability and customizable surface and optical properties for multifunctional bioimaging.10–12 Despite the exciting advances, in vivo PL imaging still encounters many issues including limited tissue penetration depth, interference of background PL and nonspecific tissue accumulation, and in vivo toxicity concern of luminescent nanoprobes. Therefore, it remains a challenge to establish sensitive and specific PL bioimaging platforms for in vivo clinical applications.13
With the considerable development of synthesis technologies of nanomaterials, sub-10 nm (the size refers to hard size determined using an electron microscope such as a transmission electron microscope) ultrasmall nanoprobes have attracted increasing attention in biological imaging applications (Fig. 1).14,15 This is because ultrasmall nanoprobes generally have a much weaker accumulation in the reticuloendothelial systems (RES) as compared to large-sized NPs, thus efficiently decreasing the background PL in major organs and enhancing the signal-to-noise ratio as well.16–19 Besides, it is feasible to utilize ultrasmall nanoprobes for labelling and tracking in vivo targets without notably affecting their inherent transport traits in the living body. Moreover, ultrasmall nanoprobes can enable some unique in vivo bioapplications, such as PL imaging of kidney dysfunction and dual-modal in vivo PL imaging and in vitro urinalysis, which cannot be performed by large-sized nanoprobes.20–23 Of note, the aforementioned hard size cannot offer dispersion information of NPs in suspension, thus necessitating the determination of hydrodynamic (HD) size by other techniques such as dynamic light scattering (DLS). The HD size takes surface ligands and solvent into account and can sometimes affect the biological behaviors of NPs (e.g., cell uptake, in vivo circulation and elimination, etc.).24 For example, the common prerequisite to allow the renal clearance of NPs is to have HD size approaching (or lower than) the glomerular filtration threshold value (∼5–10 nm). Ultrasmall nanoprobes with HD sizes in this range exhibit efficient renal clearance and low toxicity in vivo, promising future in vivo bioapplications.17,25 Recently, several ultrasmall luminescent nanomaterials have been tailored for in vivo PL imaging, such as quantum dots (QDs), metal nanoclusters (NCs), lanthanide-doped NPs, and silicon NPs.26–32 The developed ultrasmall nanoprobes have been implemented to analyze in vivo diseases – especially cancer-associated hallmarks, such as ions, small molecules, nucleic acids, proteins and enzymes. As such, it is anticipated that the ultrasmall luminescent nanoprobes will serve as potent PL imaging tools and provide a solution to in vivo imaging.33–35 We should note that some specific challenges may also arise in the design and in vivo application of ultrasmall nanoprobes such as insufficient tumor targeting efficacy.
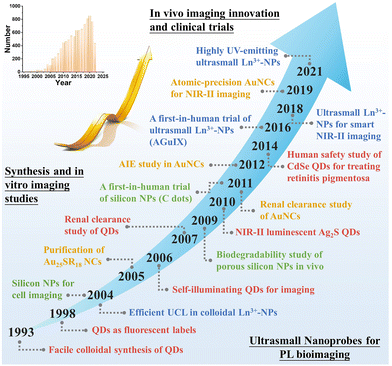 |
| Fig. 1 Timeline of some significant events in the synthesis, bioimaging, and clinical trials of ultrasmall NPs. Inset: Count of paper result by searching “(ultrasmall OR quantum dot OR nanocluster) AND imaging” in Pubmed (obtained on July 27, 2022). | |
In this review, we provide a comprehensive summary of emerging ultrasmall luminescent nanoprobes towards PL imaging of diseases in vivo. Note that there is still a lack of systematic and contrastive discussion on the design and application principles of the emerging ultrasmall luminescent nanoprobes. We first summarize the size-dependent nano-bio interactions to understand the biodistribution, targeting, clearance, and toxicity of NPs. The unique advantages and significant roles of ultrasmall nanoprobes in PL bioimaging in vivo are discussed, in comparison with traditional molecular and large-sized probes. We then detail the basic attributes, rational design methodologies, and in vivo PL imaging applications of four important kinds of ultrasmall luminescent inorganic nanoprobes, including QDs, metal NCs, lanthanide-doped NPs, and silicon (and silica) NPs (as illustrated in Fig. 2). Finally, we highlight the current challenges in this field, and propose potential strategies to improve in vivo PL imaging and propel clinical applications. We believe that this review may offer insights into in vivo PL bioimaging and propel future clinical translation of ultrasmall luminescent nanoprobes.
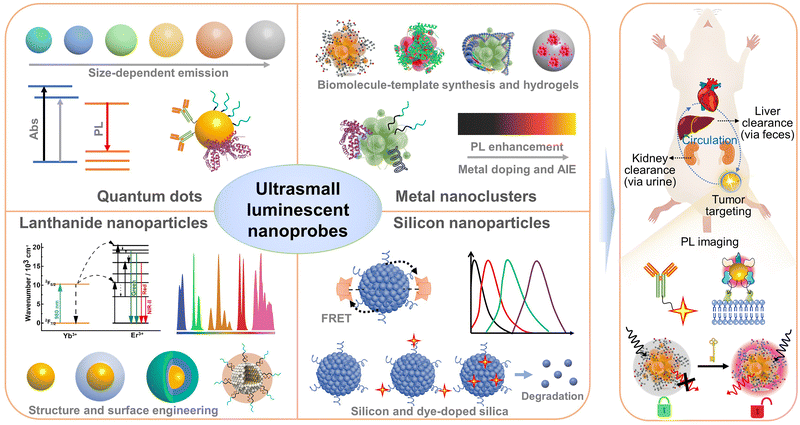 |
| Fig. 2 Schematic illustration of the design of ultrasmall luminescent nanoprobes and their clearance and bioimaging in mice. The synthesis and material structures, key luminescence properties, and surface engineering strategies of these ultrasmall luminescent nanoprobes are shown. After administration in the body, these ultrasmall nanoprobes can possibly be excreted via liver clearance and/or kidney clearance pathways. They can be implemented for diverse bioimaging, such as molecular labeling and tracking, analyte-activated imaging, and cell/tissue-targeted imaging. | |
2. Size-dependent nano-bio interactions
Currently, different kinds of nanoagents have emerged in biological applications and in parallel their performances have been shown to be associated with their size, structure, and surface properties.36–38 Due to these facts, it is important to understand nano-bio interactions prior to biological application studies. We here focus on summarizing and discussing material size effects on nano-bio interactions, including size-dependent in vitro cell uptake, biodistribution, and toxicity issues (Fig. 3).
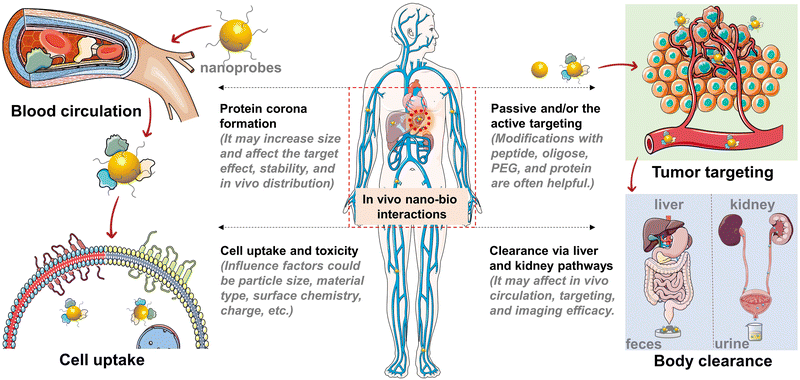 |
| Fig. 3 Schematic illustration of nano-bio interactions that may influence the in vivo pharmacokinetics, cell uptake, tumor targeting, and body clearance of nanoprobes. The formation of protein corona on NPs and its effect on cell uptake are also shown. Note that disease targeting and body clearance properties are two crucial factors determining the in vivo imaging performance and clinical translation potential of nanoprobes. Pictures were adapted with permission from Servier Medical Art by Servier (https://smart.servier.com/), licensed under a Creative Commons Attribution 3.0 Unported License. | |
2.1 Size-dependent in vitro cell uptake efficacy
Efficient cell uptake of NPs is a prerequisite for exerting desired biological imaging and therapy functions. Significantly, nanoagents can enter into the cells via multiple endocytosis pathways, while most small molecules (<1 kDa) penetrate into cells via simple diffusion, and macromolecules may have low endocytosis efficiency, with the exception of those showing strong binding to the receptors and/or cell membrane such as folate, transferrin, cell penetrating peptides, and so on.39–41 This has also inspired the use of NPs for delivering cargos (such as drugs, nucleic acids, proteins, etc.) into cells.37 However, it is difficult to achieve specific uptake of NPs by certain cell types due to their strong nonspecific adhesion and binding to the cell membrane.37 Functionalization of targeting agents is a popular strategy to impart specific cellular uptake and subcellular organelle targeting abilities to NPs.42 One should note that cell uptake is not always wanted, as in the case of cell membrane labeling.43–45 In this context, conferring NPs with controllable uptake efficiency and intracellular location is one of the important objectives.
In the past few years, impressive efforts have been dedicated to understanding the effects of nanoparticle size on cell uptake, showing a vital role in the uptake pathway and efficacy of NPs. For example, Chan et al. found that herceptin conjugated gold NPs of hard size ranging from 2 to 100 nm showed a size-dependent internalization and the maximal cell uptake was observed in the 25–50 nm NPs.46 In the case of mesoporous silica NPs (30–280 nm, hard size), Mou et al. also revealed the highest cell uptake occurring in the 50 nm NPs.47 Note that the binding between the ligands of NPs and the receptors on the cell membrane can decrease Gibbs free energy, and result in membrane wrapping around the NPs.48 The membrane-wrapping process is likely acceptable to describe the size-dependent uptake behaviors.49 In this model, large-sized NPs have more ligand-to-receptor interaction per particle than that in the small NPs, thus exhibiting higher cell uptake efficacy. However, the cell membrane may have receptor shortage and be unable to bind many NPs of >50 nm (hard size). The optimal size of NPs for cell uptake is determined as 30–50 nm.50 In fact, there are currently some available methods to quantify the ligand number and density on NPs, which may provide further understanding on the size effect on cell uptake.51–53 Cell uptake of ultrasmall NPs has also been preliminarily studied. For instance, Liang et al. prepared tiopronin-coated Au NPs with hard sizes of 2–15 nm, and found size-dependent cell uptake efficacy in MCF-7 cells. Especially, Au NPs of 2 nm showed a higher cell uptake than 6 nm and 15 nm NPs.54 Inconsistent with the results, the cell uptake efficacy of cationic Au NPs was found to be elevated with increasing hard size from 2, 4, to 6 nm, contrary to the results obtained with both anionic and zwitterionic counterparts.55,56 This suggests that it is hard to obtain consistent trends of the size effect on cell uptake in different kinds of NPs.
Moreover, apart from size, cell uptake of NPs is also affected by other key factors such as surface chemistry, elasticity, and material shape.55,57–59 Even for one particular factor, its effect on cell uptake should be evaluated case by case, similar to the aforementioned size effect on different materials. For instance, tuning elasticity has shown opposite effects on the cell uptake of silica nanocapsules and alginate-encapsulating liposomes.60,61 Interestingly, at a certain particle size, it is possible to control cell uptake by tuning surface chemistry, in which surface ligands can be engineered to possess diverse properties such as density (or surface coverage), hydrophobicity, charge, and targeting motif.62–65 Therefore, cell uptake can be affected by size effect but is dominated by the combined effect of multiple factors.
2.2 Size-dependent protein corona and in vivo targeting
In order to understand the size effects on cell uptake efficacy, and in vivo biodistribution and targeting, the protein corona formation on NPs has attracted much research interest. The interplay between biological proteins and NPs is one of the decisive factors in the cell uptake process and in vivo pharmacokinetics.36,66 When NPs are dispersed in the biological environment, serum proteins can dynamically adsorb on NPs, forming the so-called protein corona.67–70 The protein corona can seriously affect the physicochemical properties, in vitro cell uptake, and in vivo fate of the NPs. For example, protein corona can facilitate or inhibit the binding of NPs to receptors on the cell membrane, thus affecting the cell–NP interactions.71,72 The active targeting efficacy of functionalized NPs in vivo could be decreased due to the protein corona-induced rapid clearance and the shielding of surface targeting ligands.73,74 Interestingly, recent studies showed that protein corona can also be beneficial for improving blood circulation and targeting by recruiting specific proteins.75–77 Increasing the size of NPs has generally led to the increase of the binding constant and amount of proteins on NPs. Compared to large-sized NPs, ultrasmall NPs have smaller surface areas and permit the formation of only a thin-layer protein corona.78–81 Such a reduced degree of serum protein coverage may retain the targeting capability of surface ligands and antibodies on NPs to a great extent. Even so, a deeper understanding of the protein corona of ultrasmall NPs is needed via experimental and theoretical studies.70,82
In comparison with molecules, NPs show significantly prolonged blood circulation and tissue targeting capability.58,83–85 Particularly, NPs can exhibit passive targeting via the enhanced permeability and retention (EPR) effect and active targeting for enhanced accumulation in tumors.86 However, clinical studies have shown that the EPR effect may not improve the accumulation of nanosized agents in human solid tumors, despite the success in preclinical animal models.87,88 In addition, NPs are often prone to be sequestered by the RES (e.g., liver, spleen, and bone marrow) after intravenous injection into animals. Similar to in vitro cell uptake, the particle size affects in vivo circulation, targeting and tissue penetration.58,89 For example, larger NPs with a HD size of 20–100 nm often have improved pharmacokinetics and tumor targeting, but show limited tumor penetration ability and serious nonspecific accumulation in the RES, while smaller NPs (<10 nm) often show lower uptake in the RES and enhanced tissue penetration but a possible fast blood clearance. Besides, NPs of >200 nm tend to be accumulated in the spleen.
2.3 Size-dependent general toxicity and clearance pathway
Due to the exposure of main organs and tissues to NPs, it is of importance to guarantee low toxicity of NPs.90,91 Owing to the high specific surface area, NPs have higher biological and chemical reactivity in comparison with bulk materials, resulting in potential toxicity concern.92 One of the main toxicity mechanisms of NPs is the formation of reactive oxygen species (ROS), which cause cell damage by the oxidation of intracellular substances, such as proteins, nucleic acids, and lipids.93 This toxicity effect is likely dependent on the type and concentration of nanomaterials. Besides, nanotoxicity is also associated with the complex interplay between NPs and biomolecules (proteins and enzymes).94 For example, the leaching of metal ions from metal-containing NPs might cause serious cytotoxicity and neurotoxicity. In this case, smaller NPs may have a faster ion release rate than larger NPs and induce higher cytotoxicity.95 With the advance in nanomaterial engineering, the nanotoxicity of NPs can be modulated, and nanotoxicity has also been transformed into an effective therapeutic strategy for tumors (e.g., selective ROS generation in cancer cells).96 After administration in animals, in vivo toxicity is highly associated with the retention time of NPs in organs and tissues.
Nowadays, liver excretion (via faces) and renal clearance (via urine) are acknowledged as the two main pathways for the body clearance of NPs.97–100 In contrast to liver clearance, renal clearance typically has a higher clearance efficiency (>50%) within a shorter period (e.g., 12 h). To allow efficient body excretion via the renal clearance pathway, NPs usually should have HD sizes near or less than the glomerular filtration threshold. In addition, the size cut-off for renal clearance is also dependent on nanoparticle type (e.g., hard or soft materials) and surface properties (e.g., shape, hydrophobicity, and charge).101 For example, several kinds of NPs with a HD size of ∼10 nm were also found to be clearable by the kidney.102,103 For NPs within the 2–6 nm range (HD size), smaller NPs are found to be cleared faster than the larger NPs, which could be due to their weaker interactions with the glomerular basement membrane.101 As such, designing ultrasmall NPs with high renal clearance capability offers a promising solution to decrease long-term in vivo toxicity. This also inspires further exploration of smart large-sized NPs (that show specific degradation or disassembly into renal clearable molecules and ultrasmall NPs) for in vivo imaging application.104,105
3. Ultrasmall nanoprobes versus molecular and large-sized probes
The development of nanophotonics and nanomaterials has triggered the expansion of luminescent nanoprobes for diverse bioapplications. Unlike molecular probes, the surface chemistry of nanoprobes can be finely tailored to fulfill imaging purposes.106,107 Nanoprobes are also able to solve the undesirable stability and optical tuning dilemmas in molecular probes, allowing multiplex imaging of targets.108 Targeting units such as peptides, proteins, and small molecules can be attached to nanoprobes via several available bioconjugation methods.109 This endows nanoprobes with passive and/or active targeting capability in tumors. Due to the specific size regime, ultrasmall nanoprobes fill the gap between molecular and large-sized probes, and sometimes provide a solution to the practical problems in disease targeting.
Even so, ultrasmall nanoprobes may have relatively low uptake in tumors than large-sized NPs due to their molecule-like renal clearance and fast blood clearance.21,110,111 Impressively, some strategies have been presented to augment the tumor accumulation efficacy of ultrasmall nanoprobes.112–115 For example, metal NPs can be synthesized in situ on template proteins (e.g., albumin), which may allow enhanced blood circulation and tumor accumulation effects than small-ligand-capped counterparts (Fig. 4a).116,117 Functionalization of targeting agents (e.g., peptides, antibodies, and aptamers) may also be achievable (Fig. 4b); however, it is a big challenge to functionalize these targeting agents on ultrasmall NPs and maintain their renal clearance property. Currently, there are only a few successful examples such as those based on leveraging small antibodies and antibody fragments (with a molecular weight below ∼40 kDa).118,119 Ultrasmall Au NCs and QDs could also self-assemble into large assemblies or integrate with other materials to form hybrids for increasing accumulation efficacy in the tumor and other desired sites (Fig. 4c).100,120,121 This direction may be helpful to design smart assemblies for tumor-specific imaging and therapy application, which however is still in its infancy. Besides, the resultant assemblies may have increased nonspecific organ accumulation and different body clearance pathways.
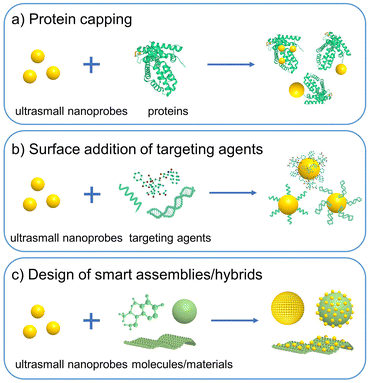 |
| Fig. 4 Strategies to improve the in vivo tumor targeting efficacy of ultrasmall nanoprobes. (a) Protein capping on NPs via in situ synthesis or post-synthesis modification. (b) Surface functionalization with aptamers, peptides, ligands, and antibodies. (c) Designing ultrasmall NP-based smart assemblies and hybrids. | |
Because of low unspecific accumulation in major organs, superior in vivo imaging quality with high signal-to-noise ratio can sometimes be realized by using ultrasmall nanoprobes.12,122 More importantly, ultrasmall nanoprobes may feature a wider scope of in vivo imaging applications than the molecular and large-sized probes. For example, ultrasmall nanoprobes permit some exclusive biological applications, such as in vivo PL bioimaging of kidney dysfunction and bladder-related diseases, and in vivo PL tracking of labeled proteins.123–125 In addition, designing nanoprobes that feature target-activated release of renal clearable ultrasmall NPs offers a new strategy to diagnose in vivo diseases by urinalysis.22,126 Such a methodology has facilitated in vitro multiplex analysis of in vivo targets.
