Field based studies on aging characteristics of pristine and aged plastic debris in a coastal environment, Bohai Bay, China†
Received
25th October 2022
, Accepted 7th December 2022
First published on 8th December 2022
Abstract
The coastal environment has become a sink of plastic due to the strong impact of plastic waste input from land. Plastics entering a coastal environment usually experience aging on land. However, few previous studies used aged plastics to study plastic aging in seawater, and the aging characteristics of aged plastics in a coastal environment are unclear. In our study, a ten-week investigation of the aging characteristics of pristine and pre-aged polypropylene plastic debris was conducted in Bohai Bay, China. During ten-week field exposure, more biofilms formed on the surfaces of pre-aged plastic debris than pristine plastic debris. However, no significant differences were found in the physicochemical properties (surface chemistry, hydrophobicity, and crystallinity) between pristine and pre-aged plastic debris. In addition, the results of redundancy analysis (RDA) illustrated that temperature was a key factor influencing the aging characteristics of plastic debris. Our research suggests that the aging history can affect the density of plastic debris by affecting the adhesion of the biofilm, which may influence the fate of plastic debris. In a coastal environment, plastic debris at different aging stages with the same initial chemical composition had basically similar changes in physicochemical properties in the short term.
Environmental significance
A comprehensive exploration of the aging characteristics of plastics is critical for understanding the fate of plastics in a coastal environment. Most plastics have undergone extensive aging on land before entering the coastal environment. The aging behaviors of plastics with a history of terrestrial aging in a coastal environment are unclear. In this work, the aging characteristics of pristine and aged plastics in a coastal environment were investigated. The results of this work indicated that the aging history of plastics was more directly affecting the biofouling of plastic debris and plastic surface morphology in a coastal environment, thus affecting the associated aging process. The differences in physicochemical properties between plastic debris were eliminated during field exposure. Plastics can exist in debris form for a long time.
|
1 Introduction
Plastics are widely used in daily life due to their durability and low cost. Since the large-scale production and use of plastics in the 1950s, plastic production has increased year by year, and reached 367 million tons in 2020.1,2 Due to its slow degradation, plastic waste can easily accumulate in the environment as a persistent pollutant. In a natural environment, the complete decomposition of plastics usually takes hundreds of years.3 The degradation period of plastics typically follows this order: polyethylene (PE, about 1000 years) > polystyrene (PS, about 500 years) > polyethylene terephthalate (PET, about 450 years) > polyvinyl chloride (PVC, about 100 years) > polypropylene (PP, 20–30 years).4 Therefore, under natural aging conditions, most plastics do not completely “disappear” in the environment but are broken down into smaller pieces by physical abrasion, ultraviolet (UV) radiation, chemical oxidation, and biodegradation. These processes can lead to the loss of physical integrity and degradation of polymers, which is known as plastic aging.5 Studies have shown that the aging process can significantly alter the physical and chemical properties of plastics, including surface chemistry,6 tensile strength,7 crystallinity,8 molecular weight,9 mass loss,10etc. As the coastal environment is an important sink of plastic debris, there has been a growing concern about the fate of plastics in seawater.
As early as the 1980s, the exposure of several types of plastic (including PE, PP and PS) to seawater was studied and a reduction in the tensile properties of plastics was found after one year of exposure.7,11 The contents of oxygen functional groups of aged PE, low-density polyethylene (LDPE) and high-density polyethylene (HDPE) were increased after 3 years of seawater/sun exposure, respectively.12 Besides, the aging behavior of some representative flexible plastic packaging films in a marine environment was investigated.13 Traditional plastics (including PE and PET) did not degrade significantly in seawater for 12 months, indicating that plastics had strong persistence in the marine environment. In aquatic environments, microbial colonization on plastic waste is a rapid process.14 Biofouling can easily affect the hydrophobicity and density of plastics. Marine phytoplankton aggregates could affect vertical migration of microplastics in the water column.15 A study of PE aging indicated that biofilms and the dominant functional microbes attached to the microplastic surface will affect the migration, sinking, aging, and degradation of microplastics in the environment.16 Zettler et al. observed that the pits on the polymer surface were conformed to the shape of colonized bacteria, indicating polymer degradation.17 However, the materials commonly used in these studies on plastic aging were pristine plastics. In the real environment, most of the plastics entering the seawater have undergone an extensive aging process on land, and the aging characteristics of the aged plastics under coastal exposure conditions are unclear. Therefore, it is necessary to use aged plastics and compare them with pristine plastics to test whether there are differences in their aging characteristics in a coastal environment.
The Bohai Sea is a semi-enclosed sea area in northern China, with an area of 1.59 × 104 km2. The Haihe River is one of the main rivers flowing into the Bohai Sea. Due to the large number of surrounding industrial cities and poor self-purification ability, plastic pollution in the Bohai Sea is serious. According to previous studies, the average concentration of microplastics floating in the Bohai Sea, China was 0.33 ± 0.34 particles per m3, of which PP accounted for 29% of the total microplastics.18
In this study, commercial PP plastic was selected as the research material, which was the most abundant type of plastic found in Bohai Bay, China.19 We conducted a ten-week field exposure study with both pre-aged plastic and pristine plastic in situ, and explored the aging process of these plastics in a coastal environment. This study concerned the persistence of plastic and might provide a reference for the management of plastic waste in a coastal environment.
2 Materials and methods
2.1 Laboratory pre-aging treatment
The plastic used in the study was transparent commercial PP plastic purchased from Dongguan Jinqiao Plastic Material Co., Ltd, with a density of 0.92–0.95 kg dm−3 and strong acid and alkali resistance. It is used in product packaging, stationery, and electronics. The PP plastic was cut into 1 cm × 1 cm × 0.3 mm debris. Half of the plastic debris was pre-aged for 600 h using two 40 W UV-lamps (UV-B, with a central wavelength of 313 nm) in the laboratory. The spectrogram of the UV lamp is shown in Fig. S1.† These plastic debris were placed 20 cm away from the lamps for pre-aging treatment. In addition, the intensity of UV-light was 1.4 W m−2 measured by using a UV intensity meter. The energy of the UV-B lamp during the day was about four times that of the natural UV-B. The pre-aged plastic debris together with the pristine plastic debris were applied in the subsequent experiments.
2.2 Field exposure experiment
The field exposure site was selected in Bohai Bay, China, where the plastic debris were deployed (Fig. 1). The pristine and pre-aged plastic debris were placed into a 8-mesh nylon bag (15 cm × 20 cm). The material of nylon bags was translucent, most of water, sunlight and microorganisms could pass through the mesh. To prevent the aggregation of plastic debris, the nylon bags were divided into small spaces with nylon rope, so that the plastic debris could evenly come into contact with the environment (Fig. S2†). A total of 30 pieces of plastic were placed in each nylon bag. Then, the nylon bags were immersed in water by using a rope and a 2 kg plumb bob. Plastic debris were deployed about 30 cm underwater at low tide. A ten-week field exposure experiment was conducted from May 18, 2021 to July 27, 2021. Both the pristine and pre-aged plastic debris were divided into five groups respectively, and one group of each type was harvested at low tide every two weeks.