4. Ultrasmall luminescent nanoprobes and in vivo bioimaging
As discussed above, ultrasmall luminescent nanoprobes offer new imaging tools for in vivo bioimaging applications. Currently, several important kinds of ultrasmall inorganic luminescent NPs have been engineered for in vivo PL bioimaging applications. In this section, we summarize the general features, design strategies, and in vivo imaging performance of ultrasmall luminescent nanoprobes based on QDs, metal NCs, lanthanide-doped NPs, and silicon NPs, respectively.
4.1 Quantum dots
4.1.1 General features.
Semiconductor QDs are crucial luminescent tags that have been used in biological diagnosis and imaging since their successful colloidal synthesis.128,129 In 1993, Bawendi and coworkers reported the facile production of nearly monodisperse QDs, inspiring the follow-up synthesis and biomedical application of QDs.130 Due to the size approaching the bulk Bohr exciton radius (namely in the range of 2–10 nm), QDs show unique electrical and optical properties, especially nanocrystal size-dependent energy band gap.131,132 Compared with fluorophores, QDs often exhibit better in vivo imaging performance, due to their narrow and tunable emissions covering from the visible to infrared regions, robust photostability, large absorbance coefficients, and high quantum yields.133 For instance, the PL wavelength of QDs can be readily tuned by controlling particle sizes, compositions, and internal structures.134,135 Currently, researchers have developed a number of approaches to synthesize QDs, including bottom-up methods (e.g., wet chemistry, pyrolysis, hydrothermal methods, etc.) and top-down methods (e.g., electrochemical etching, laser ablation, etc.).136,137 Besides, surface functionalization of QDs can be conducted by diverse modification strategies, such as ligand exchange, amphiphilic polymer coating and silica coating.133,138 Such attributes make QDs an important label for molecular PL imaging in vivo.
Typically, metal chalcogenide QDs are composed of elements from Group II–VI (e.g., CdSe, CdTe, HgTe) and IV–VI (e.g., PbS, PbSe). The earlier works based on these QDs have brought them to the forefront in biomedical imaging. However, heavy metal elements, such as Cd, Hg, and Pb, are rather toxic to cells. Besides, although it is currently a routine to synthesize QDs with near-infrared (NIR) PL (700–900 nm), their excitation wavelength still lies in the visible region (<500 nm), which hinders in vivo practical application. To circumvent such issues, the past years have witnessed the rapid development of core–shell QDs with an inert shell (e.g., ZnS) and low-toxicity QDs without heavy metals, including Group III–V (e.g., InP, InAs), I–VI (e.g., Ag2S, Ag2Se),139 and I–III–VI (e.g., CuInSe, AgInS2). These QDs may have improved in vivo long-term biostability and also extend emission wavelength into the second NIR region (NIR-II, 1000–1700 nm). Over the past few decades, the in vitro and in vivo toxicity effects of QDs have attracted much research, as summarized in several nice reviews.140–142 The toxicity of QDs is mostly caused by the release of metal ions and ROS formation, which are dependent on dosage, composition and surface chemistry properties. Generally, inert shell coating and biomolecule/PEG modifications could be beneficial for reducing the potential toxicity.143
4.1.2 Biodistribution and body clearance.
The in vivo biodistribution fate of QDs is largely dependent on the surface properties (e.g., ligands, charges, and proteins) and particle size.145,146 In 2007, Bawendi and Frangioni et al. studied the size and surface charge effects of QDs on their urinary excretion properties after intravenous injection.127 The QDs were synthesized in an organic phase and coated with zwitterionic or neutral organic ligands to render water dispersibility. The authors found that QDs with a HD diameter of <5.5 nm were effectively cleared into the urine within a few hours (Fig. 5). This study provides a foundation for future exploitation of renal clearable nanoagents for biomedical application. However, the HD size of QDs may be increased (e.g., >10 nm) after surface modification with polymers, proteins, and antibodies. These QD nanoprobes may be sequestrated by the liver and encounter subsequent slow hepatic clearance via the feces.133,147 For example, Wang et al. reported the encapsulation of Ag2S QDs in protein nanocages (PNC) and their further modification with polyethylene glycol (PEGylation), which both led to their major accumulation in the liver, but the blood circulation time was significantly prolonged after PEGylation (Fig. 6).144 Dai et al. also found the size increase of the synthesized QDs from 6.9 nm (hard size) to 18.2 nm (HD size) after PEGylation. The resulting QDs enabled sensitive in vivo imaging of tumor and can be mainly excreted in 72 h via the biliary pathway (Fig. 7).148 In addition, the biological stability of QDs should be considered to avoid potential toxicity of metal ions caused by decomposition.149 Thereby, careful functionalization of QDs with suitable agents is necessary to impart body-clearable and disease-targeted imaging capabilities to QD nanoprobes for improving bioimaging efficacy.
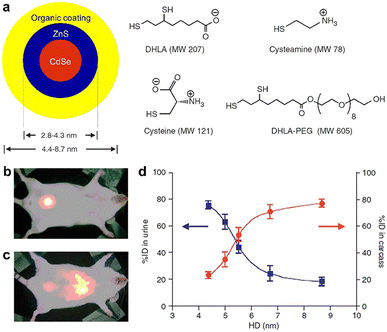 |
| Fig. 5 (a) Sketch map of the core–shell QDs and the chemical structure of the used ligands. (b and c) Overlay of the bright field images and gamma-ray images of mice at 4 h post intravenous injection of 99mTc-QD515 (4.36 nm, HD size) and 99mTc-QD574 (8.65 nm, HD size). (d) Urine excretion (blue curve) and carcass retention (red curve) of 99mTc-QDs with various HD diameters at 4 h after the injection. Adapted with permission.127 Copyright 2007, Springer Nature. | |
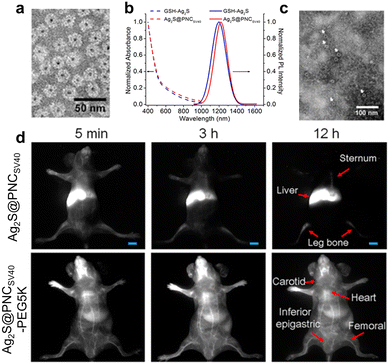 |
| Fig. 6 (a) Negatively stained TEM image of Ag2S-PNC. (b) Absorption and PL spectra of the synthesized nanoprobes. (c) TEM image of Ag2S-PNC after incubation with serum for 2 days. (d) In vivo real-time NIR-II tracking of Ag2S-PNC and PEGylated Ag2S-PNC after intravenous injection. Adapted with permission.144 Copyright 2015, American Chemical Society. | |
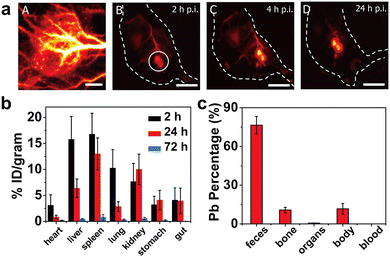 |
| Fig. 7 (a) Time evolution of in vivo tumor imaging of PEGylated PbS/CdS QDs. (b) Biodistribution of QDs at different time after intravenous injection. (c) Excreted percentage of Pb in various parts of mice at 28 d post injection. Adapted with permission.148 Copyright 2018, National Academy of Sciences. | |
4.1.3
In vivo PL bioimaging.
To avoid the potential toxicity of heavy metal ions (e.g., Cd, and Hg) in animals, toxic-metal-free QDs with outer ZnS shells are the ideal choices for bioimaging.131 Importantly, the ZnS shell can also lower the toxicity of Cd-/Pb-based QDs, as evidenced by the minimal in vivo toxicity of CdSe/CdS/ZnS and PbS/CdS/ZnS QDs.150–152 To bypass the possible toxicity of heavy metal ions, Pons et al. synthesized core–shell CuInS2/ZnS QDs with intense emissions centered at 800 nm and a high quantum yield (QY) of 20%, which retained bright fluorescence after dispersing in water and were applied to in vivo sensitive imaging of sentinel lymph nodes.153 The QDs also had a better stability and lower in vivo acute toxicity in comparison with Cd-containing QDs. By contrast, CuInS2 QDs were found to evoke serious in vitro and in vivo toxicity when there was no ZnS shell coating, corroborating the significance of the surface inert shell in reducing toxicity.154 The QY of core–shell CuInS2/ZnS QDs can be further improved to 70–80% by using template and cation exchange synthesis methods.155,156 Upon functionalizing QDs with targeting agents, like peptides, nucleic acids, and antibodies, cancer-targeted PL imaging was realized to visualize in vivo tumors.129,157,158 For instance, Pang and colleagues doped Mn2+ into Ag2Se QDs, conferring QDs with both NIR PL imaging and magnetic resonance imaging abilities.159 The ultrasmall nanoprobes (HD, 4.3 nm) were encapsulated in the cell-derived microvesicles (MVs) for labeling, thereby enabling whole-body tracking of MVs via dual-modal imaging. Also, emission-tunable QDs allow multicolor and multiplex PL imaging by targeting different biomarkers.160,161 In order to improve in vivo imaging specificity, QDs can be engineered with target-activatable linkers (e.g., enzyme-responsive peptides) and energy acceptors (e.g., dyes), thus enabling specific PL imaging of targets via modulating Förster resonance energy transfer between the QDs and acceptors. This strategy has been implemented to image cancer-associated markers, including proteases, ions (e.g., H+, metal ions), small molecules (e.g., reactive oxygen species), and nucleic acids (e.g., mRNA, microRNA).162–165 Interestingly, in 2006, Rao et al. introduced self-illuminating QD conjugates for in vivo multiplex imaging.166 Through the bioluminescence resonance energy transfer between QDs and luciferase, the conjugates emitted bright PL for in vivo imaging with a high signal-to-background ratio. So far, the self-illuminating QD nanoprobes have been developed by several design strategies, providing multifunctional autofluorescence-free tools for in vivo imaging of diverse diseases.167–170
In comparison with PL imaging in the NIR-I region (700–900 nm), NIR-II imaging techniques have realized better imaging sensitivity and resolution in deep tissues, due to significantly reduced light scattering and tissue absorption as well as negligible background PL in such a spectral range. Currently there are several kinds of NIR-II QDs, mainly including PbS, Ag2S, InAs, and CuInS2.171–175 In 2010, Wang et al. first reported the synthesis of monodisperse NIR-II Ag2S QDs with a hard size of 10 nm and PL emission at ∼1058 nm.176 Wang et al. recently developed activatable NIR-II nanoprobes for real-time assessment of the early traumatic brain injury (Fig. 8).177 Bruns et al. exploited the NIR-II-emitting InAs-based core–shell QDs with a QY up to 30%, and demonstrated multifunctional in vivo PL imaging applications, such as quantitative blood flow mapping in brain and tumor microvascular imaging in mice (Fig. 9).178 Importantly, activatable NIR-II QD nanoprobes show high imaging sensitivity and specificity to targets of interest, and are increasingly explored for specific diagnosis of cancer and PL imaging-guided surgery.179,180 Ultrasmall QDs will serve as promising imaging toolkits for in vivo bioimaging, but it may still not be easy to make such multifunctional QD nanoprobes renal clearable currently.
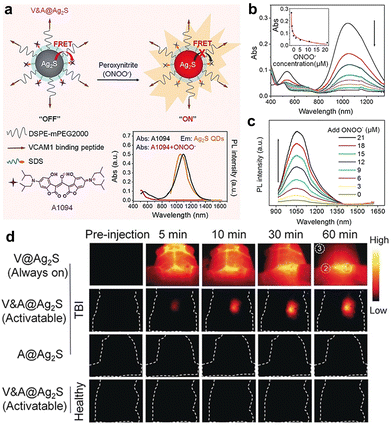 |
| Fig. 8 (a) Schematic diagram of activatable Ag2S QD nanoprobes. (b and c) Absorbance and PL spectra of nanoprobes upon treatment with various concentrations of ONOO−. (d) In vivo imaging performance of nanoprobes in mice with brain vascular injury and control mice, respectively. Adapted with permission.177 Copyright 2019, Wiley-VCH. | |
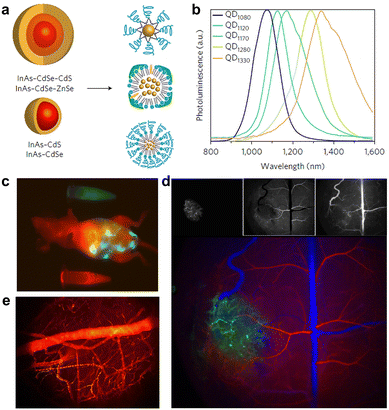 |
| Fig. 9 (a) Schematics of core–shell structures of the designed QDs and surface functionalization with phospholipid micelles (upper), lipoproteins (middle), and emulsified composite QDs (bottom). (b) PL spectra of the synthesized QDs. (c–e) Multicolor whole-body imaging, brain angiograph and blood flow mapping after intravenous injection of QDs, respectively. Adapted with permission.178 Copyright 2017, Springer Nature. | |
4.2 Metal nanoclusters
4.2.1 General features.
Metal NCs, such as Au, Ag, Cu, and Pt NCs, are ultrasmall metal NPs that generally have core sizes below 2 nm.181,182 Herein, we focus on Au NCs in view of their good physicochemical stability and low tissue toxicity. Due to the definitive atom number and molecular formulae, atomically precise metal NCs provide the missing links between the atoms and nanocrystals (>3 nm).183,184 Owing to the ultrasmall size approaching the Fermi wavelength of electrons, Au NCs show discrete electronic states and attractive optical absorption and PL properties. In particular, Au NCs usually feature tunable PL from UV to NIR regions, long lifetime (μs level), large Stokes shift (>100 nm), and high photostability. Such attributes make Au NCs interesting ultrasmall luminescent nanoprobes for biosensing and bioimaging.110,185,186 Despite the general low toxicity, Au NCs also have toxicity concern caused by the induced ROS generation in cells and organs. Especially, protein-protected Au NCs have shown toxicity to liver, kidney, and spleen after long-term accumulation in organs, while no toxicity was observed in small-ligand-capped Au NCs.187,188 Besides, a serious cytotoxicity of ultrasmall Au NPs (1 nm, hard size) capped with organic ligands was observed.189 Therefore, the toxicity of Au NCs is highly related to surface ligands, which might dominate their interaction with cells/biomolecules and the in vivo clearance way.190
Typically, Au NCs are synthesized by reducing metal precursors in the presence of certain protective ligands, such as thiolates, polymers, proteins, and nucleic acids.191–193 Apart from “bottom-up” synthesis strategies, Au NCs can also be obtained through the “top-down” etching strategy. Thiolate ligands are commonly used to synthesize water-soluble metal NCs.194 In 2005, Tsukuda et al. synthesized and purified glutathione (GSH) capped Au NCs, and characterized the classical Au25(SG)18 NCs by using high-resolution electrospray ionization mass spectrometry.195 To prepare high-purity Au NCs, a “size focusing” method has been developed and proven effective for the synthesis of numerous NCs, such as Au25(SR)18,196 Au38(SR)24,197 and Au144(SR)60 NCs,198 which can be applied to versatile thiolate ligands.199 By employing CO gas as a reducing agent, Xie's group developed a facile way to synthesize a series of atomically precise Au NCs.200 Interestingly, thiolate-capped Au NCs can further be modified for diverse bioimaging and therapy applications via several strategies, such as ligand exchange/addition, protein functionalization, and the formation of hybrid nanomaterials.193
Compared with the organic fluorophores and aforementioned QDs, Au NCs (such as thiolate-protected Au NCs) have a relatively low QY (typically <10%).202,203 Additionally, sometimes PL origins of Au NCs remain elusive, which could be due to the lack of enough precise structures and PL information of Au NCs. In order to improve the PL property of Au NCs, great efforts have been devoted into modulating the composition, structure and surface rigidity. For example, Zhu and coworkers introduced the silver doping strategy to dramatically augment the PL QY of Au25 NCs by ∼40%.204 Lee et al. reported the distinct enhancement of Au22(SG)18's QY by rigidifying the Au(I)-thiolate shell with surface bulky groups, and achieved a QY of ∼60%.205 Similar to the aggregation-induced emission (AIE) phenomenon in the organic AIE nanodots, Xie et al. observed the poor solvent-induced aggregation and large PL enhancement in the Au(I)-GSH complexes. Subsequent one-pot synthesis of Au NCs by aggregation of Au(I)-GSH complexes on the in situ generated Au(0) core resulted in a high QY of ∼15%.206 Xie et al. further conducted systematic spectroscopic investigations on a series of Au(I)-GSH complexes and the atomically precise GSH-Au NCs.207 This study reveals the efficient modulation of the PL origins of Au NCs and their emission wavelengths (from the visible to NIR-II regions) by controlling the length of the surface Au(I)-thiolate motif, which provides new insights to control the emission wavelength and intensity of thiolate-protected Au NCs. Apart from the above strategies, Au NCs’ QY can also be enhanced by confinement and self-assembly approaches.208–211
4.2.2 Molecule-like in vivo biodistribution property.
Unlike large-sized NPs, Au NCs with HD sizes of ∼2 nm tend to be quickly cleared out from the body via kidney filtration, similarly to small molecules. In 2011, Zheng et al. first reported the high-efficiency renal clearance (>60%) of luminescent GSH-Au NCs within 24 h after intravenous injection (Fig. 10), while citrate and cysteine protected Au NCs were prone to be accumulated in the RES (especially liver and spleen).201 This reveals a vital role of surface chemistry in dominating the biodistribution fate of Au NCs. Oppositely, molecule-like Au NCs possess a relatively short blood circulation time, which may invalidate the EPR effect for passive tumor targeting. Liu and Zheng found that PEGylation (with a molecular weight of ∼1 kDa) was capable of endowing Au NCs with enhanced tumor targeting, prolonged blood circulation lifetime, and effective renal clearance, in contrast to GSH-Au NCs (Fig. 11).212 Besides, Au NCs can be functionalized with additional agents (e.g., folate, peptides, and antibodies) for active tumor targeting.186 Interestingly, Zheng et al. found that GSH-mediated biotransformation in the liver can modulate the transport of NPs in vivo.213 The indocyanine green (ICG)-modified Au25 NCs were first transported to the liver and then transformed into renal clearable ICG and GSH-Au25 for systemic blood circulation. This resulted in the enhanced tumor accumulation of ICG and GSH-Au25 NCs, offering a strategy to tailor in vivo targeting and clearance of ultrasmall nanoprobes. It should be noticed that the core size, length of the ligand, surface charge and protein corona could also affect the biodistribution of Au NCs.21 For in vivo applications, high-efficiency renal clearance of Au NCs can increase the signal-to-noise ratio in the targeted site after the elimination of off-target NCs.214 Based on these findings, Au NCs could hold great clinic translation potential.22,101
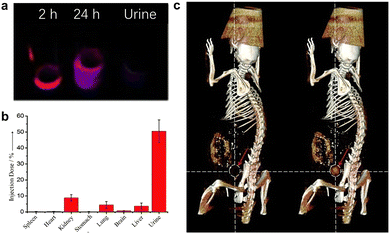 |
| Fig. 10 (a) Time-dependent PL images of urine samples post intravenous injection under UV excitation. (b) Biodistribution of GSH-Au NCs in mice at 24 h post injection. (c) X-ray computed tomography images of mice before (left) and after (right, 30 min) the injection of GSH-Au NCs. Adapted with permission.201 Copyright 2011, Wiley-VCH. | |
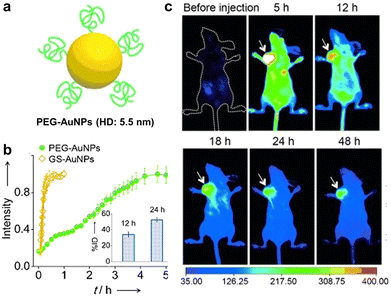 |
| Fig. 11 (a) Schematic illustration of PEG-Au NCs and HD size. (b) Renal clearance kinetics of PEG- and GSH-Au NCs. Inset: PEG-Au NC contents in urine at 12 and 24 h post injection. (c) Time-dependent PL images of mice after injection (arrows indicate the tumor sites). Adapted with permission.212 Copyright 2013, Wiley-VCH. | |
4.2.3
In vivo PL bioimaging.