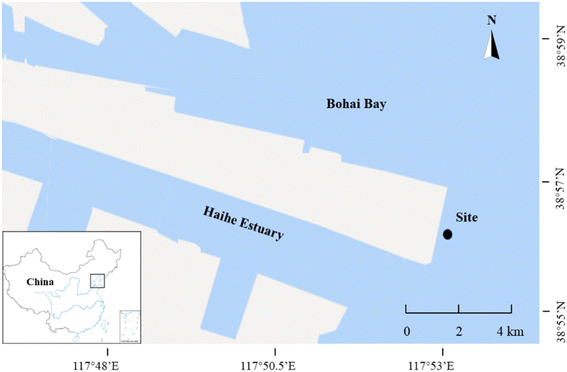 |
| Fig. 1 The selected site in Bohai Bay, China. | |
2.3 Determination of environmental parameters
When deploying and harvesting plastic debris each time, a 2 L seawater sample was collected at about 30 cm underwater using a water collector.20 The environmental parameters, including temperature, salinity, dissolved oxygen (DO), pH and total organic carbon (TOC) were measured at each sampling. Temperature, pH, and DO were measured in situ. A pH meter was used to determine the seawater temperature and pH, and a portable dissolved oxygen analyzer was used to determine the dissolved oxygen content. Salinity was measured in the laboratory using a salinometer. After the collected seawater sample was transported to the laboratory, it was first filtered through fast quantitative filter paper (80–120 μm) to remove impurities, and then passed through a 0.22 μm polycarbonate filter (Merck Millipore, Billerica, MA). A TOC analyzer was used to measure the total organic carbon of the filtered seawater sample. Three parallel seawater samples were used for the determination of environmental parameters, and the average value was taken. The latitude and longitude as well as the environmental parameters of the sampling site are summarized in Table S1.†
2.4 Surface topography
The biofilm morphology and plastic surface morphology of plastic debris were observed by using a scanning electron microscope (SEM) (Zeiss Sigma, Germany). For the SEM observation, plastic debris with biofilms were fixed in 3% (v/v) glutaraldehyde solution at 4 °C overnight, and then dehydrated with 25%, 50%, 75%, and 100% ethanol for 10 minutes successively.21 For the observation of the surface morphology of plastic debris, it was necessary to remove the biofilm on plastic debris using 2% (v/v) sodium dodecyl sulfate (SDS). All the plastic debris were platinum-plated and then observed under a SEM.21
2.5 Biomass measurement
The biofilm content of plastic debris was evaluated by the method of crystal violet staining. The principle of biofilm content determination is that the optical density is proportional to the biomass of biofilms.22,23 According to a previous study,23 plastic debris were rinsed 3 times with sterile water and then placed in sterile Petri dishes with three replicates. Next, plastic debris were air-dried at room temperature for at least 45 minutes and stained with crystal violet dye (1% w/v) for 45 minutes. The plastic debris were further rinsed 3 times with sterile water and air-dried at room temperature for another 45 minutes. Five pieces of plastic debris were then placed in a 50 mL sterilized centrifuge tube and 10 mL of ethanol (95% v/v) was added. The ethanol was used to elute crystal violet on the biofilm, and then the diluted ethanol was transferred to a 1 cm quartz cuvette and measured by using a UV-Vis spectrophotometer (DR6000, HACH Company, USA) at 595 nm.
2.6 Surface chemistry
In order to study the changes of surface functional groups during field exposure, the plastic debris were analyzed by attenuated total reflection Fourier transformed infrared spectroscopy (ATR-FTIR) (Bruker TENSOR II, Germany). Before testing by ATR-FTIR, the biofilm on plastic debris should be removed. Briefly, the plastic debris were placed in a 1.5 mL EP tube, and 30% (v/v) HF was added to remove the organic matter attached on the surface.24 After 24 h, ultrasonic treatment was performed for 2 minutes. Then, the plastic debris were rinsed with Milli-Q water three times, and dried in an oven at 30 °C before ATR-FTIR was conducted. The spectral range was set at 400–4000 cm−1 with a resolution of 4 cm−1 for 32 scans. Indices of vinyl, carbonyl, and hydroxyl were calculated as the ratio of the maximum absorbance value for the bond peak relative to the value of a reference peak.25 The indices for PP were measured using the following formulae: vinyl index = I1640/I1465, carbonyl index = I1715/I1465, and hydroxyl index = I3370/I1465.25,26 The average index of three pieces of plastic debris was used for the analysis.
2.7 Surface hydrophobicity
The biofilm was removed from the plastic debris (the same method described in FTIR analysis), and then the hydrophobicity of plastic debris surfaces was measured by using a contact angle meter (JC2000D1, Shanghai Zhongchen Digital Technology Equipment Co., Ltd). Briefly, 1 μL distilled water was dropped onto the surface of plastic debris and the five-point fitting method was applied for calculating the contact angle of each sample. Three locations on each sample were randomly selected to measure the surface contact angle of plastic debris, and three pieces of plastic debris were selected as parallel samples.
2.8 Degree of crystallinity
X-ray diffraction (XRD) (Rigaku D/M 2500) was used to measure the changes in crystallinity of plastic debris, and triplicate samples were set up for analysis. The scattering angle was set at 5–30° with a scanning speed of 8° min−1.
2.9 Correlation analysis
Correlations between aging characteristics (include biomass, surface functional groups, hydrophobicity, and crystallinity) and environmental parameters were explored by redundancy analysis (RDA). RDA was carried out in R with the vegan 2.5-7 package.
2.10 Quality assurance
All plastic debris and nylon bags were sterilized with a 70% alcohol solution to remove bacteria and contaminants, and stored in sterile glass bottles before the field exposure experiments. The plastic debris harvested from the field were kept in ice bags during transportation. In the laboratory, the plastic debris were stored at −20 °C and water samples were stored at 4 °C. Cotton laboratory coats and gloves should be worn during the sample processing in the laboratory, and plastic debris should be covered with aluminum foil in time to avoid contamination. To ensure the reliability of the experimental data, three parallel samples were set up in this study. The data are shown in the figure as mean ± standard deviation (n = 3).
3 Results and discussion
3.1 Surface morphology of plastic debris
In the first sampling (two-week exposure), the surfaces of plastic debris were colonized by microorganisms. Photos of harvested plastic debris are shown in Table S2.† It can be seen that the biomass on the surface of plastic debris increased with the extension of field exposure time. The presence of algae and bacteria could be seen in the SEM micrographs (Fig. 2). The algae observed belong to many genera, including Ulothrix, Pinnularia, Nitzschia, Rhizosolenia, Navicula, Micrasterias, etc. The genera Navicula and Nitzschia, both of which belong to the diatoms, are commonly substrate-associated and known as biofilm formers in aquatic environments.17 On the surfaces of biofilms, no large number of bacterial cells was observed due to the coating of sediment particles.
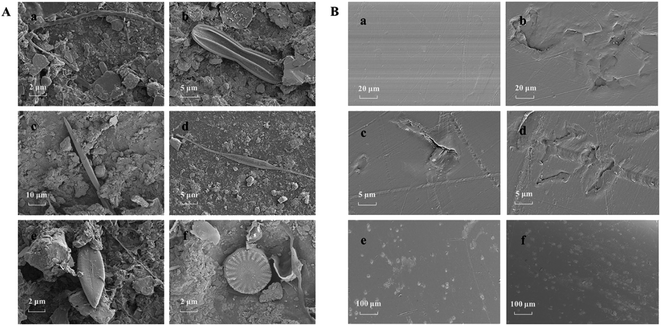 |
| Fig. 2 (A) Morphology of the biofilm on the surfaces of plastic debris: (a–f) Algae and bacteria on the surface of plastic debris. (B) Surface morphology of plastic debris: (a and b) morphology of plastic debris before and after pre-aging treatment; (c and d) morphology of pristine and pre-aged plastic debris after four-week field exposure; (e and f) morphology of pristine and pre-aged plastic debris after eight-week field exposure. | |
Before field exposure, the pristine plastic debris had a smooth surface, while the pre-aged plastic debris had some crack formation (Fig. 2B(a and b)). The surfaces of the pre-aged plastic debris developed more cracks and pitting corrosion than those of the pristine plastic debris after four-week (Fig. 2B(c and d)) and eight-week (Fig. 2B(e and f)) field exposure, respectively, indicating that the pre-aged plastic debris were more likely to be further eroded in a coastal environment.
3.2 Time-dependent variation of the biofilm content on the surfaces of plastic debris
During field exposure, biofilms were formed on the plastic debris. The biomass of the surface biofilm was quantified. It could be seen that the biomass on the surfaces of plastic debris increased with field exposure time (Fig. 3A). During the first four weeks, the biomass of the pristine plastic debris was not significantly different from that of the pre-aged plastic debris, which was due to the slow growth of biofilms on plastic surfaces in the early days. However, from the sixth to the tenth week, the biomass of pre-aged plastic debris was higher than that of the pristine plastic debris. From the second to the tenth week, the pre-aged plastic debris had an average of 17.24% higher surface biomass than that of the pristine plastic debris. Cracks on the surfaces of plastic debris were more conducive to the attachment of organisms. Moreover, plastic aging changed the properties of plastics, such as surface morphology, hydrophobicity, and chemical functional groups,26 which will facilitate the attachment of organisms. The formation of biofilms also changed the buoyancy of plastics in the water body, and thereby affected the vertical distribution of plastics in the water column,27 where the relevant environmental factors (such as temperature, microorganisms, etc.) at different depths could further affect plastic aging.16 In addition, though the biofilms on the plastic debris blocked UV degradation, they also increased the possibility of plastic biodegradation.