In the bioimaging field, the unique advantage of Au NCs could be their low toxicity, rapid body clearance, and theranostic functions.215,216 The favorable NIR-emitting Au NCs can be synthesized by directly using peptides and proteins as both capping and active targeting agents. For instance, Kircher and colleagues recently reported a series of emission-tunable Au NCs with HD sizes of ∼2–6 nm that were protected by low molecular mass metalloprotein alpha-lactalbumin (α-LA).217 The Au NCs showed a high tumor accumulation ability for effectively visualizing in vivo breast tumors and guiding the tumor resection process (Fig. 12). Besides, the α-LA-capped Au NCs not only enabled dual-modal bioimaging by integrating X-ray computed tomography with magnetic resonance imaging, but also utilized cytotoxic α-LA-oleic acid complexes for tumor theranostics.
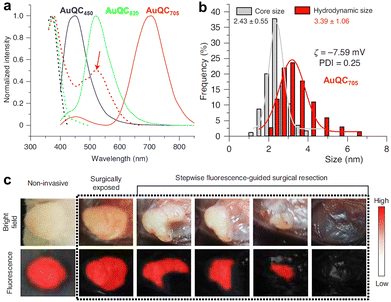 |
| Fig. 12 (a) Excitation (dotted lines) and emission (solid lines; excitation, 360 nm) spectra of emission-tunable Au NCs. (b) Core size, zeta potential, and HD diameter of the 705 nm-emitting Au NCs. (c) PL imaging guided intraoperative surgery of in vivo mouse tumors. Adapted with permission.217 Copyright 2020, Springer Nature. | |
Beyond always-on PL imaging, Au NCs can be assembled with other types of luminescent agents for turn-on or ratiometric imaging of analytes (e.g., ROS).219–222 Additionally, luminescent Au NCs with an efficient renal clearance feature can serve as imaging agents to noninvasively monitor the renal clearance kinetics and image kidney diseases.223,224 To improve the in vivo targeting efficacy to tumors, it is required to tailor the pharmacokinetics and biodistribution of Au NCs, which could be achieved by surface modifications. For example, Liu et al. recently synthesized the charge-reversal luminescent Au NCs protected with thiolate PEG and small ligands, which had optimized pharmacokinetics and pH-regulated HD sizes and surface charges.218 Such nanoprobes enabled high tumor targeting for the selective PL imaging of tiny metastatic tumors in the liver and lungs (Fig. 13). Importantly, Au NCs may retain the HD sizes below 6 nm even after further functionalization of some small-sized agents (e.g., antibody, protein, PEG), while QDs with similar modifications may have overall sizes of >10 nm and couldn’t be renal clearable.
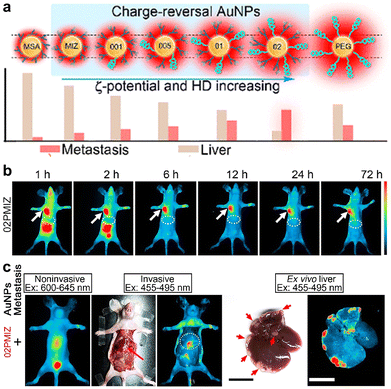 |
| Fig. 13 (a) Schematic of Au NCs with identical core sizes (∼1.7 nm) and different modifications and HD sizes. (b) PL imaging of mice after injection of the optimized Au NCs. (c) Whole-body and ex vivo liver imaging of liver-metastasis-bearing mice at 24 h post injection (arrows show the liver and metastasis sites). Adapted with permission.218 Copyright 2020, American Chemical Society. | |
In recent years, NIR-II PL has been observed in classical thiolate-protected Au25 NCs, which provide better PL imaging contrast than the conventional NIR-I-emitting Au NCs.225,226 The NIR-II PL was found to be enhanced by precise doping of metal ions (such as Zn2+), thus allowing for the sensitive imaging of brain blood flow dynamics and cancer metastasis.227 By using cyclodextrin (CD) as the capping agent, we and colleagues facilely synthesized CD-Au NCs with an intense emission at ∼1050 nm.125 The NCs not only feature efficient renal clearance, but also enable robust protein/antibody labelling via the host–guest interaction for in vivo tracking and tumor-targeted PL imaging (Fig. 14). Also, Li et al. developed the ribonuclease-A capped Au NCs with bright NIR-II emissions for gastrointestinal tract imaging and intestinal tumor diagnosis, due to their outstanding photostability in the gastric environment.228 By virtue of the high renal clearance efficiency and decreased potential toxicity, luminescent Au NCs are increasingly explored as ultrasmall nanoprobes and promise future clinical translation.229,230
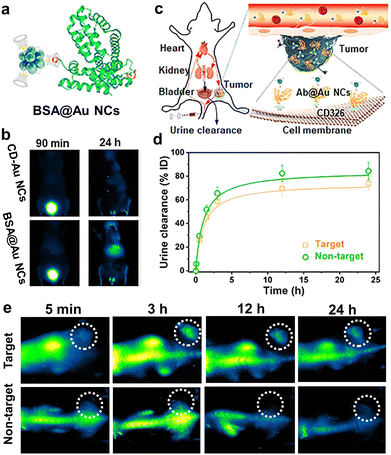 |
| Fig. 14 (a) Sketch map of the BSA@Au NCs via host–guest interaction. (b) Representative PL images of mice after the injection of CD-Au NCs and BSA@Au NCs. (c) Schematic of renal-clearable antibody-modified Au NCs for tumor targeting. (d) Urine clearance properties and (e) PL images of mice after injection of antibody@Au NCs (target) and CD-Au NCs (non-target), respectively. Adapted with permission.125 Copyright 2021, Wiley-VCH. | |
4.3 Lanthanide-doped nanoparticles
4.3.1 General features.
Lanthanides are the important 15 elements covering from lanthanum (atomic number 57) to lutetium (atomic number 71), which are considered as rare earth elements together with scandium and yttrium. Lanthanide ions (Ln3+) have the electron configuration of 4fn5s25p6 (n = 0–14), and emit tunable emissions covering from UV, visible, to NIR regions originating from the 4f–4f transitions.231–234 When doped into suitable host crystalline lattices or coordinating with suitable ligands, Ln3+ have intense, narrow, tunable emissions with good photostability, because of the well-defined and ladder-like energy levels.235–238 In addition, their 4f orbitals are shielded by the filled 5s and 5p orbitals, providing stable and distinguishable spectroscopic signals for PL bioimaging in the biological environment.239,240 Moreover, Ln3+ ions show long-lived luminescence and narrow band emissions, which enable time-resolved optical imaging and multiplex PL imaging.241,242 In 2004, Haase et al. reported the efficient photo upconversion in colloidal lanthanide-doped NaYF4 nanocrystals.243 The development of the core–shell structure and co-doping design further improves the upconversion luminescence QYs of lanthanide-doped nanocrystals.244–247 Significantly, owing to the weakened light scattering and absorption of tissues in the NIR-II regions, lanthanide NPs with NIR-II emissions have currently attracted increasing attention in PL bioimaging. The fantastic upconversion NIR-I PL and down-shifting NIR-II PL upon deep-tissue penetrable light excitation (e.g., 808, 980, 1208 nm, and X-ray sources) make lanthanide nanoprobes a unique multifunctional imaging tool in vivo.248,249
Notably, high-quality lanthanide NPs are generally prepared in organic solvents with surface coating of oleic acid (and/or oleylamine) ligands. It is nowadays a routine to control the nanocrystal size and morphology of lanthanide-doped NPs.252–254 To engineer functional nanoprobes, much efforts have been devoted to conducting surface modifications through silica and polymer coating and surface ligand exchange strategies.255,256 The rich surface lanthanide ions facilitate facile polymer functionalization via both electrostatic interaction and the strong coordination between Ln3+ and carboxyl and amino groups. In comparison with nanoprobes established based on QDs and metal NCs, lanthanide nanoprobes might show advantages in the well-tunable and narrow PL in NIR-I/-II regions and the multiple excitation lights (e.g., NIR lasers and X-rays), which promise broad bioimaging application. However, ultrasmall lanthanide NPs may encounter low luminescence QYs, owing to small absorption cross-section and surface quenching. In 2010, Prasad et al. prepared ultrasmall (7.1 nm, hard size) NaYbF4:2% Tm3+ NPs with high upconversion efficiency than that of classical NaYF4:20% Yb3+, 2% Tm3+ NPs.257 So far, several strategies have been proposed to increase PL efficiency in ultrasmall lanthanide NPs, such as host engineering and energy transfer modulation.257–263 Interestingly, Liu et al. discovered that the UV emissions of ultrasmall (5 nm, hard size) NaGdF4:Yb/Tm NPs can be boosted by about 11
000 folds after coordinating surface ions with bidentate molecules (namely pyridine-2-carboxylic acid), which may induce reconstruction of the orbital hybridization and crystal-field splitting of surface Yb3+.264 In spite of these attempts, studies on highly luminescent ultrasmall lanthanide-doped NPs are still in infancy.265
With regard to toxicity, many studies have been carried out to examine the in vitro and in vivo toxicity of lanthanide NPs, most of which have indicated their low toxicity to cells and animals.266 However, time- and concentration-dependent cytotoxicity was observed in several reports.267 It is also required to consider the nephrotoxicity of Gd3+ and other lanthanide ions. Besides, bare lanthanide NPs were reported to induce serious cytotoxicity due likely to strong interactions with biological molecules such as adenosine triphosphate (ATP).268,269 Nevertheless, lanthanide NPs have shown relatively low in vitro and in vivo toxicity after suitable functionalization (e.g., peptides, proteins, phospholipid shell).270,271
4.3.2
In vivo biodistribution and body clearance.
Owing to the relatively low toxicity and high photo- and chemical-stability, lanthanide NPs are regarded as biocompatible probes.266,272 Different from the hard size below 10 nm of QDs and metal NCs, lanthanide NPs often have sizes between 20 nm and 100 nm to achieve high PL QYs. Lanthanide NPs within such a size range tend to be accumulated in the RES after intravenous injection. In 2010, Li and colleagues studied the in vivo long-term biodistribution of Ln3+-doped NaYF4 NPs (11.5 nm, hard size), showing initial accumulation in the liver and spleen after intravenous injection and gradual body clearance over 115 days (Fig. 15).250 Such behaviors were similar to those of silica coated NaYF4 NPs with observable body clearance after 7 days.273 However, Liu and coworkers observed the long-term body retention (over 3 months) of 30 nm (hard size) NaYF4:Ln NPs coated with polyacrylic acid (PAA) and PEG layers.274 The surface chemistry, particle size, and administration manner therefore can greatly affect the biodistribution and clearance of lanthanide nanoprobes.266
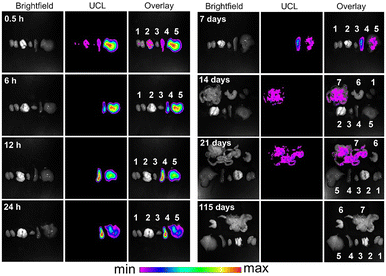 |
| Fig. 15 Real-time ex vivo upconversion PL imaging of mice with the intravenous injection of PAA-lanthanide NPs. Sites 1, 2, 3, 4, 5, 6, and 7 indicate the kidney, lungs, heart, spleen, liver, stomach, and intestine regions, respectively. Adapted with permission.250 Copyright 2010, Elsevier. | |
In the past decade, the excretion pathway of lanthanide NPs has been increasingly researched. For example, Li and coworkers utilized radioisotope labeling to track the biodistribution of lanthanide NPs (hard size, ∼22 nm), revealing high accumulation in the mononuclear phagocyte systems. Only a fraction of lanthanide NPs were excreted into mice feces (<5%) via the hepatic clearance pathway.275 When decreasing particle sizes or changing surface properties, it is feasible to elevate the hepatic clearance efficiency of NPs.276 For instance, Dai et al. reported the surface functionalization of lanthanide NPs with cross-linked hydrophilic polymers, resulting in a notably enhanced hepatic clearance efficiency of ∼90% (Fig. 16).251 Notably, sub-6 nm lanthanide NPs have also been prepared for PL imaging. Zhang's group reported that lanthanide NPs of 5.3 nm HD size can be excreted into both feces (∼45%) and urine (∼30%) at the fourth day post intravenous injection.122 Prasad and colleagues studied the in vivo clearance efficiency of Ln3+-doped NaGdF4 NPs with a HD diameter of ∼5.1 nm. The injected NPs were almost eliminated from the body, showing a liver clearance of 55% and a kidney clearance of 40% over 4 days.277 Overall, ultrasmall lanthanide NPs may be favorable to be excreted via both liver and kidney pathways, while large-sized lanthanide NPs tend to accumulate in the body for a relatively long time. Nevertheless, lanthanide nanoprobes are body-clearable, which can be beneficial for long-term in vivo PL bioimaging.
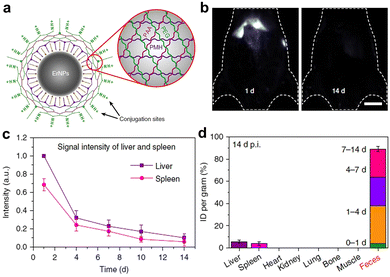 |
| Fig. 16 (a) Sketch map of cross-linking polymer modified hydrophilic lanthanide NPs. (b) PL imaging of mice at 1 d and 14 d post injection of Er3+-doped lanthanide NPs. (c) PL intensity in the liver and spleen post injection. (d) Organ and tissue accumulation at 14 d post injection. The excreted amount of lanthanide NPs in the faeces within different time periods is also shown. Adapted with permission.251 Copyright 2019, Springer Nature. | |
4.3.3
In vivo PL bioimaging.
To construct ultrasmall lanthanide nanoprobes for PL bioimaging, the synthesis of sub-10 nm lanthanide NPs with bright PL is the first essential challenge. Previous studies have realized it by changing the synthetic methodologies, such as the design of ion doping and core–shell structure, which has been reviewed.260,265,278 In 2011, Li et al. reported the synthesis of ultrasmall NaLuF4:Gd/Yb/Tm NPs (HD size, 8.6 nm) with intense upconversion PL, realizing cell labeling and in vivo sensitive upconversion PL tracking.279 Ultrasmall upconversion nanoprobes can also be adopted for multimodal tumor imaging.280,281 In spite of these advances, the upconversion PL efficiency of lanthanide NPs is still far less than that of down-shifting PL. Moreover, NIR-II PL imaging with low background signals and high tissue penetration depth has led to increasing research interest in NIR-II-emitting lanthanide nanoprobes. For example, Zhang et al. synthesized 1064 nm-emitting Nd3+-doped NPs (HD size, 5.3 nm), which can be functionalized with GSH to construct ROS-activatable nanoprobes (Fig. 17).122 The ultrasmall nanoprobes were trapped in inflamed areas subjected to the ROS-triggered crosslinking reaction, achieving precise NIR-II PL imaging of mice inflammation. Meanwhile, other free nanoprobes were able to be excreted into the feces and urine, leading to the high-contrast in vivo PL imaging.
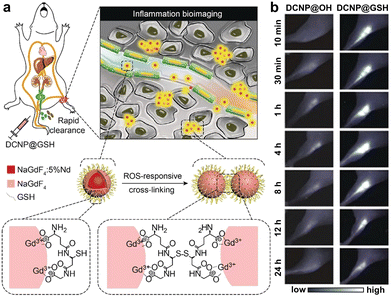 |
| Fig. 17 (a) Schematic illustration of the design and NIR-II PL imaging of inflammation using ultrasmall lanthanide nanoprobes. ROS responsive crosslinking of the nanoprobes is shown. (b) NIR-II PL imaging of mice inflammation after injecting different nanoprobes. Adapted with permission.122 Copyright 2018, Wiley-VCH. | |
Apart from the conventional excitation of UV-vis and NIR lights, lanthanide NPs can also emit PL upon excitation of high-energy rays, such as X-rays and cathode rays.282 Because of the broad clinical use and nearly limitless tissue penetration depth of X-rays, X-ray-activated PL imaging offers a new opportunity for deep-tissue disease diagnosis and further clinical translation.283 In 2011, Xing et al. synthesized small Eu3+-doped NPs with a hard size of 14 nm, and utilized their intense X-ray-excited NIR radioluminescence for in vivo imaging, which showed better imaging contrast than the conventional NIR imaging.284 X-ray-excited NIR-II imaging was also presented through utilizing core–shell Er3+-doped lanthanide NPs, enabling sensitive in vivo visualization of lymph nodes.285 Recently, lanthanide NPs with X-ray-excited NIR-I/-II persistent radioluminescence have been studied, allowing high-contrast imaging in deep tissue.286,287 In addition, it was realized to translate UV-vis Cerenkov luminescence of radioisotopes into NIR lights for in vivo PL imaging by combining with lanthanide NPs.288,289 For example, Grimm et al. integrated the ultrasmall europium-doped NPs (hard size 7 nm, HD size 11 nm) with 89Zr isotopes for simultaneous positron emission tomography (PET) and NIR PL imaging (Fig. 18).31 The original Cerenkov radiation in the UV-vis region can excite lanthanide NPs and emit NIR PL signals to address the limited tissue penetration depth of UV-vis excitation light. Such a design provides new possibilities to improve the practical medical application of the Cerenkov radiation. However, sub-10 nm ultrasmall lanthanide NPs are still rarely explored for in vivo PL bioimaging currently, which might be due to their relatively low PL efficiency.