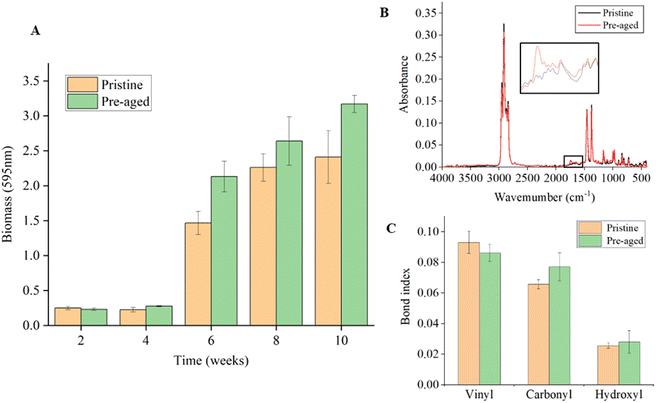 |
| Fig. 3 (A) Changes in biomass on the surfaces of plastic debris for each sampling. (B) The FTIR spectra of pristine and pre-aged plastic debris. (C) The functional group indices of pristine and pre-aged plastic debris. | |
3.3 Time-dependent variation of surface functional groups of plastic debris
The variation of functional groups is often considered as a sign of oxidation of plastic. As shown in Fig. 3B, FTIR spectra of plastic debris before and after pre-aging treatment were compared. The characteristic peaks of plastic debris in the range of 1550–1850 cm−1 and 3300–3400 cm−1 were changed after pre-aging treatment, and correspond to the functional groups of PP plastic including the vinyl group, carbonyl group and hydroxyl group. Pristine PP can show a higher vinyl index, which may be attributed to the β-scission of the polymer chain, favored by thermos-mechanical degradation during the pelletizing process of the polymers in the factory.28 The indices of these three functional groups related to plastic aging were calculated (Fig. 3C). Due to oxidation by UV radiation, the carbonyl and hydroxyl indices of pre-aged plastic debris slightly increased while the vinyl index decreased, which were consistent with the previous study.6 Photooxidation is the most common abiotic degradation pathway for plastic debris exposed to sunlight. During the induction period of PP photooxidation, the carbonyl index increased due to the stronger backbone scission.28 Hydroxyl groups were formed in the polymer structure in the form of hydroperoxide, alcohol and carboxylic acid groups.28 The decrease in the vinyl index was attributed to the tendency of this group towards crosslinking, or the sensitivity of the carbon–carbon double bond to UV light.28 The FTIR spectra of plastic debris during field exposure are shown in Fig. S3.† It can be observed that the characteristic peaks of pristine and pre-aged plastic debris varied in the range of 3300–3400 cm−1 and 1550–1850 cm−1. The time-dependent variation of the functional group index of plastic debris is shown in Fig. 4A. Although pristine and pre-aged plastic debris showed different functional groups at the beginning, both of them underwent a ten-week deployment in the same environment, leading to a similar functional group index change between pristine and pre-aged plastic debris. Besides, Fig. 4A shows that the vinyl index decreased with the increase in exposure time, which could be ascribed to the tendency of this group towards crosslinking, or the oxidation of carbon–carbon double bonds.28 Furthermore, both carbonyl and hydroxyl indices of the plastic debris were also decreased due to the comprehensive effects of environmental oxidation and biofilm utilization. The oxygen-containing functional groups of plastics would increase under environmental oxidation and decrease due to bioavailability.10,29 The exact mechanism of the big changes in the properties of plastics in 2–4 weeks remains to be studied, but they are likely to involve the comprehensive effects of the water environment and microbial attachment.