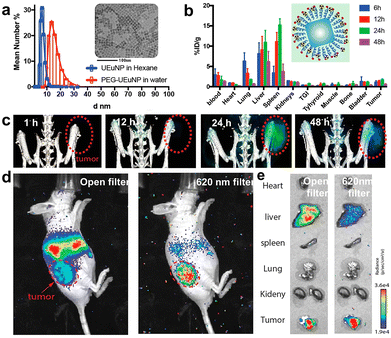 |
| Fig. 18 (a) HD diameters and TEM image of ultrasmall europium-doped NPs. (b) Biodistribution of PEG functionalized 89Zr-europium NPs in tumor-bearing mice after intravenous injection. (c) PET imaging and (d) Cerenkov imaging of tumor-bearing mice. (e) Cerenkov imaging of ex vivo organs. Adapted with permission.31 Copyright 2021, American Chemical Society. | |
4.4 Silicon nanoparticles
4.4.1 General features.
Silicon is considered as the second most abundant element in the earth's crust after oxygen, leading to the wide applications of silicon materials in various fields, including photonics, microelectronics, and biomedicine.290–292 Note that biologically used Si-based nanomaterials mainly include sub-10 nm ultrasmall silicon NPs and non-luminescent silica NPs.293 With the crystal size near bulk-Si exciton Bohr radius (∼4 nm), ultrasmall silicon NPs possess unique optical properties (e.g., PL).294,295 Luminescent silicon NPs usually have low cytotoxicity and tunable PL in the UV-vis and NIR range. Notably, silicon NPs could be biodegraded into metabolizable molecules (e.g., silicic acid) in the biological environment.296–298 This makes silicon NPs very promising for clinical translation. Excitingly, ultrasmall silica NPs have been approved by the U.S. Food and Drug Administration as imaging agents for human clinical trials.26,299
In the last several years, luminescent silicon NPs have been widely utilized for in vitro biodetection and in vivo imaging.298,300,301 The synthesis method can be roughly divided into two main categories, namely “top-down” and “bottom-up” approaches. The former means the crushing of large-sized silicon precursors into silicon NPs by physicochemical treatments, such as chemical etching, laser ablation, thermal decomposition, and mechanical milling.292,302,303 These methods offer a facile strategy to synthesize silicon NPs with tunable emissions, but it needs hazardous agents or treatments, such as high-concentration HF and heat treatments. The alternative bottom-up methods rely on the self-assembly of molecular silicon precursors under microwave and solvent-thermal treatments.304–306 This strategy can obtain silicon NPs with decided surface chemistry by using different precursors.298 Significantly, the crystal size of silicon NPs markedly impacts the PL emission wavelength, lifetime, and QY, due to the PL origin from the recombination of quantum confined charge carriers of silicon.297,299 Silicon NPs are prone to be oxidized and biodegraded in the biological environment,296 which may reduce crystal size and affect PL properties.307,308 Consequently, surface passivation of hydrogen-terminated silicon NPs is necessary for improving their photostability. It has been found that the PL and photostability of silicon NPs were improved by surface coating of organic ligands (e.g., alkene and alkyne ligands).309 For example, Sato et al. coated silicon NPs with silicone elastomer as the passivation layer and improved their photostability in the atmosphere and in various solvents.310 Typically, silicon NPs can be functionalized with polymers and other biological agents via hydrosilylation and functional phospholipid coating.293,311
4.4.2
In vivo biodistribution and toxicity.
Biological toxicity and the in vivo clearance manner are the two key considerations that determine whether nanomaterials can be further used for biomedical applications. Notably, silicon NPs possess lower cytotoxicity compared to heavy metal-containing QDs.312 Although silicon NPs may have ROS generation-associated toxicity at high doses in cells and animals, their good in vivo clearance can greatly raise long-term safety.313 It has also been found that silicon NPs were degraded into silicic acid in vivo and excreted via the kidney.314–316 Sailor et al. reported that luminescent porous silicon NPs were almost biodegraded in biological media within 4 h (>95%). The silicon NPs were accumulated first in the liver and spleen, and then gradually degraded within 4 weeks post injection without causing noticeable in vivo toxicity.314 Ultrasmall silicon NPs of HD size below 5 nm also allow high renal clearance and urine excretion. Louie and Kauzlarich synthesized manganese-doped silicon NPs with hard size below 5 nm and HD diameters ranging from 8 nm to 43 nm depending on the surface coatings.317 These silicon NPs were nontoxic to mammalian cells, and can be labeled with the 64Cu2+ complex for biodistribution analysis via PET imaging. The results revealed their kidney clearance way and partial accumulation in the liver.318 Recently, Cai et al. conjugated ultrasmall silica NPs (HD size, 13.5 nm) with the isotopic pair 90/86Y and systematically tracked their in vivo biodistribution and clearance.16 It was observed that the silica NPs conferred enhanced tumor targeting and rapid hepatobiliary and renal clearance, showing high-contrast tumor imaging performance (Fig. 19).
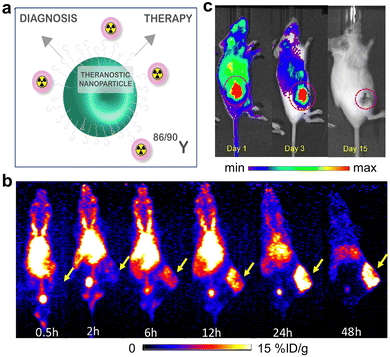 |
| Fig. 19 (a) Sketch map of 90/86Y-labeled silica NPs for diagnosis and therapy applications. (b) Time evolution of PET images of tumor-bearing mice injected with 90/86Y-labeled silica NPs. (c) Time evolution of Cerenkov luminescence images of mice after injection. Adapted with permission.16 Copyright 2021, American Chemical Society. | |
In 2014, a first-in-human clinical trial of silica NPs was reported for tumor diagnosis (Fig. 20).26 In this trial, silica NPs with HD sizes of 6–7 nm were modified with 124I, PEG, and peptides, thus facilitating systematic evaluation of their safety, pharmacokinetics, clearance properties, and cancer targeting efficacy via PET and CT imaging. The silica NPs were renal-clearable, and outperformed the clinical standard radiotracers in imaging sensitivity and specificity of human melanoma. As supported by many studies, silicon/silica NPs significantly hold high clinical translation potential.320,321
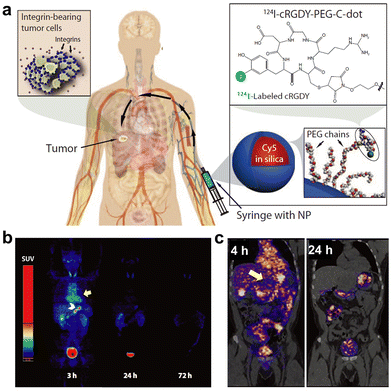 |
| Fig. 20 (a) Schematic illustration of C dots and in vivo imaging. (b) Time-dependent PET imaging of human after intravenous injection of C dots (bladder, heart, and bowel are marked with *, yellow arrow, and white arrowhead, respectively). (c) PET-CT images of human at 4 h and 24 h post injection (the tumor metastasis site is marked). Adapted with permission.26 Copyright 2009, American Association for the Advancement of Science. | |
4.4.3
In vivo luminescence imaging.
In order to prepare silicon nanoprobes, hydrophilic molecules and polymers are frequently employed as surface coating agents during the synthesis or during post-synthesis modification.322,323 In particular, amphiphilic polymers protect silicon NPs from the oxidation and also improve their water dispersibility and in vivo blood circulation life.324,325 Targeting agents, such as peptides, antibodies, and oligosaccharides, can be functionalized on silicon NPs to endow them with specific targeting capability to various diseases.326–328 In 2004, Ruckenstein et al. reported the synthesis of NIR-emitting silicon NPs with relatively high stability for live cell imaging,329 which motivated the increasing exploitation of silicon NPs for PL bioimaging.290,297,330,331 Prasad et al. demonstrated the synthesis of PEGylated silicon NPs with intense and tunable emissions at 450–900 nm.319 The PEGylation and RGD peptide modification can bypass rapid oxidative degradation and RES uptake during blood circulation, thus achieving sensitive PL imaging of sentinel lymph nodes and mouse tumors (Fig. 21). Moreover, PL in the liver and spleen almost disappeared (>95%) after 2 months, indicative of gradual in vivo degradation property. In another attempt, cyclic RGD-conjugated silicon NPs (hard size, ∼4 nm) were designed to specifically label PAT-3 for imaging muscle attachment structures in Caenorhabditis elegans.328 Vancomycin can then be immobilized on silicon NPs to enable in vivo tracking of Staphylococcus aureus infections for a long time (8 days),332 and to rapidly image Gram-positive bacteria-induced keratitis in living mice.333 Based on the strong PL of silicon NPs, He et al. further explored the labeling of exosomes with silicon NPs (SiNPs@EXO) for in vivo imaging.334 Significantly, SiNPs@EXO showed high biosafety and enabled sensitive diagnosis of metastatic lymph nodes (Fig. 22). Also, NIR-II-emitting silicon NPs have been synthesized and applied to in vivo bioimaging. Fujii et al. first synthesized ∼9 nm (hard size) silicon NPs with NIR-II PL centered at ∼1000 nm.335 They further improved NIR-II PL via doping boron and phosphorus, achieving a QY of ∼1.74%.336,337 Taking advantage of the microscale lifetime (>10 μs), luminescent silicon NPs can also be employed for time-gated PL imaging.338
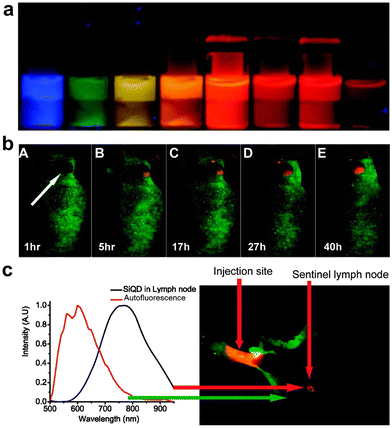 |
| Fig. 21 (a) Photograph of emission-tunable silicon NPs upon 365 nm lamp excitation. (b) Time-dependent PL imaging of Panc-1 tumor-bearing mice after injection of RGD-modified silicon NPs. (c) Sentinel lymph node imaging upon local injection of silicon NPs. Adapted with permission.319 Copyright 2011, American Chemical Society. | |
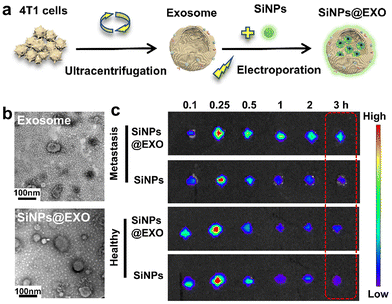 |
| Fig. 22 (a) Schematic illustration of the preparation of the exosome and the silicon NP-labelled exosome (SiNPs@EXO). (b) TEM images of the exosome and SiNPs@EXO. (c) PL imaging of the popliteal lymph nodes in healthy mice and lymphatic metastatic mice at various times post injection. Adapted with permission.334 Copyright 2021, American Chemical Society. | |
Apart from the bioimaging by using the inherent luminescence of silicon NPs, non-luminescent silica NPs can also be explored for in vivo molecular PL imaging by integrating with fluorescent dyes.293,339 This can be achieved by either encapsulating NIR dyes in the silica matrix or modifying them on the surface. In contrast to silicon NPs, dye-doped silica NPs may have richer structures, compositions, and morphology types (e.g., pore structure, shape, dopant, etc.), thus allowing diverse bioapplication scenarios.340 For example, Chen et al. developed fluorescent dye-doped ultrasmall silica NPs (∼6 nm, HD size) for in vivo melanoma-targeted PL imaging and molecular phenotyping of sentinel lymph nodes.341 The ultrasmall silica NPs were modified with Cy5.5 and CW800 dyes to construct two spectrally distinct ultrasmall nanoprobes for multiplex imaging. Therefore, the simultaneous imaging of two tumor-associated biomarkers was capable of identifying the metastatic tumors and their heterogeneity in the nodes. Such an imaging tool facilitates intraoperative imaging guided surgical decision-making and improves surgical efficacy.
In addition, it is feasible to encapsulate the lanthanide chelates and radioisotopes in silica NPs for in vivo PL imaging.293 The long-lived luminescence of lanthanide ions offers the potential for time-resolved in vivo imaging.342,343 Meanwhile, radioisotopes enable Cherenkov luminescence imaging for improving imaging depth and sensitivity in vivo.344 Silicon/silica NPs are therefore very promising nanoprobes for in vivo imaging of diseases, and may be clinically applicable in the near future by virtue of the ongoing clinical trial.
4.5 Other ultrasmall luminescent nanoprobes
Apart from the aforementioned nanoprobes, there are several other kinds of ultrasmall luminescent nanoprobes.33,34 One typical example is the renal clearable ultrasmall dye-doped nanoprobes, such as dye-doped AGuIX (activation and guiding of irradiation by X-rays) NPs, aggregation-induced emission (AIE) nanodots, and nanosized dye hybrids.345–348 Besides, luminescent metal chalcogenide dots (e.g., MoS2, WSe2, etc.), carbon dots, and polymer dots have been explored as renal clearable nanoprobes for in vivo bioimaging of tumors.349–352 The past few years have also witnessed the size reduction of classical luminescent NPs down to the sub-10 nm region. For example, ultrasmall (2.5 nm, hard size) NPs with persistent luminescence were synthesized, enabling the satisfactory persistent luminescence and renal clearance properties for in vivo imaging.353,354 We believe that more and more kinds of ultrasmall luminescent nanoprobes will be emerging with the rapid development in nanoscience.
5. Conclusions and perspectives
In this review, we have presented the pressing demand of exploiting sub-10 nm ultrasmall luminescent nanoprobes for in vivo imaging, and summarized recent advances of PL bioimaging based on various vital kinds of ultrasmall luminescent nanoprobes. We have discussed the size-dependent nano-bio interactions and compared the ultrasmall nanoprobes with conventional molecular fluorophores and large-sized probes. Notably, ultrasmall nanoprobes may have relatively low RES uptake and weak nonspecific accumulation in the body, thus helping to image in vivo diseases of interest with high sensitivity. Recent studies have proven the significance of ultrasmall luminescent nanoprobes in molecule/protein labeling and in vivo tracking of kidney/bladder diseases, which usually cannot be achieved by large-sized nanoprobes. Nevertheless, it is improper to draw the conclusion that ultrasmall nanoprobes outperform large-sized nanoprobes in PL imaging in vivo. With the continued discovery of novel ultrasmall NPs and the design of functional nanoprobes, the sensitivity and selectivity of PL imaging could be further improved. In contrast to molecular and large-sized probes, ultrasmall luminescent nanoprobes have distinct advantages (and disadvantages) and deserve future increasing explorations and clinical trials.
Since the clinical approval of PEGylated liposomal doxorubicin (Doxil) in 1995, considerable efforts have been devoted to developing nanomaterials for cancer imaging and therapy. Hitherto, a number of imaging NPs have been clinically approved or undergoing clinical trials, as exemplified in Table 1.355,356 Notably, a vast majority of the approved imaging nanoagents are implemented for magnetic resonance imaging (MRI), most probably due to the pervasive use of MRI in clinical diagnosis. Some nanoagents for ultrasound imaging are also clinically available. However, there is still a big gap between material engineering and clinical trial/translation of imaging nanoprobes. Regarding PL imaging, one example is the ultrasmall Cornell dots (7 nm, HD size) that have been in clinical trial for tumor-targeted PL imaging and positron emission tomography. Despite high imaging sensitivity and spatial resolution, PL imaging faces some challenges for its clinical applications.357 A major challenge could be the limited penetration depth of PL imaging in the body. Excitingly, the emerging NIR-II imaging has extended tissue penetration depth from millimeters to centimeters. Also, further improvement in the PL QYs of nanoprobes is highly desired. Moreover, PL nanoprobes are capable of realizing surgical navigation, in which the deep tissue imaging is sometimes not necessary.358–360 Additionally, it is still difficult to attain nanoprobes with clinically acceptable toxicity, biodistribution, and clearance properties. Ultrasmall nanoprobes with efficient renal clearance have garnered increasing interest in clinical trials (such as quantum dots as shown in Table 1). Besides, imaging nanoprobes intended for clinical use should be reproducible on a large scale and of high quality. In this context, atomically precise metal NCs have some advantages such as well-defined structures and compositions, which may meet some requirements for quality control of clinical agents. Though a number of imaging nanoprobes have been explored for in vivo PL imaging, these underutilized nanoprobes need further in-depth investigation for advancing clinical translation.
Table 1 Examples of imaging nanoparticles (and microparticles) that have been clinically approved or are undergoing clinical trials
Name |
Material composition |
Identifiers |
Size |
Imaging mode |
Diseases and applications |
Approval and phase status |
Abbreviations: MR, magnetic resonance; US, ultrasound; PET, positron emission tomography; FL, fluorescence; FDA, Food and Drug Administration; EMA, European Medicines Agency; NA, not applicable. The identifiers, phase status, and startup year information were found on ClinicalTrials.gov. |
Feridex (Ferumoxides) |
Fe3O4-γ-Fe2O3 with dextran coating |
NA |
100 nm |
MR |
Imaging of liver lesions |
FDA (1996) Discontinued (2008) |
Resovist |
Fe3O4 with carboxydextran coating |
NA |
60 nm |
MR |
Imaging of liver lesions |
Sweden (2001), discontinued (2009) |
Feraheme (Ferumoxytol) |
Carbohydrate-coated ultra-small superparamagnetic iron oxide |
NA |
17–31 nm |
MR |
Anaemia, and pancreatic cancer |
FDA (2009) |
Ferumoxsil |
Siloxane-coated non-stoichiometric magnetite |
NA |
400 nm |
MR |
Gastrointestinal imaging |
FDA (1996) |
Definity |
Perflutren lipid microsphere |
NA |
1.1–3.3 μm |
US |
Cardiovascular diseases, and prostatic neoplasm |
FDA (2001) |
Optison |
Albumin coated Perflutren lipid microsphere |
NA |
3–4.5 μm |
US |
Echocardiography, and renal cell carcinoma |
FDA (1997), EMA (1998) |
124I-cRGDY-PEG-dots |
124I-labeled cRGDY silica nanoparticle |
NCT01266096 |
∼7 nm |
PET |
Melanoma and malignant brain tumors |
Startup (2011) Phase NA |
Fluorescent cRGDY-PEG-Cy5.5-C dots |
Silica nanoparticles, cRGDY-PEG-Cy5.5 |
NCT02106598 |
∼8 nm |
FL |
Head and neck melanoma; Imaging-guided intraoperative mapping of nodal metastases |
Startup (2014), phase 2 (recruiting) |
64Cu-NOTA-PSMAi-PEG-Cy5.5-C′ dots |
Ultrasmall silica nanoparticles, 64Cu-NOTA-PSMAi-PEG-Cy5.5 |
NCT04167969 |
∼10 nm |
PET/MR |
Prostate cancer |
Startup (2021) Phase 1 (recruiting) |
QDs-VELD |
CdS/ZnS QDs-COOH, veldoreotide (VELD) |
NCT04138342 |
∼10 nm |
FL |
Breast cancer, skin cancer, and skin diseases |
Startup (2019) Phase 1 (recruiting) |
ONM-100 |
Micelle conjugated to indocyanine green |
NCT03735680 |
<100 nm |
FL |
Intraoperative imaging of solid tumors |
Startup (2019) Phase 2 (completed) |
AGuIX |
Polysiloxane Gd-chelate based nanoparticles |
NCT04789486 |
∼3 nm |
MR |
Lung tumors and pancreatic cancer |
Startup (2021) Phase 2 (recruiting) |
Furthermore, regarding the design of ultrasmall luminescent nanoprobes, there are still many challenges to be solved. Firstly, when particle sizes decrease to the sub-10 nm level, the absorption coefficient and PL QYs of NPs may drop sharply. For example, as for lanthanide NPs, the controlled synthesis of sub-10 nm core–shell NPs remains a challenge and their PL QYs and brightness are usually low because of the low absorption coefficient and increased defect quenching. It is highly demanded to develop new strategies for improving the PL QYs and brightness of ultrasmall NPs. Secondly, in order to satisfy the general threshold value of renal clearance, a limited number of agents are available for surface functionalization of ultrasmall nanoprobes. For instance, amphiphilic polymers, biomimetic targeting agents (e.g., cell membrane), and large-sized proteins that may markedly increase HD diameters of ultrasmall nanoprobes are not suitable for surface functionalization. This hampers the design of ultrasmall luminescent nanoprobes and highlights the growing need of new functionalization strategies. Thirdly, although ultrasmall NPs can facilitate rapid renal clearance, their relatively short blood circulation time may weaken in vivo accumulation in the desired tumor sites. Modifying ultrasmall nanoprobes with the specific targeting ligand/antibody will be helpful. This however is still challenging, especially in imaging the orthotopic tumors located in major organs and brain. Designing size-changeable smart assemblies of ultrasmall NPs could be an alternative strategy for tumor targeting, but the clearance pathway of nanoprobes needs more investigation. Fourthly, it is also highly needed to develop commercial mature imaging apparatus to improve imaging performance in large animals. It could be interesting to develop PL imaging techniques that can practically address the unmet needs in clinical disease imaging and therapy. Similar demands lie in establishing standardized in vivo imaging evaluation methods. Fifthly, the long-term in vivo safety of ultrasmall nanoprobes requires more in-depth studies. For example, it is hard to know whether the nanoprobes have side effects on the body after long-term metabolism and transformation.
Nevertheless, increasing studies and advances have showcased that ultrasmall luminescent nanoprobes can offer great opportunities for in vivo PL imaging and clinical application. With the rapid development of nanoprobes and PL imaging techniques, we strongly believe that ultrasmall luminescent nanoprobes will be a powerful engine for the development of the PL bioimaging technique, and may propel realization of precise diagnosis and therapy of diverse diseases in vivo.
Author contributions
S. Li, X. Song, J. Xie, and H. Yang conceived the review, S. Li, J. Wei, Q. Yao, and X. Song drafted the manuscript, Q. Yao helped to revise the structure and content, and X. Song, J. Xie, and H. Yang supervised the project and finalized the manuscript.
Conflicts of interest
There are no conflicts to declare.
Acknowledgements
This work was supported by the National Natural Science Foundation of China (No. 22027805, 22274024 and 22071174), the Major Project of Science and Technology of Fujian Province (No. 2020HZ06006), the Young Elite Scientist Sponsorship Program by CAST (No. YESS20200110), and China Postdoctoral Science Foundation (No. 2022M720737, 2021T140117).
Notes and references
- D. P. Cormode, T. Skajaa, Z. A. Fayad and W. J. Mulder, Arterioscler., Thromb., Vasc. Biol., 2009, 29, 992–1000 CrossRef CAS PubMed.
- M. Schaferling, Angew. Chem., Int. Ed., 2012, 51, 3532–3554 CrossRef PubMed.
- K. D. Wegner and N. Hildebrandt, Chem. Soc. Rev., 2015, 44, 4792–4834 RSC.
- V. Ntziachristos, C. Bremer and R. Weissleder, Eur. Radiol., 2003, 13, 195–208 CrossRef PubMed.
- R. Weissleder and M. Nahrendorf, Proc. Natl. Acad. Sci. U. S. A., 2015, 112, 14424–14428 CrossRef CAS PubMed.
- K. Pu, N. Chattopadhyay and J. Rao, J. Controlled Release, 2016, 240, 312–322 CrossRef CAS PubMed.
- H. S. Peng and D. T. Chiu, Chem. Soc. Rev., 2015, 44, 4699–4722 RSC.
- U. Resch-Genger, M. Grabolle, S. Cavaliere-Jaricot, R. Nitschke and T. Nann, Nat. Methods, 2008, 5, 763–775 CrossRef CAS PubMed.
- K. Zhang, Y. J. Gao, P. P. Yang, G. B. Qi, J. P. Zhang, L. Wang and H. Wang, Adv. Healthcare Mater., 2018, 7, e1800344 CrossRef PubMed.
- D. Kim, N. Lee, Y. I. Park and T. Hyeon, Bioconjugate Chem., 2017, 28, 115–123 CrossRef CAS PubMed.
- J. Zhao, D. Zhong and S. Zhou, J. Mater. Chem. B, 2018, 6, 349–365 RSC.
- W. R. Algar, M. Massey, K. Rees, R. Higgins, K. D. Krause, G. H. Darwish, W. J. Peveler, Z. Xiao, H. Y. Tsai, R. Gupta, K. Lix, M. V. Tran and H. Kim, Chem. Rev., 2021, 121, 9243–9358 CrossRef CAS PubMed.
- X. He, J. Gao, S. S. Gambhir and Z. Cheng, Trends Mol. Med., 2010, 16, 574–583 CrossRef CAS PubMed.
- Z. Li, Q. Sun, Y. Zhu, B. Tan, Z. P. Xu and S. X. Dou, J. Mater. Chem. B, 2014, 2, 2793–2818 RSC.
- K. Zarschler, L. Rocks, N. Licciardello, L. Boselli, E. Polo, K. P. Garcia, L. De Cola, H. Stephan and K. A. Dawson, Nanomedicine, 2016, 12, 1663–1701 CrossRef CAS PubMed.
- C. A. Ferreira, S. Goel, E. B. Ehlerding, Z. T. Rosenkrans, D. Jiang, T. Sun, E. Aluicio-Sarduy, J. W. Engle, D. Ni and W. Cai, Nano Lett., 2021, 21, 4692–4699 CrossRef CAS PubMed.
- X. Jiang, B. Du, Y. Huang and J. Zheng, Nano Today, 2018, 21, 106–125 CrossRef CAS PubMed.
- M. Yu and J. Zheng, ACS Nano, 2015, 9, 6655–6674 CrossRef CAS PubMed.
- Z. H. Hu, W. H. Chen, J. Tian and Z. Cheng, Trends Mol. Med., 2020, 26, 469–482 CrossRef CAS PubMed.
- M. Yu, J. Zhou, B. Du, X. Ning, C. Authement, L. Gandee, P. Kapur, J. T. Hsieh and J. Zheng, Angew. Chem., Int. Ed., 2016, 55, 2787–2791 CrossRef CAS PubMed.
- M. Yu, J. Xu and J. Zheng, Angew. Chem., Int. Ed., 2019, 58, 4112–4128 CrossRef CAS PubMed.
- C. N. Loynachan, A. P. Soleimany, J. S. Dudani, Y. Lin, A. Najer, A. Bekdemir, Q. Chen, S. N. Bhatia and M. M. Stevens, Nat. Nanotechnol., 2019, 14, 883–890 CrossRef CAS PubMed.
- J. G. Huang, C. Xie, X. D. Zhang, Y. Y. Jiang, J. C. Li, Q. L. Fan and K. Y. Pu, Angew. Chem., Int. Ed., 2019, 58, 15120–15127 CrossRef CAS PubMed.
- L. Shang, K. Nienhaus and G. U. Nienhaus, J. Nanobiotechnol., 2014, 12, 5 CrossRef PubMed.
- J. Huang, J. Li, Y. Lyu, Q. Miao and K. Pu, Nat. Mater., 2019, 18, 1133–1143 CrossRef CAS PubMed.
- E. Phillips, O. Penate-Medina, P. B. Zanzonico, R. D. Carvajal, P. Mohan, Y. Ye, J. Humm, M. Gonen, H. Kalaigian, H. Schoder, H. W. Strauss, S. M. Larson, U. Wiesner and M. S. Bradbury, Sci. Transl. Med., 2014, 6, 260ra149 Search PubMed.
- D. Cassano, S. Pocovi-Martinez and V. Voliani, Bioconjugate Chem., 2018, 29, 4–16 CrossRef CAS PubMed.
- W. R. Algar, K. Susumu, J. B. Delehanty and I. L. Medintz, Anal. Chem., 2011, 83, 8826–8837 CrossRef CAS PubMed.
- D. Jiang, Y. Pan, H. Yao, J. Sun, W. Xiong, L. Li, F. Zheng, S. Sun and J. J. Zhu, Anal. Chem., 2022, 94, 9074–9080 CrossRef CAS PubMed.
- S. M. van de Looij, E. R. Hebels, M. Viola, M. Hembury, S. Oliveira and T. Vermonden, Bioconjugate Chem., 2022, 33, 4–23 CrossRef CAS PubMed.
- Q. Zhang, E. C. Pratt, R. Tamura, A. Ogirala, H. T. Hsu, N. Farahmand, S. O'Brien and J. Grimm, Nano Lett., 2021, 21, 4217–4224 CrossRef CAS PubMed.
- Z. Y. Liu, A. A. Liu, H. Fu, Q. Y. Cheng, M. Y. Zhang, M. M. Pan, L. P. Liu, M. Y. Luo, B. Tang, W. Zhao, J. Kong, X. Shao and D. W. Pang, J. Am. Chem. Soc., 2021, 143, 12867–12877 CrossRef CAS PubMed.
- S. Mosleh-Shirazi, M. Abbasi, M. Shafiee, S. R. Kasaee and A. M. Amani, Mater. Today Commun., 2021, 26, 102064 CrossRef CAS.
- R. Q. Yin, X. C. Zhang, J. X. Ge, L. Wen, L. Chen, J. F. Zeng, Z. Li and M. Y. Gao, Part. Part. Syst. Char., 2021, 38, 2000270 CrossRef CAS.
- K. Y. Zheng and J. P. Xie, Trends Chem., 2020, 2, 665–679 CrossRef CAS.
- S. Behzadi, V. Serpooshan, W. Tao, M. A. Hamaly, M. Y. Alkawareek, E. C. Dreaden, D. Brown, A. M. Alkilany, O. C. Farokhzad and M. Mahmoudi, Chem. Soc. Rev., 2017, 46, 4218–4244 RSC.
- J. Mosquera, I. Garcia and L. M. Liz-Marzan, Acc. Chem. Res., 2018, 51, 2305–2313 CrossRef CAS PubMed.
- X. Wang, X. Cui, Y. Zhao and C. Chen, Sci. China: Life Sci., 2020, 63, 1168–1182 CrossRef CAS PubMed.
- J. J. Rennick, A. P. R. Johnston and R. G. Parton, Nat. Nanotechnol., 2021, 16, 266–276 CrossRef CAS PubMed.
- M. Sousa de Almeida, E. Susnik, B. Drasler, P. Taladriz-Blanco, A. Petri-Fink and B. Rothen-Rutishauser, Chem. Soc. Rev., 2021, 50, 5397–5434 RSC.
- S. Zhang, H. Gao and G. Bao, ACS Nano, 2015, 9, 8655–8671 CrossRef CAS PubMed.
- A. C. Marques, P. J. Costa, S. Velho and M. H. Amaral, J. Controlled Release, 2020, 320, 180–200 CrossRef CAS PubMed.
- H. Zhu, L. Gao, X. Jiang, R. Liu, Y. Wei, Y. Wang, Y. Zhao, Z. Chai and X. Gao, Chem. Commun., 2014, 50, 3695–3698 RSC.
- H. Liang, K. Yang, Y. Yang, Z. Hong, S. Li, Q. Chen, J. Li, X. Song and H. Yang, Nano Lett., 2022, 22, 9045–9053 CrossRef CAS PubMed.
- M. Gao, T. Yang, W. Qin, Q. Wang, M. Huang, H. Peng, M. Shao, W. Yao, X. Yi, G. Sun and X. He, Small, 2022, 18, e2204689 CrossRef PubMed.
- W. Jiang, B. Y. Kim, J. T. Rutka and W. C. Chan, Nat. Nanotechnol., 2008, 3, 145–150 CrossRef CAS PubMed.
- F. Lu, S. H. Wu, Y. Hung and C. Y. Mou, Small, 2009, 5, 1408–1413 CrossRef CAS PubMed.
- H. Gao, W. Shi and L. B. Freund, Proc. Natl. Acad. Sci. U. S. A., 2005, 102, 9469–9474 CrossRef CAS PubMed.
- A. Albanese, P. S. Tang and W. C. Chan, Annu. Rev. Biomed. Eng., 2012, 14, 1–16 CrossRef CAS PubMed.
- H. Yuan, J. Li, G. Bao and S. Zhang, Phys. Rev. Lett., 2010, 105, 138101 CrossRef PubMed.
- A. M. Smith, K. A. Johnston, S. E. Crawford, L. E. Marbella and J. E. Millstone, Analyst, 2016, 142, 11–29 RSC.
- A. M. Alkilany, L. Zhu, H. Weller, A. Mews, W. J. Parak, M. Barz and N. Feliu, Adv. Drug Delivery Rev., 2019, 143, 22–36 CrossRef CAS PubMed.
- L. Tong, E. Lu, J. Pichaandi, P. P. Cao, M. Nitz and M. A. Winnik, Chem. Mater., 2015, 27, 4899–4910 CrossRef CAS.
- K. Huang, H. Ma, J. Liu, S. Huo, A. Kumar, T. Wei, X. Zhang, S. Jin, Y. Gan, P. C. Wang, S. He, X. Zhang and X. J. Liang, ACS Nano, 2012, 6, 4483–4493 CrossRef CAS PubMed.
- Y. Jiang, S. Huo, T. Mizuhara, R. Das, Y. W. Lee, S. Hou, D. F. Moyano, B. Duncan, X. J. Liang and V. M. Rotello, ACS Nano, 2015, 9, 9986–9993 CrossRef CAS PubMed.
- M. Liang, I. C. Lin, M. R. Whittaker, R. F. Minchin, M. J. Monteiro and I. Toth, ACS Nano, 2010, 4, 403–413 CrossRef CAS PubMed.
- E. Oh, J. B. Delehanty, K. E. Sapsford, K. Susumu, R. Goswami, J. B. Blanco-Canosa, P. E. Dawson, J. Granek, M. Shoff, Q. Zhang, P. L. Goering, A. Huston and I. L. Medintz, ACS Nano, 2011, 5, 6434–6448 CrossRef CAS PubMed.
- N. Hoshyar, S. Gray, H. Han and G. Bao, Nanomedicine, 2016, 11, 673–692 CrossRef CAS PubMed.
- A. C. Anselmo and S. Mitragotri, Adv. Drug Delivery Rev., 2017, 108, 51–67 CrossRef CAS PubMed.
- Y. Hui, X. Yi, D. Wibowo, G. Yang, A. P. J. Middelberg, H. Gao and C. X. Zhao, Sci. Adv., 2020, 6, eaaz4316 CrossRef CAS PubMed.
- P. Guo, D. Liu, K. Subramanyam, B. Wang, J. Yang, J. Huang, D. T. Auguste and M. A. Moses, Nat. Commun., 2018, 9, 130 CrossRef PubMed.
- L. Gong, Y. Chen, K. He and J. Liu, ACS Nano, 2019, 13, 1893–1899 CrossRef CAS PubMed.
- Y. Wei, N. R. Jana, S. J. Tan and J. Y. Ying, Bioconjugate Chem., 2009, 20, 1752–1758 CrossRef CAS PubMed.
- E. Porret, L. Sancey, A. Martin-Serrano, M. I. Montanez, R. Seeman, A. Yahia-Arnmar, H. Okuno, F. Gomez, A. Ariza, N. Hildebrandt, J. B. Fleury, J. L. Coll and X. Le Guevel, Chem. Mater., 2017, 29, 7497–7506 CrossRef CAS.
- S. Ashraf, J. Park, M. A. Bichelberger, K. Kantner, R. Hartmann, P. Maffre, A. H. Said, N. Feliu, J. Lee, D. Lee, G. U. Nienhaus, S. Kim and W. J. Parak, Nanoscale, 2016, 8, 17794–17800 RSC.
- X. Wang, X. Wang, X. Bai, L. Yan, T. Liu, M. Wang, Y. Song, G. Hu, Z. Gu, Q. Miao and C. Chen, Nano Lett., 2019, 19, 8–18 CrossRef CAS PubMed.
- P. C. Ke, S. Lin, W. J. Parak, T. P. Davis and F. Caruso, ACS Nano, 2017, 11, 11773–11776 CrossRef CAS PubMed.
- M. Hadjidemetriou and K. Kostarelos, Nat. Nanotechnol., 2017, 12, 288–290 CrossRef CAS PubMed.
- M. P. Monopoli, C. Aberg, A. Salvati and K. A. Dawson, Nat. Nanotechnol., 2012, 7, 779–786 CrossRef CAS PubMed.
- R. Cai and C. Chen, Adv. Mater., 2019, 31, e1805740 CrossRef PubMed.
- W. Xiao and H. Gao, Int. J. Pharm., 2018, 552, 328–339 CrossRef CAS PubMed.
- M. Mahmoudi, N. Bertrand, H. Zope and O. C. Farokhzad, Nano Today, 2016, 11, 817–832 CrossRef CAS.
- S. Schottler, K. Landfester and V. Mailander, Angew. Chem., Int. Ed., 2016, 55, 8806–8815 CrossRef PubMed.
- A. K. Barui, J. Y. Oh, B. Jana, C. Kim and J. H. Ryu, Adv. Ther., 2020, 3, 1900124 CrossRef.
- A. Lesniak, A. Salvati, M. J. Santos-Martinez, M. W. Radomski, K. A. Dawson and C. Aberg, J. Am. Chem. Soc., 2013, 135, 1438–1444 CrossRef CAS PubMed.
- P. Del Pino, B. Pelaz, Q. Zhang, P. Maffre, G. U. Nienhaus and W. J. Parak, Mater. Horiz., 2014, 1, 301–313 RSC.
- Q. Xiao, M. Zoulikha, M. Qiu, C. Teng, C. Lin, X. Li, M. A. Sallam, Q. Xu and W. He, Adv. Drug Delivery Rev., 2022, 186, 114356 CrossRef CAS PubMed.
- T. Cedervall, I. Lynch, S. Lindman, T. Berggard, E. Thulin, H. Nilsson, K. A. Dawson and S. Linse, Proc. Natl. Acad. Sci. U. S. A., 2007, 104, 2050–2055 CrossRef CAS PubMed.
- A. Bekdemir and F. Stellacci, Nat. Commun., 2016, 7, 13121 CrossRef CAS PubMed.
- J. Piella, N. G. Bastus and V. Puntes, Bioconjugate Chem., 2017, 28, 88–97 CrossRef CAS PubMed.
- M. M. Yin, P. Dong, W. Q. Chen, S. P. Xu, L. Y. Yang, F. L. Jiang and Y. Liu, Langmuir, 2017, 33, 5108–5116 CrossRef CAS PubMed.
- A. Verma, O. Uzun, Y. Hu, Y. Hu, H. S. Han, N. Watson, S. Chen, D. J. Irvine and F. Stellacci, Nat. Mater., 2008, 7, 588–595 CrossRef CAS PubMed.
- S. D. Li and L. Huang, Mol. Pharm., 2008, 5, 496–504 CrossRef CAS PubMed.
- S. Chen and X. J. Liang, Sci. China: Life Sci., 2018, 61, 371–372 CrossRef PubMed.
- S. H. Li, Y. L. Chen, W. Zhu, W. Yang, Z. W. Chen, J. B. Song, X. R. Song, X. Chen and H. H. Yang, Adv. Funct. Mater., 2021, 31, 2010337 CrossRef CAS.
- M. Bjornmalm, K. J. Thurecht, M. Michael, A. M. Scott and F. Caruso, ACS Nano, 2017, 11, 9594–9613 CrossRef CAS PubMed.
- J. W. Nichols and Y. H. Bae, J. Controlled Release, 2014, 190, 451–464 CrossRef CAS PubMed.
- D. X. Sun, S. Zhou and W. Gao, ACS Nano, 2020, 14, 12281–12290 CrossRef CAS PubMed.
- X. Duan and Y. Li, Small, 2013, 9, 1521–1532 CrossRef CAS PubMed.
- H. C. Fischer and W. C. Chan, Curr. Opin. Biotechnol, 2007, 18, 565–571 CrossRef CAS PubMed.
- S. J. Soenen, P. Rivera-Gil, J. M. Montenegro, W. J. Parak, S. C. De Smedt and K. Braeckmans, Nano Today, 2011, 6, 446–465 CrossRef CAS.
- L. Yildirimer, N. T. Thanh, M. Loizidou and A. M. Seifalian, Nano Today, 2011, 6, 585–607 CrossRef CAS PubMed.
- K. L. Aillon, Y. Xie, N. El-Gendy, C. J. Berkland and M. L. Forrest, Adv. Drug Delivery Rev., 2009, 61, 457–466 CrossRef CAS PubMed.
- H. Arami, A. Khandhar, D. Liggitt and K. M. Krishnan, Chem. Soc. Rev., 2015, 44, 8576–8607 RSC.
- M. Ajdary, M. A. Moosavi, M. Rahmati, M. Falahati, M. Mahboubi, A. Mandegary, S. Jangjoo, R. Mohammadinejad and R. S. Varma, Nanomaterials, 2018, 8, 634 CrossRef PubMed.
- S. Y. Wang, F. Y. Li, X. Hu, M. Lv, C. H. Fan and D. S. Ling, Adv. Ther., 2018, 1, 1800059 CrossRef.
- G. B. Yang, S. Z. F. Phua, A. K. Bindra and Y. L. Zhao, Adv. Mater., 2019, 31, 1805730 CrossRef PubMed.
- B. Wang, X. He, Z. Zhang, Y. Zhao and W. Feng, Acc. Chem. Res., 2013, 46, 761–769 CrossRef CAS PubMed.
- Y. N. Zhang, W. Poon, A. J. Tavares, I. D. McGilvray and W. C. W. Chan, J. Controlled Release, 2016, 240, 332–348 CrossRef CAS PubMed.
- S. H. Li, Q. P. Ma, C. L. Wang, K. D. Yang, Z. Z. Hong, Q. S. Chen, J. B. Song, X. R. Song and H. H. Yang, Anal. Chem., 2022, 94, 2641–2647 CrossRef CAS PubMed.
- B. J. Du, M. X. Yu and J. Zheng, Nat. Rev. Mater., 2018, 3, 358–374 CrossRef.
- L. Cheng, D. Jiang, A. Kamkaew, H. F. Valdovinos, H. J. Im, L. Feng, C. G. England, S. Goel, T. E. Barnhart, Z. Liu and W. Cai, Adv. Funct. Mater., 2017, 27, 1702928 CrossRef PubMed.
- T. Cao, Y. Yang, Y. Sun, Y. Wu, Y. Gao, W. Feng and F. Li, Biomaterials, 2013, 34, 7127–7134 CrossRef CAS PubMed.
- X. Wang, X. Zhong, J. Li, Z. Liu and L. Cheng, Chem. Soc. Rev., 2021, 50, 8669–8742 RSC.
- G. Yang, S. Z. F. Phua, A. K. Bindra and Y. Zhao, Adv. Mater., 2019, 31, e1805730 CrossRef PubMed.
- R. Mout, D. F. Moyano, S. Rana and V. M. Rotello, Chem. Soc. Rev., 2012, 41, 2539–2544 RSC.
- J. Zhang, L. Mou and X. Jiang, Chem. Sci., 2020, 11, 923–936 RSC.
- X. D. Meng, F. Yang, H. F. Dong, L. Dou and X. J. Zhang, Nano Today, 2021, 38, 101156 CrossRef CAS.
- M. Ovais, M. Guo and C. Chen, Adv. Mater., 2019, 31, e1808303 CrossRef PubMed.
- K. Y. Zheng and J. P. Xie, Trends Chem., 2020, 2, 665–679 CrossRef CAS.
- R. Q. Yin, X. C. Zhang, J. X. Ge, L. Wen, L. Chen, J. F. Zeng, Z. Li and M. Y. Gao, Part. Part. Syst. Char., 2021, 38, 2000270 CrossRef CAS.
- X. Luo and J. Liu, Adv. Sci., 2022, 9, e2103971 CrossRef PubMed.
- F. Chen, K. Ma, B. Madajewski, L. Zhuang, L. Zhang, K. Rickert, M. Marelli, B. Yoo, M. Z. Turker, M. Overholtzer, T. P. Quinn, M. Gonen, P. Zanzonico, A. Tuesca, M. A. Bowen, L. Norton, J. A. Subramony, U. Wiesner and M. S. Bradbury, Nat. Commun., 2018, 9, 4141 CrossRef PubMed.
- C. Verry, S. Dufort, B. Lemasson, S. Grand, J. Pietras, I. Tropres, Y. Cremillieux, F. Lux, S. Meriaux, B. Larrat, J. Balosso, G. Le Duc, E. L. Barbier and O. Tillement, Sci. Adv., 2020, 6, eaay5279 CrossRef CAS PubMed.
- X. R. Song, S. H. Li, J. Dai, L. Song, G. Huang, R. Lin, J. Li, G. Liu and H. H. Yang, Small, 2017, 13, 1603997 CrossRef PubMed.
- Y. Cui, J. Yang, Q. Zhou, P. Liang, Y. Wang, X. Gao and Y. Wang, ACS Appl. Mater. Interfaces, 2017, 9, 5900–5906 CrossRef CAS PubMed.
- W. Yang, C. Xiang, Y. Xu, S. Chen, W. Zeng, K. Liu, X. Jin, X. Zhou and B. Zhang, Biomaterials, 2020, 255, 120186 CrossRef CAS PubMed.
- F. Chen, K. Ma, B. Madajewski, L. Zhuang, L. Zhang, K. Rickert, M. Marelli, B. Yoo, M. Z. Turker, M. Overholtzer, T. P. Quinn, M. Gonen, P. Zanzonico, A. Tuesca, M. A. Bowen, L. Norton, J. A. Subramony, U. Wiesner and M. S. Bradbury, Nat. Commun., 2018, 9, 4141 CrossRef PubMed.
- X. Song, W. Zhu, X. Ge, R. Li, S. Li, X. Chen, J. Song, J. Xie, X. Chen and H. Yang, Angew. Chem., Int. Ed., 2021, 60, 1306–1312 CrossRef CAS PubMed.
- M. H. Li, Z. Luo and Y. L. Zhao, Chem. Mater., 2018, 30, 25–53 CrossRef CAS.
- X. D. Lai, L. S. Tan, X. L. Deng, J. B. Liu, A. Q. Li, J. Y. Liu and J. Q. Hu, ACS Appl. Mater. Interfaces, 2017, 9, 5118–5127 CrossRef CAS PubMed.
- M. Zhao, R. Wang, B. Li, Y. Fan, Y. Wu, X. Zhu and F. Zhang, Angew. Chem., Int. Ed., 2019, 58, 2050–2054 CrossRef CAS PubMed.
- P. H. Cheng and K. Y. Pu, Nat. Rev. Mater., 2021, 6, 1095–1113 CrossRef CAS.
- J. G. Huang, Y. Y. Jiang, J. C. Li, S. S. He, J. S. Huang and K. Y. Pu, Angew. Chem., Int. Ed., 2020, 59, 4415–4420 CrossRef CAS PubMed.
- X. Song, W. Zhu, X. Ge, R. Li, S. Li, X. Chen, J. Song, J. Xie, X. Chen and H. Yang, Angew. Chem., Int. Ed., 2021, 60, 1306–1312 CrossRef CAS PubMed.
- J. Huang, X. Chen, Y. Jiang, C. Zhang, S. He, H. Wang and K. Pu, Nat. Mater., 2022, 21, 598–607 CrossRef CAS PubMed.
- H. S. Choi, W. Liu, P. Misra, E. Tanaka, J. P. Zimmer, B. Itty Ipe, M. G. Bawendi and J. V. Frangioni, Nat. Biotechnol., 2007, 25, 1165–1170 CrossRef CAS PubMed.
- M. Bruchez, Jr., M. Moronne, P. Gin, S. Weiss and A. P. Alivisatos, Science, 1998, 281, 2013–2016 CrossRef PubMed.
- X. Michalet, F. F. Pinaud, L. A. Bentolila, J. M. Tsay, S. Doose, J. J. Li, G. Sundaresan, A. M. Wu, S. S. Gambhir and S. Weiss, Science, 2005, 307, 538–544 CrossRef CAS PubMed.
- C. B. Murray, D. J. Norris and M. G. Bawendi, J. Am. Chem. Soc., 1993, 115, 8706–8715 CrossRef CAS.
- A. M. Wagner, J. M. Knipe, G. Orive and N. A. Peppas, Acta Biomater., 2019, 94, 44–63 CrossRef CAS PubMed.
- J. Owen and L. Brus, J. Am. Chem. Soc., 2017, 139, 10939–10943 CrossRef CAS PubMed.
- K. J. McHugh, L. Jing, A. M. Behrens, S. Jayawardena, W. Tang, M. Gao, R. Langer and A. Jaklenec, Adv. Mater., 2018, 30, e1706356 CrossRef PubMed.
- D. J. Norris and M. G. Bawendi, Phys. Rev. B: Condens. Matter Mater. Phys., 1996, 53, 16338–16346 CrossRef CAS PubMed.
- R. E. Bailey and S. Nie, J. Am. Chem. Soc., 2003, 125, 7100–7106 CrossRef CAS PubMed.
- M. A. Farzin and H. Abdoos, Talanta, 2021, 224, 121828 CrossRef CAS PubMed.
- H. R. Chandan, J. D. Schiffman and R. G. Balakrishna, Sens. Actuators, B, 2018, 258, 1191–1214 CrossRef CAS.
- G. Pereira, C. A. P. Monteiro, G. M. Albuquerque, M. I. A. Pereira, M. P. Cabrera, P. E. Cabral, G. A. L. Pereira, A. Fontes and B. S. Santos, J. Braz. Chem. Soc., 2019, 30, 2536–2560 CAS.
- Y. P. Gu, R. Cui, Z. L. Zhang, Z. X. Xie and D. W. Pang, J. Am. Chem. Soc., 2012, 134, 79–82 CrossRef CAS PubMed.
- S. Filali, F. Pirot and P. Miossec, Trends Biotechnol., 2020, 38, 163–177 CrossRef CAS PubMed.
- S. Nikazar, V. S. Sivasankarapillai, A. Rahdar, S. Gasmi, P. S. Anumol and M. S. Shanavas, Biophys. Rev., 2020, 12, 703–718 CrossRef PubMed.
- A. Hoshino, S. Hanada and K. Yamamoto, Arch. Toxicol., 2011, 85, 707–720 CrossRef CAS PubMed.
- H. Sun, F. Zhang, H. Wei and B. Yang, J. Mater. Chem. B, 2013, 1, 6485–6494 RSC.
- C. Li, F. Li, Y. Zhang, W. Zhang, X. E. Zhang and Q. Wang, ACS Nano, 2015, 9, 12255–12263 CrossRef CAS PubMed.
- M. L. Schipper, G. Iyer, A. L. Koh, Z. Cheng, Y. Ebenstein, A. Aharoni, S. Keren, L. A. Bentolila, J. Li, J. Rao, X. Chen, U. Banin, A. M. Wu, R. Sinclair, S. Weiss and S. S. Gambhir, Small, 2009, 5, 126–134 CrossRef CAS PubMed.
- Y. Zhang, Y. Zhang, G. Hong, W. He, K. Zhou, K. Yang, F. Li, G. Chen, Z. Liu, H. Dai and Q. Wang, Biomaterials, 2013, 34, 3639–3646 CrossRef CAS PubMed.
- J. Bourquin, A. Milosevic, D. Hauser, R. Lehner, F. Blank, A. Petri-Fink and B. Rothen-Rutishauser, Adv. Mater., 2018, 30, e1704307 CrossRef PubMed.
- M. Zhang, J. Yue, R. Cui, Z. Ma, H. Wan, F. Wang, S. Zhu, Y. Zhou, Y. Kuang, Y. Zhong, D. W. Pang and H. Dai, Proc. Natl. Acad. Sci. U. S. A., 2018, 115, 6590–6595 CrossRef CAS PubMed.
- E. Oh, R. Liu, A. Nel, K. B. Gemill, M. Bilal, Y. Cohen and I. L. Medintz, Nat. Nanotechnol., 2016, 11, 479–486 CrossRef CAS PubMed.
- L. Ye, K. T. Yong, L. W. Liu, I. Roy, R. Hu, J. Zhu, H. X. Cai, W. C. Law, J. W. Liu, K. Wang, J. Liu, Y. Q. Liu, Y. Z. Hu, X. H. Zhang, M. T. Swihart and P. N. Prasad, Nat. Nanotechnol., 2012, 7, 453–458 CrossRef CAS PubMed.
- T. S. Hauck, R. E. Anderson, H. C. Fischer, S. Newbigging and W. C. W. Chan, Small, 2010, 6, 138–144 CrossRef CAS PubMed.
- S. Jeong, I. Song, W. Lee, Y. M. Ryu, Y. Jung, S. Y. Kim, K. Kim, S. C. Hong, S. J. Myung and S. Kim, Nano Lett., 2017, 17, 1378–1386 CrossRef CAS PubMed.
- T. Pons, E. Pic, N. Lequeux, E. Cassette, L. Bezdetnaya, F. Guillemin, F. Marchal and B. Dubertret, ACS Nano, 2010, 4, 2531–2538 CrossRef CAS PubMed.
- J. C. Kays, A. M. Saeboe, R. Toufanian, D. E. Kurant and A. M. Dennis, Nano Lett., 2020, 20, 1980–1991 CrossRef CAS PubMed.
- C. Xia, J. D. Meeldijk, H. C. Gerritsen and C. de Mello Donega, Chem. Mater., 2017, 29, 4940–4951 CrossRef CAS PubMed.
- L. Li, T. J. Daou, I. Texier, T. K. C. Tran, Q. L. Nguyen and P. Reiss, Chem. Mater., 2009, 21, 2422–2429 CrossRef CAS.
- X. Gao, Y. Cui, R. M. Levenson, L. W. Chung and S. Nie, Nat. Biotechnol., 2004, 22, 969–976 CrossRef CAS PubMed.
- W. Cai and X. Chen, Nat. Protoc., 2008, 3, 89–96 CrossRef CAS PubMed.
- J. Y. Zhao, G. Chen, Y. P. Gu, R. Cui, Z. L. Zhang, Z. L. Yu, B. Tang, Y. F. Zhao and D. W. Pang, J. Am. Chem. Soc., 2016, 138, 1893–1903 CrossRef CAS PubMed.
- J. Liu, S. K. Lau, V. A. Varma, R. A. Moffitt, M. Caldwell, T. Liu, A. N. Young, J. A. Petros, A. O. Osunkoya, T. Krogstad, B. Leyland-Jones, M. D. Wang and S. Nie, ACS Nano, 2010, 4, 2755–2765 CrossRef CAS PubMed.
- B. Liu, B. Jiang, Z. P. Zheng and T. C. Liu, J. Lumin., 2019, 209, 61–68 CrossRef CAS.
- Y. P. Wang, Y. F. Jiang, M. Zhang, J. Tan, J. M. Liang, H. X. Wang, Y. P. Li, H. N. He, V. C. Yang and Y. Z. Huang, Adv. Funct. Mater., 2014, 24, 5443–5453 CrossRef CAS.
- X. Liu, G. B. Braun, M. Qin, E. Ruoslahti and K. N. Sugahara, Nat. Commun., 2017, 8, 343 CrossRef PubMed.
- H. Mattoussi, G. Palui and H. B. Na, Adv. Drug Delivery Rev., 2012, 64, 138–166 CrossRef CAS PubMed.
- D. Geissler and N. Hildebrandt, Anal. Bioanal. Chem., 2016, 408, 4475–4483 CrossRef CAS PubMed.
- M. K. So, C. Xu, A. M. Loening, S. S. Gambhir and J. Rao, Nat. Biotechnol., 2006, 24, 339–343 CrossRef CAS PubMed.
- G. Yang, W. Wang, J. Song and J. Zhang, Nanomedicine, 2021, 16, 1737–1740 CrossRef CAS PubMed.
- X. Sun, X. Huang, J. Guo, W. Zhu, Y. Ding, G. Niu, A. Wang, D. O. Kiesewetter, Z. L. Wang, S. Sun and X. Chen, J. Am. Chem. Soc., 2014, 136, 1706–1709 CrossRef CAS PubMed.
- X. Xu, H. An, D. Zhang, H. Tao, Y. Dou, X. Li, J. Huang and J. Zhang, Sci. Adv., 2019, 5, eaat2953 CrossRef PubMed.
- Q. Li, J. Zeng, Q. Miao and M. Gao, Front. Bioeng. Biotechnol., 2019, 7, 326 CrossRef PubMed.
- A. Zebibula, N. Alifu, L. Q. Xia, C. W. Sun, X. M. Yu, D. W. Xue, L. W. Liu, G. H. Li and J. Qian, Adv. Funct. Mater., 2018, 28, 1703451 CrossRef.
- S. S. Ling, X. H. Yang, C. Y. Li, Y. J. Zhang, H. C. Yang, G. C. Chen and Q. B. Wang, Angew. Chem., Int. Ed., 2020, 59, 7219–7223 CrossRef CAS PubMed.
- W. Lian, D. T. Tu, P. Hu, X. R. Song, Z. L. Gong, T. Chen, J. B. Song, Z. Chen and X. Y. Chen, Nano Today, 2020, 35, 100943 CrossRef CAS.
- H. M. Gil, T. W. Price, K. Chelani, J. S. G. Bouillard, S. D. J. Calaminus and G. J. Stasiuk, Iscience, 2021, 24, 102189 CrossRef CAS PubMed.
- J. J. Zhang, Y. Lin, H. Zhou, H. He, J. J. Ma, M. Y. Luo, Z. L. Zhang and D. W. Pang, Adv. Healthcare Mater., 2019, 8, 1900341 CrossRef PubMed.
- Y. Du, B. Xu, T. Fu, M. Cai, F. Li, Y. Zhang and Q. Wang, J. Am. Chem. Soc., 2010, 132, 1470–1471 CrossRef CAS PubMed.
- C. Li, W. Li, H. Liu, Y. Zhang, G. Chen, Z. Li and Q. Wang, Angew. Chem., Int. Ed., 2020, 59, 247–252 CrossRef CAS PubMed.
- O. T. Bruns, T. S. Bischof, D. K. Harris, D. Franke, Y. X. Shi, L. Riedemann, A. Bartelt, F. B. Jaworski, J. A. Carr, C. J. Rowlands, M. W. B. Wilson, O. Chen, H. Wei, G. W. Hwang, D. M. Montana, I. Coropceanu, O. B. Achorn, J. Kloepper, J. Heeren, P. T. C. So, D. Fukumura, K. F. Jensen, R. K. Jain and M. G. Bawendi, Nat. Biomed. Eng., 2017, 1, 0056 CrossRef CAS PubMed.
- Y. F. Tang, F. Pei, X. M. Lu, Q. L. Fan and W. Huang, Adv. Opt. Mater., 2019, 7, 1900917 CrossRef CAS.
- R. Tian, H. L. Ma, S. J. Zhu, J. Lau, R. Ma, Y. J. Liu, L. S. Lin, S. Chandra, S. Wang, X. F. Zhu, H. Z. Deng, G. Niu, M. X. Zhang, A. L. Antaris, K. S. Hettie, B. Yang, Y. Y. Liang and X. Y. Chen, Adv. Mater., 2020, 32, 1907365 CrossRef CAS PubMed.
- R. C. Jin, Nanoscale, 2015, 7, 1549–1565 RSC.
- R. C. Jin, C. J. Zeng, M. Zhou and Y. X. Chen, Chem. Rev., 2016, 116, 10346–10413 CrossRef CAS PubMed.
- Y. Z. Lu and W. Chen, Chem. Soc. Rev., 2012, 41, 3594–3623 RSC.
- I. Chakraborty and T. Pradeep, Chem. Rev., 2017, 117, 8208–8271 CrossRef CAS PubMed.
- L. B. Zhang and E. K. Wang, Nano Today, 2014, 9, 132–157 CrossRef CAS.
- E. Porret, X. Le Guevel and J. L. Coll, J. Mater. Chem. B, 2020, 8, 2216–2232 RSC.
- X. D. Zhang, D. Wu, X. Shen, P. X. Liu, F. Y. Fan and S. J. Fan, Biomaterials, 2012, 33, 4628–4638 CrossRef CAS PubMed.
- L. Dong, M. Li, S. Zhang, J. Li, G. Shen, Y. Tu, J. Zhu and J. Tao, Small, 2015, 11, 2571–2581 CrossRef CAS PubMed.
- Y. Pan, S. Neuss, A. Leifert, M. Fischler, F. Wen, U. Simon, G. Schmid, W. Brandau and W. Jahnen-Dechent, Small, 2007, 3, 1941–1949 CrossRef CAS PubMed.
- E. Porret, X. Le Guevel and J. L. Coll, J. Mater. Chem. B, 2020, 8, 2216–2232 RSC.
- J. J. Li, J. J. Zhu and K. Xu, TrAC, Trends Anal. Chem., 2014, 58, 90–98 CrossRef CAS.
- L. Shang, S. J. Dong and G. U. Nienhaus, Nano Today, 2011, 6, 401–418 CrossRef CAS.
- X. R. Song, N. Goswami, H. H. Yang and J. P. Xie, Analyst, 2016, 141, 3126–3140 RSC.
- Y. Tao, M. Q. Li, J. S. Ren and X. G. Qu, Chem. Soc. Rev., 2015, 44, 8636–8663 RSC.
- Y. Negishi, K. Nobusada and T. Tsukuda, J. Am. Chem. Soc., 2005, 127, 5261–5270 CrossRef CAS PubMed.
- Y. Shichibu, Y. Negishi, H. Tsunoyama, M. Kanehara, T. Teranishi and T. Tsukuda, Small, 2007, 3, 835–839 CrossRef CAS PubMed.
- H. Qian, Y. Zhu and R. Jin, ACS Nano, 2009, 3, 3795–3803 CrossRef CAS PubMed.
- H. Qian and R. Jin, Nano Lett., 2009, 9, 4083–4087 CrossRef CAS PubMed.
- H. Qian, M. Zhu, Z. Wu and R. Jin, Acc. Chem. Res., 2012, 45, 1470–1479 CrossRef CAS PubMed.
- Y. Yu, X. Chen, Q. F. Yao, Y. Yu, N. Yan and J. P. Xie, Chem. Mater., 2013, 25, 946–952 CrossRef CAS.
- C. Zhou, M. Long, Y. Qin, X. Sun and J. Zheng, Angew. Chem., Int. Ed., 2011, 50, 3168–3172 CrossRef CAS PubMed.
- Y. G. Srinivasulu, Q. F. Yao, N. Goswami and J. P. Xie, Mater. Horiz., 2020, 7, 2596–2618 RSC.
- X. Kang and M. Z. Zhu, Chem. Soc. Rev., 2019, 48, 2422–2457 RSC.
- S. Wang, X. Meng, A. Das, T. Li, Y. Song, T. Cao, X. Zhu, M. Zhu and R. Jin, Angew. Chem., Int. Ed., 2014, 53, 2376–2380 CrossRef CAS PubMed.
- K. Pyo, V. D. Thanthirige, K. Kwak, P. Pandurangan, G. Ramakrishna and D. Lee, J. Am. Chem. Soc., 2015, 137, 8244–8250 CrossRef CAS PubMed.
- Z. Luo, X. Yuan, Y. Yu, Q. Zhang, D. T. Leong, J. Y. Lee and J. Xie, J. Am. Chem. Soc., 2012, 134, 16662–16670 CrossRef CAS PubMed.
- Z. Wu, Q. Yao, O. J. H. Chai, N. Ding, W. Xu, S. Zang and J. Xie, Angew. Chem., Int. Ed., 2020, 59, 9934–9939 CrossRef CAS PubMed.
- A. Cantelli, G. Guidetti, J. Manzi, V. Caponetti and M. Montalti, Eur. J. Inorg. Chem., 2017, 5068–5084 CrossRef CAS.
- C. H. Yao, C. Q. Xu, I. H. Park, M. Zhao, Z. Y. Zhu, J. Li, X. Hai, H. Y. Fang, Y. Zhang, G. Macam, J. H. Teng, L. Li, Q. H. Xu, F. C. Chuang, J. P. Lu, C. L. Su, J. Li and J. Lu, Angew. Chem., Int. Ed., 2020, 59, 8270–8276 CrossRef CAS PubMed.
- Z. N. Wu, Y. H. Du, J. Liu, Q. F. Yao, T. K. Chen, Y. T. Cao, H. Zhang and J. P. Xie, Angew. Chem., Int. Ed., 2019, 58, 8139–8144 CrossRef CAS PubMed.
- A. Yahia-Ammar, D. Sierra, F. Merola, N. Hildebrandt and X. Le Guevel, ACS Nano, 2016, 10, 2591–2599 CrossRef CAS PubMed.
- J. Liu, M. Yu, X. Ning, C. Zhou, S. Yang and J. Zheng, Angew. Chem., Int. Ed., 2013, 52, 12572–12576 CrossRef CAS PubMed.
- X. Jiang, B. Du and J. Zheng, Nat. Nanotechnol., 2019, 14, 874–882 CrossRef CAS PubMed.
- J. Xu and L. Shang, Chin. Chem. Lett., 2018, 29, 1436–1444 CrossRef CAS.
- X. Q. Meng, I. Zare, X. Y. Yan and K. L. Fan, Wiley Interdiscip. Rev.: Nanomed. Nanobiotechnol., 2020, 12, e1602 Search PubMed.
- Z. J. Qiao, J. Zhang, X. Hai, Y. C. Yan, W. L. Song and S. Bi, Biosens. Bioelectron., 2021, 176, 112898 CrossRef CAS PubMed.
- J. Yang, T. Wang, L. N. Zhao, V. K. Rajasekhar, S. Joshi, C. Andreou, S. Pal, H. T. Hsu, H. W. Zhang, I. J. Cohen, R. M. Huang, R. C. Hendrickson, M. M. Miele, W. B. Pei, M. B. Brendel, J. H. Healey, G. Chiosis and M. F. Kircher, Nat. Biomed. Eng., 2020, 4, 686–703 CrossRef CAS PubMed.
- Y. Tan, K. He, B. Tang, H. Chen, Z. Zhao, C. Zhang, L. Lin and J. Liu, ACS Nano, 2020, 14, 13975–13985 CrossRef CAS PubMed.
- E. G. Ju, Z. Liu, Y. D. Du, Y. Tao, J. S. Ren and X. G. Qu, ACS Nano, 2014, 8, 6014–6023 CrossRef CAS PubMed.
- T. T. Chen, Y. H. Hu, Y. Cen, X. Chu and Y. Lu, J. Am. Chem. Soc., 2013, 135, 11595–11602 CrossRef CAS PubMed.
- H. F. Fang, H. Yu, Q. Lu, X. Fang, Q. L. Zhang, J. T. Zhang, L. L. Zhu and Q. B. Ma, Anal. Chem., 2020, 92, 12825–12832 CrossRef CAS PubMed.
- Y. Chen, L. B. Li, L. S. Gong, T. Y. Zhou and J. B. Liu, Adv. Funct. Mater., 2019, 29, 1806945 CrossRef.
- J. Q. Wang and G. Liu, Angew. Chem., Int. Ed., 2018, 57, 3008–3010 CrossRef CAS PubMed.
- J. Xu, M. X. Yu and J. Zheng, Angew. Chem., Int. Ed., 2017, 56, 13356–13360 CrossRef CAS PubMed.
- Y. Chen, D. M. Montana, H. Wei, J. M. Cordero, M. Schneider, X. Le Guevel, O. Chen, O. T. Bruns and M. G. Bawendi, Nano Lett., 2017, 17, 6330–6334 CrossRef CAS PubMed.
- Z. Yu, B. Musnier, K. D. Wegner, M. Henry, B. Chovelon, A. Desroches-Castan, A. Fertin, U. Resch-Genger, S. Bailly, J. L. Coll, Y. Usson, V. Josserand and X. Le Guevel, ACS Nano, 2020, 14, 4973–4981 CrossRef CAS PubMed.
- H. Liu, G. Hong, Z. Luo, J. Chen, J. Chang, M. Gong, H. He, J. Yang, X. Yuan, L. Li, X. Mu, J. Wang, W. Mi, J. Luo, J. Xie and X. D. Zhang, Adv. Mater., 2019, 31, e1901015 CrossRef PubMed.
- W. Wang, Y. Kong, J. Jiang, Q. Xie, Y. Huang, G. Li, D. Wu, H. Zheng, M. Gao, S. Xu, Y. Pan, W. Li, R. Ma, M. X. Wu, X. Li, H. Zuilhof, X. Cai and R. Li, Angew. Chem., Int. Ed., 2020, 59, 22431–22435 CrossRef CAS PubMed.
- Q. Li, C. J. t Zeman, Z. Ma, G. C. Schatz and X. W. Gu, Small, 2021, 17, e2007992 CrossRef PubMed.
- Y. Ishida, R. D. Corpuz and T. Yonezawa, Acc. Chem. Res., 2017, 50, 2986–2995 CrossRef CAS PubMed.
- F. Wang and X. G. Liu, Acc. Chem. Res., 2014, 47, 1378–1385 CrossRef CAS PubMed.
- G. Y. Chen, C. H. Yang and P. N. Prasad, Acc. Chem. Res., 2013, 46, 1474–1486 CrossRef CAS PubMed.
- Y. J. Yang, D. T. Tu, Y. Q. Zhang, P. Zhang and X. Y. Chen, Iscience, 2021, 24, 102062 CrossRef CAS PubMed.
- H. Li, X. Wang, T. Y. Ohulchanskyy and G. Y. Chen, Adv. Mater., 2021, 33, 2000678 CrossRef CAS PubMed.
- J. P. Leonard, C. B. Nolan, F. Stomeo and T. Gunnlaugsson, Top. Curr. Chem., 2007, 281, 1–43 CrossRef CAS.
- G. F. de Sa, O. L. Malta, C. D. Donega, A. M. Simas, R. L. Longo, P. A. Santa-Cruz and E. F. da Silva, Coordin. Chem. Rev., 2000, 196, 165–195 CrossRef CAS.
- J. C. G. Bunzli, Coordin. Chem. Rev., 2015, 293, 19–47 CrossRef.
- S. V. Eliseeva and J. C. Bunzli, Chem. Soc. Rev., 2010, 39, 189–227 RSC.
- H. Dong, S. R. Du, X. Y. Zheng, G. M. Lyu, L. D. Sun, L. D. Li, P. Z. Zhang, C. Zhang and C. H. Yan, Chem. Rev., 2015, 115, 10725–10815 CrossRef CAS PubMed.
- X. R. Song, S. H. Li, H. H. Guo, W. W. You, X. Y. Shang, R. F. Li, D. T. Tu, W. Zheng, Z. Chen, H. H. Yang and X. Y. Chen, Angew. Chem., Int. Ed., 2019, 58, 18981–18986 CrossRef CAS PubMed.
- Q. Q. Ma, J. Wang, Z. H. Li, X. B. Lv, L. Liang and Q. Yuan, Small, 2019, 15, 1804969 CrossRef PubMed.
- H. H. Guo, X. R. Song, W. Lei, C. He, W. W. You, Q. Z. Lin, S. Y. Zhou, X. Y. Chen and Z. Chen, Angew. Chem., Int. Ed., 2019, 58, 12195–12199 CrossRef CAS PubMed.
- S. Heer, K. Kompe, H. U. Gudel and M. Haase, Adv. Mater., 2004, 16, 2102–2105 CrossRef CAS.
- Y. Fan, L. Liu and F. Zhang, Nano Today, 2019, 25, 68–84 CrossRef CAS.
- H. Dong, L. D. Sun, L. D. Li, R. Si, R. Liu and C. H. Yan, J. Am. Chem. Soc., 2017, 139, 18492–18495 CrossRef CAS PubMed.
- F. Wang, R. R. Deng, J. Wang, Q. X. Wang, Y. Han, H. M. Zhu, X. Y. Chen and X. G. Liu, Nat. Mater., 2011, 10, 968–973 CrossRef CAS PubMed.
- K. A. Abel, J. C. Boyer and F. C. van Veggel, J. Am. Chem. Soc., 2009, 131, 14644–14645 CrossRef CAS PubMed.
- S. H. Yu, D. T. Tu, W. Lian, J. Xu and X. Y. Chen, Sci. China: Mater., 2019, 62, 1071–1086 CAS.
- S. W. Ding, L. F. Lu, Y. Fan and F. Zhang, J. Rare Earth., 2020, 38, 451–463 CrossRef CAS.
- L. Xiong, T. Yang, Y. Yang, C. Xu and F. Li, Biomaterials, 2010, 31, 7078–7085 CrossRef CAS PubMed.
- Y. T. Zhong, Z. R. Ma, F. F. Wang, X. Wang, Y. J. Yang, Y. L. Liu, X. Zhao, J. C. Li, H. T. Du, M. X. Zhang, Q. H. Cui, S. J. Zhu, Q. C. Sun, H. Wan, Y. Tian, Q. Liu, W. Z. Wang, K. C. Garcia and H. J. Dai, Nat. Biotechnol., 2019, 37, 1322–1331 CrossRef CAS PubMed.
- Y. S. Liu, D. T. Tu, H. M. Zhu and X. Y. Chen, Chem. Soc. Rev., 2013, 42, 6924–6958 RSC.
- G. F. Wang, Q. Peng and Y. D. Li, Acc. Chem. Res., 2011, 44, 322–332 CrossRef CAS PubMed.
- B. Liu, C. X. Li, P. P. Yang, Z. Y. Hou and J. Lin, Adv. Mater., 2017, 29, 1605434 CrossRef PubMed.
- A. Sedlmeier and H. H. Gorris, Chem. Soc. Rev., 2015, 44, 1526–1560 RSC.
- L. N. Sun, R. Y. Wei, J. Feng and H. J. Zhang, Coord. Chem. Rev., 2018, 364, 10–32 CrossRef CAS.
- G. Chen, T. Y. Ohulchanskyy, R. Kumar, H. Agren and P. N. Prasad, ACS Nano, 2010, 4, 3163–3168 CrossRef CAS PubMed.
- S. Y. Han, R. R. Deng, X. J. Xie and X. G. Liu, Angew. Chem., Int. Ed., 2014, 53, 11702–11715 CrossRef CAS PubMed.
- X. D. Wang, R. R. Valiev, T. Y. Ohulchanskyy, H. Agren, C. H. Yang and G. Y. Chen, Chem. Soc. Rev., 2017, 46, 4150–4167 RSC.
- C. Li, L. Xu, Z. Liu, Z. Li, Z. Quan, A. A. Al Kheraif and J. Lin, Dalton Trans., 2018, 47, 8538–8556 RSC.
- D. J. Gargas, E. M. Chan, A. D. Ostrowski, S. Aloni, M. V. P. Altoe, E. S. Barnard, B. Sanii, J. J. Urban, D. J. Milliron, B. E. Cohen and P. J. Schuck, Nat. Nanotechnol., 2014, 9, 300–305 CrossRef CAS PubMed.
- M. Quintanilla, F. Q. Ren, D. L. Ma and F. Vetrone, ACS Photonics, 2014, 1, 662–669 CrossRef CAS.
- Y. Zhang, Z. Z. Yu, J. Q. Li, Y. X. Ao, J. W. Xue, Z. P. Zeng, X. L. Yang and T. T. Y. Tan, ACS Nano, 2017, 11, 2846–2857 CrossRef CAS PubMed.
- H. Xu, S. Y. Han, R. R. Deng, Q. Q. Su, Y. Wei, Y. A. Tang, X. Qin and X. G. Liu, Nat. Photonics, 2021, 15, 732–737 CrossRef CAS.
- T. Joshi, C. Mamat and H. Stephan, ChemistryOpen, 2020, 9, 703–712 CrossRef CAS PubMed.
- Y. Sun, W. Feng, P. Yang, C. Huang and F. Li, Chem. Soc. Rev., 2015, 44, 1509–1525 RSC.
- A. Gnach, T. Lipinski, A. Bednarkiewicz, J. Rybka and J. A. Capobianco, Chem. Soc. Rev., 2015, 44, 1561–1584 RSC.
- J. Tian, X. Zeng, X. Xie, S. Han, O. W. Liew, Y. T. Chen, L. Wang and X. Liu, J. Am. Chem. Soc., 2015, 137, 6550–6558 CrossRef CAS PubMed.
- S. Z. Chen, C. M. Zhang, G. Jia, J. L. Duan, S. X. Wang and J. C. Zhang, Mater. Sci. Eng., C, 2014, 43, 330–342 CrossRef CAS PubMed.
- Q. Zhang, S. O'Brien and J. Grimm, Nanotheranostics, 2022, 6, 184–194 CrossRef PubMed.
- A. A. A. Ansari, A. K. K. Parchur and G. Y. Chen, Coordin. Chem. Rev., 2022, 457, 214423 CrossRef CAS.
- A. Gnach, T. Lipinski, A. Bednarkiewicz, J. Rybka and J. A. Capobianco, Chem. Soc. Rev., 2015, 44, 1561–1584 RSC.
- R. Abdul Jalil and Y. Zhang, Biomaterials, 2008, 29, 4122–4128 CrossRef CAS PubMed.
- L. Cheng, K. Yang, M. Shao, X. Lu and Z. Liu, Nanomedicine, 2011, 6, 1327–1340 CrossRef CAS PubMed.
- Y. Sun, Q. Liu, J. Peng, W. Feng, Y. Zhang, P. Yang and F. Li, Biomaterials, 2013, 34, 2289–2295 CrossRef CAS PubMed.
- H. Li, X. Wang, X. L. Li, S. J. Zeng and G. Y. Chen, Chem. Mater., 2020, 32, 3365–3375 CrossRef CAS.
- J. A. Damasco, T. Y. Ohulchanskyy, S. Mahajan, G. Chen, A. Singh, H. L. Kutscher, H. Huang, S. G. Turowski, J. A. Spernyak, A. K. Singh, J. F. Lovell, M. Seshadri and P. N. Prasad, Cancer Nanotechnol., 2021, 12, 4 CrossRef CAS PubMed.
- L. D. Sun, Y. F. Wang and C. H. Yan, Acc. Chem. Res., 2014, 47, 1001–1009 CrossRef CAS PubMed.
- Q. Liu, Y. Sun, T. Yang, W. Feng, C. Li and F. Li, J. Am. Chem. Soc., 2011, 133, 17122–17125 CrossRef CAS PubMed.
- D. Yang, Y. Dai, J. Liu, Y. Zhou, Y. Chen, C. Li, P. Ma and J. Lin, Biomaterials, 2014, 35, 2011–2023 CrossRef CAS PubMed.
- F. Ren, L. Ding, H. Liu, Q. Huang, H. Zhang, L. Zhang, J. Zeng, Q. Sun, Z. Li and M. Gao, Biomaterials, 2018, 175, 30–43 CrossRef CAS PubMed.
- X. Ou, X. Qin, B. Huang, J. Zan, Q. Wu, Z. Hong, L. Xie, H. Bian, Z. Yi, X. Chen, Y. Wu, X. Song, J. Li, Q. Chen, H. Yang and X. Liu, Nature, 2021, 590, 410–415 CrossRef CAS PubMed.
- H. Y. Liang, Z. Z. Hong, S. H. Li, X. R. Song, D. Zhang, Q. S. Chen, J. Li and H. H. Yang, Adv. Funct. Mater., 2021, 31, 2006353 CrossRef CAS.
- C. Sun, G. Pratx, C. M. Carpenter, H. Liu, Z. Cheng, S. S. Gambhir and L. Xing, Adv. Mater., 2011, 23, H195–H199 CrossRef CAS PubMed.
- D. J. Naczynski, C. Sun, S. Turkcan, C. Jenkins, A. L. Koh, D. Ikeda, G. Pratx and L. Xing, Nano Lett., 2015, 15, 96–102 CrossRef CAS PubMed.
- P. Pei, Y. Chen, C. Sun, Y. Fan, Y. Yang, X. Liu, L. Lu, M. Zhao, H. Zhang, D. Zhao, X. Liu and F. Zhang, Nat. Nanotechnol., 2021, 16, 1011–1018 CrossRef CAS PubMed.
- Z. Xue, X. Li, Y. Li, M. Jiang, H. Liu, S. Zeng and J. Hao, ACS Appl. Mater. Interfaces, 2017, 9, 22132–22142 CrossRef CAS PubMed.
- Z. Hu, Y. Qu, K. Wang, X. Zhang, J. Zha, T. Song, C. Bao, H. Liu, Z. Wang, J. Wang, Z. Liu, H. Liu and J. Tian, Nat. Commun., 2015, 6, 7560 CrossRef PubMed.
- S. Goel, F. Chen, E. B. Ehlerding and W. Cai, Small, 2014, 10, 3825–3830 CrossRef CAS PubMed.
- F. Peng, Y. Su, Y. Zhong, C. Fan, S. T. Lee and Y. He, Acc. Chem. Res., 2014, 47, 612–623 CrossRef CAS PubMed.
- Y. He, C. H. Fan and S. T. Lee, Nano Today, 2010, 5, 282–295 CrossRef CAS.
- J. H. Liang, C. B. Huang and X. Gong, ACS Sustainable Chem. Eng., 2019, 7, 18213–18227 CrossRef CAS.
- D. S. Karaman, M. P. Sarparanta, J. M. Rosenholm and A. J. Airaksinen, Adv. Mater., 2018, 30, e1703651 CrossRef PubMed.
- S. Morozova, M. Alikina, A. Vinogradov and M. Pagliaro, Front. Chem., 2020, 8, 191 CrossRef CAS PubMed.
- S. Chinnathambi, S. Chen, S. Ganesan and N. Hanagata, Adv. Healthcare Mater., 2014, 3, 10–29 CrossRef CAS PubMed.
- M. Qiu, A. Singh, D. Wang, J. L. Qu, M. Swihart, H. Zhang and P. N. Prasad, Nano Today, 2019, 25, 135–155 CrossRef CAS.
- B. F. McVey and R. D. Tilley, Acc. Chem. Res., 2014, 47, 3045–3051 CrossRef CAS PubMed.
- X. Y. Cheng, S. B. Lowe, P. J. Reece and J. J. Gooding, Chem. Soc. Rev., 2014, 43, 2680–2700 RSC.
- B. Song and Y. He, Nano Today, 2019, 26, 149–163 CrossRef CAS.
- X. Cheng, E. Hinde, D. M. Owen, S. B. Lowe, P. J. Reece, K. Gaus and J. J. Gooding, Adv. Mater., 2015, 27, 6144–6150 CrossRef CAS PubMed.
- X. Ji, F. Peng, Y. Zhong, Y. Su, X. Jiang, C. Song, L. Yang, B. Chu, S. T. Lee and Y. He, Adv. Mater., 2015, 27, 1029–1034 CrossRef CAS PubMed.
- A. S. Heintz, M. J. Fink and B. S. Mitchell, Adv. Mater., 2007, 19, 3984–3988 CrossRef CAS.
- Z. Yuan, T. Nakamura, S. Adachi and K. Matsuishi, J. Phys. Chem. C, 2017, 121, 8623–8629 CrossRef CAS.
- Y. Han, Y. Chen, J. Feng, J. Liu, S. Ma and X. Chen, Anal. Chem., 2017, 89, 3001–3008 CrossRef CAS PubMed.
- H. L. Ye, S. J. Cai, S. Li, X. W. He, W. Y. Li, Y. H. Li and Y. K. Zhang, Anal. Chem., 2016, 88, 11631–11638 CrossRef CAS PubMed.
- S. Guruvenket, J. M. Hoey, K. J. Anderson, M. T. Frohlich, R. Krishnan, J. Sivaguru, M. P. Sibi and P. Boudjouk, J. Mater. Chem. C, 2016, 4, 8206–8213 RSC.
- A. Gupta, M. T. Swihart and H. Wiggers, Adv. Funct. Mater., 2009, 19, 696–703 CrossRef CAS.
- C. M. Hessel, E. J. Henderson and J. G. C. Veinot, Chem. Mater., 2006, 18, 6139–6146 CrossRef CAS.
- X. Li, Y. He and M. T. Swihart, Langmuir, 2004, 20, 4720–4727 CrossRef CAS PubMed.
- K. Sato, N. Fukata, K. Hirakuri, M. Murakami, T. Shimizu and Y. Yamauchi, Chem. – Asian J., 2010, 5, 50–55 CrossRef CAS PubMed.
- S. Chinnathambi, S. Chen, S. Ganesan and N. Hanagata, Adv. Healthcare
Mater., 2014, 3, 10–29 CrossRef CAS PubMed.
- M. Montalti, A. Cantelli and G. Battistelli, Chem. Soc. Rev., 2015, 44, 4853–4921 RSC.
- J. Liu, F. Erogbogbo, K. T. Yong, L. Ye, J. Liu, R. Hu, H. Chen, Y. Hu, Y. Yang, J. Yang, I. Roy, N. A. Karker, M. T. Swihart and P. N. Prasad, ACS Nano, 2013, 7, 7303–7310 CrossRef CAS PubMed.
- J. H. Park, L. Gu, G. von Maltzahn, E. Ruoslahti, S. N. Bhatia and M. J. Sailor, Nat. Mater., 2009, 8, 331–336 CrossRef CAS PubMed.
- L. Gu, D. J. Hall, Z. Qin, E. Anglin, J. Joo, D. J. Mooney, S. B. Howell and M. J. Sailor, Nat. Commun., 2013, 4, 2326 CrossRef PubMed.
- H. Wang and Y. He, Sensors, 2017, 17, 268 CrossRef PubMed.
- C. Tu, X. Ma, P. Pantazis, S. M. Kauzlarich and A. Y. Louie, J. Am. Chem. Soc., 2010, 132, 2016–2023 CrossRef CAS PubMed.
- C. Tu, X. Ma, A. House, S. M. Kauzlarich and A. Y. Louie, ACS Med. Chem. Lett., 2011, 2, 285–288 CrossRef CAS PubMed.
- F. Erogbogbo, K. T. Yong, I. Roy, R. Hu, W. C. Law, W. Zhao, H. Ding, F. Wu, R. Kumar, M. T. Swihart and P. N. Prasad, ACS Nano, 2011, 5, 413–423 CrossRef CAS PubMed.
- J. G. Croissant, K. S. Butler, J. I. Zink and C. J. Brinker, Nat. Rev. Mater., 2020, 5, 886–909 CrossRef CAS.
- T. I. Janjua, Y. X. Cao, C. Z. Yu and A. Popat, Nat. Rev. Mater., 2021, 6, 1072–1074 CrossRef CAS PubMed.
- S. Sato and M. T. Swihart, Chem. Mater., 2006, 18, 4083–4088 CrossRef CAS.
- Y. Y. Su, X. Y. Ji and Y. He, Adv. Mater., 2016, 28, 10567–10574 CrossRef CAS PubMed.
- F. Erogbogbo, K. T. Yong, I. Roy, G. X. Xu, P. N. Prasad and M. T. Swihart, ACS Nano, 2008, 2, 873–878 CrossRef CAS PubMed.
- F. Erogbogbo, K. T. Yong, R. Hu, W. C. Law, H. Ding, C. W. Chang, P. N. Prasad and M. T. Swihart, ACS Nano, 2010, 4, 5131–5138 CrossRef CAS PubMed.
- C. H. Lai, J. Hutter, C. W. Hsu, H. Tanaka, S. Varela-Aramburu, L. De Cola, B. Lepenies and P. H. Seeberger, Nano Lett., 2016, 16, 807–811 CrossRef CAS PubMed.
- C. Song, Y. Zhong, X. Jiang, F. Peng, Y. Lu, X. Ji, Y. Su and Y. He, Anal. Chem., 2015, 87, 6718–6723 CrossRef CAS PubMed.
- Y. F. Zhou, Y. Zhang, Y. L. Zhong, R. Fu, S. C. Wu, Q. Wang, H. Y. Wang, Y. Y. Su, H. M. Zhang and Y. He, Nano Res., 2018, 11, 2336–2346 CrossRef CAS.
- Z. F. Li and E. Ruckenstein, Nano Lett., 2004, 4, 1463–1467 CrossRef CAS.
- J. H. Warner, A. Hoshino, K. Yamamoto and R. D. Tilley, Angew. Chem., Int. Ed., 2005, 44, 4550–4554 CrossRef CAS PubMed.
- S. Chinnathambi, S. Chen, S. Ganesan and N. Hanagata, Adv. Healthcare Mater., 2014, 3, 10–29 CrossRef CAS PubMed.
- X. Zhai, B. Song, B. B. Chu, Y. Y. Su, H. Y. Wang and Y. He, Nano Res., 2018, 11, 6417–6427 CrossRef CAS.
- L. Zhang, X. Y. Ji, Y. Y. Su, X. Zhai, H. Xu, B. Song, A. R. Jiang, D. X. Guo and Y. He, Nano Res., 2021, 14, 52–58 CrossRef.
- J. Han, L. Zhang, M. Cui, Y. Su and Y. He, Anal. Chem., 2021, 93, 10122–10131 CrossRef CAS PubMed.
- S. Takeoka, M. Fujii and S. Hayashi, Phys. Rev. B: Condens. Matter Mater. Phys., 2000, 62, 16820–16825 CrossRef CAS.
- M. Sakiyama, H. Sugimoto and M. Fujii, Nanoscale, 2018, 10, 13902–13907 RSC.
- H. Sugimoto, M. Fujii, K. Imakita, S. Hayashi and K. Akamatsu, J. Phys. Chem. C, 2013, 117, 11850–11857 CrossRef CAS.
- Y. Xu, P. Li, D. Cheng, C. Wu, Q. Lu, W. Yang, X. Zhu, P. Yin, M. Liu, H. Li and Y. Zhang, J. Mater. Chem. B, 2020, 8, 10290–10308 RSC.
- K. Ma, H. Sai and U. Wiesner, J. Am. Chem. Soc., 2012, 134, 13180–13183 CrossRef CAS PubMed.
- R. Narayan, U. Y. Nayak, A. M. Raichur and S. Garg, Pharmaceutics, 2018, 10, 118 CrossRef CAS PubMed.
- F. Chen, B. Madajewski, K. Ma, D. K. Zanoni, H. Stambuk, M. Z. Turker, S. Monette, L. Zhang, B. Yoo, P. M. Chen, R. J. C. Meester, S. de Jonge, P. Montero, E. Phillips, T. P. Quinn, M. Gonen, S. Sequeira, E. de Stanchina, P. Zanzonico, U. Wiesner, S. G. Patel and M. S. Bradbury, Sci. Adv., 2019, 5, eaax5208 CrossRef CAS PubMed.
- H. Ma, B. Song, Y. Wang, D. Cong, Y. Jiang and J. Yuan, Chem. Sci., 2017, 8, 150–159 RSC.
- P. Kalluru, R. Vankayala, C. S. Chiang and K. C. Hwang, Adv. Funct. Mater., 2016, 26, 7908–7920 CrossRef CAS.
- D. L. J. Thorek, A. Ogirala, B. J. Beattie and J. Grimm, Nat. Med., 2013, 19, 1345–1350 CrossRef CAS PubMed.
- F. Lux, V. L. Tran, E. Thomas, S. Dufort, F. Rossetti, M. Martini, C. Truillet, T. Doussineau, G. Bort, F. Denat, F. Boschetti, G. Angelovski, A. Detappe, Y. Cremillieux, N. Mignet, B. T. Doan, B. Larrat, S. Meriaux, E. Barbier, S. Roux, P. Fries, A. Muller, M. C. Abadjian, C. Anderson, E. Canet-Soulas, P. Bouziotis, M. Barberi-Heyob, C. Frochot, C. Verry, J. Balosso, M. Evans, J. Sidi-Boumedine, M. Janier, K. Butterworth, S. McMahon, K. Prise, M. T. Aloy, D. Ardail, C. Rodriguez-Lafrasse, E. Porcel, S. Lacombe, R. Berbeco, A. Allouch, J. L. Perfettini, C. Chargari, E. Deutsch, G. Le Duc and O. Tillement, Br. J. Radiol., 2019, 92, 20180365 Search PubMed.
- D. Yan, T. Li, Y. Yang, N. Niu, D. Wang, J. Ge, L. Wang, R. Zhang, D. Wang and B. Z. Tang, Adv. Mater., 2022, 34, e2206643 CrossRef PubMed.
- J. Xu, T. Han, Y. Wang, F. Zhang, M. Li, L. Bai, X. Wang, B. Sun, X. Wang, J. Du, K. Liu, J. Zhang and S. Zhu, Nano Lett., 2022, 22, 7965–7975 CrossRef CAS PubMed.
- L. Cheng, D. Jiang, A. Kamkaew, H. F. Valdovinos, H. J. Im, L. Feng, C. G. England, S. Goel, T. E. Barnhart, Z. Liu and W. Cai, Adv. Funct. Mater., 2017, 27, 2100549 Search PubMed.
- B. S. Tian, S. K. Liu, L. L. Feng, S. H. Liu, S. L. Gai, Y. L. Dai, L. S. Xie, B. Liu, P. P. Yang and Y. L. Zhao, Adv. Funct. Mater., 2021, 31, 2100549 CrossRef CAS.
- H. X. Liu, J. Y. Wang, Y. Q. Jing, J. Yang, X. T. Bai, X. Y. Mu, F. J. Xu, X. H. Xue, L. F. Liu, Y. M. Sun, Q. Liu, H. T. Dai, C. L. Liu and X. D. Zhang, Part. Part. Syst. Char., 2017, 34, 1700035 CrossRef.
- S. H. Zheng, M. Zhang, H. Y. Bai, M. J. He, L. N. Dong, L. L. Cai, M. M. Zhao, Q. Wang, K. Xu and J. J. Li, Int. J. Nanomed., 2019, 14, 9513–9524 CrossRef CAS PubMed.
- X. Men, X. R. Geng, Z. Zhang, H. B. Chen, M. Du, Z. Y. Chen, G. Liu, C. F. Wu and Z. Yuan, Mater. Today Bio., 2022, 16, 100383 CrossRef CAS PubMed.
- J. Wang, Q. Z. Li, H. Zhao, W. T. Yue, K. W. Zhang, X. Y. Jiang and K. Li, ACS Nano, 2022, 16, 462–472 CrossRef CAS PubMed.
- J. Liu, T. Lecuyer, J. Seguin, N. Mignet, D. Scherman, B. Viana and C. Richard, Adv. Drug Delivery Rev., 2019, 138, 193–210 CrossRef CAS PubMed.
- A. C. Anselmo and S. Mitragotri, Bioeng. Transl. Med., 2019, 4, e10143 Search PubMed.
- Imaging nanoparticle-all studies, https://clinicaltrials.gov/ct2/results?recrs=&cond=&term=%22imaging%22+AND+%22nanoparticle%22&cntry=&state=&city=&dist=, (accessed July 27, 2022).
- S. M. Park, A. Aalipour, O. Vermesh, J. H. Yu and S. S. Gambhir, Nat. Rev. Mater., 2017, 2, 1–20 Search PubMed.
- C. Wang, W. Fan, Z. Zhang, Y. Wen, L. Xiong and X. Chen, Adv. Mater., 2019, 31, e1904329 CrossRef PubMed.
- M. T. Olson, Q. P. Ly and A. M. Mohs, Mol. Imaging Biol., 2019, 21, 200–218 CrossRef PubMed.
- L. J. Lauwerends, P. van Driel, R. J. Baatenburg de Jong, J. A. U. Hardillo, S. Koljenovic, G. Puppels, L. Mezzanotte, C. Lowik, E. L. Rosenthal, A. L. Vahrmeijer and S. Keereweer, Lancet Oncol., 2021, 22, e186–e195 CrossRef CAS PubMed.
|
This journal is © The Royal Society of Chemistry 2023 |
Click here to see how this site uses Cookies. View our privacy policy here.