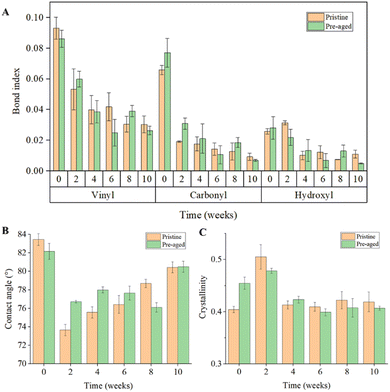 |
| Fig. 4 (A) The functional group indices of pristine and pre-aged plastics debris during field exposure. (B) Variation of the contact angle of plastic debris with field exposure time. (C) Variation of crystallinity of plastic debris with field exposure time. | |
3.4 Time-dependent variation of surface hydrophobicity of plastic debris
The water contact angle was widely used as an indicator to detect the hydrophobicity of materials. The larger the contact angle, the stronger the hydrophobicity of the material. As shown in Fig. 4B, both pristine and pre-aged plastic debris were hydrophobic as reflected by the initial large degree of contact angle at, which was attributed to the organic structure. Besides, before field exposure, the degree of contact angle of the pre-aged plastic debris was smaller than that of pristine plastic debris because of more carbonyl and hydroxyl groups and fewer vinyl groups. When the plastic debris was deployed in a coastal environment, the micro-morphological variation (Fig. 4B) might lead to reduced hydrophobicity reflected by the smaller degree of contact angle.16 However, with the prolongation of exposure time the degree of contact angle increased possibly due to the change in functional groups caused by environmental oxidation and biofilm utilization (Fig. 2). It should be also noted that the microorganisms preferred to colonize the hydrophobic surface,30 which further accelerated the increase in hydrophobicity.
3.5 Time-dependent variation of crystallinity of plastic debris
The X-ray diffraction curves of the PP plastic debris before and after the pre-aging treatment are shown in Fig. S4.† It can be seen that the intensity of the (110) and (300) crystal planes of plastic debris increased after the pre-aging treatment, while the intensity of the amorphous region decreased, which would present an increase in the crystallinity of the polymer. The tendency of the vinyl group towards crosslinking, or the sensitivity of the carbon–carbon double bond to UV light could result in a regular structure of PP and cause an increase in crystallinity (Fig. 2C). The X-ray diffraction curves of plastic debris during field exposure are shown in Fig. S5.† It was observed that both the crystalline and amorphous fractions of pristine and pre-aged plastic debris changed with field exposure, indicating a corresponding change in the crystallinity of the plastic debris. The crystallinity changes in plastic debris with the exposure time are shown in Fig. 4C. After two-week deployment, the crystallinity of pristine and pre-aged plastic debris increased because of the further oxidation of functional groups in a coastal environment. Meanwhile, the biofilm on the plastics began to form. One possible mechanism for the increase in crystallinity of all plastic debris in two weeks was that the biofilm facilitated the removal of low molecular weight components (such as monomers and dimers). The loss of these compounds contributed to the increase in polymer crystallization.31 During the field exposure of plastic debris, the chain scission caused by oxidation in the environment mainly occurred in the amorphous phase of the polymer.8 This fragmentation was thought to generate sufficient chain mobility to initiate secondary crystallization, leading to the increase in regulation.8 Some (surface)chemical aging and cross-linking processes may be responsible for the decreased crystallinity of pre-aged plastic debris.13 Niaounakis et al. observed that the crystallinity of PE decreased after 8–12 months in seawater, while the crystallinity increased after exposure to UV radiation in water for 2–3 days.13 This indicated that the crystallinity of plastic debris could be affected by the field exposure time.
3.6 Correlation analysis
The interpretation of the RDA map and the map with auxiliary marking are shown in Paragraph S1 and Fig. S6,† respectively. Using correlation analysis, the correlation between biomass and environmental parameters was analyzed. As shown in Fig. 5A, biomass was mainly positively correlated with dissolved oxygen, salinity and total organic carbon in 2–4 weeks. This indicated that the formation of biofilms on plastic debris mainly depended on dissolved oxygen and total organic carbon at the early stage, which provided the conditions for the formation and growth of biofilms. In a coastal environment, the attachment and survival of microorganisms need an appropriate range of salinity, so the growth of biofilm on the surface of plastic debris could also be affected by salinity. During a period of 6–10 weeks, biomass was positively correlated with temperature. Generally, metabolism would be accelerated by the increase in temperature. Therefore, the massive growth of biofilms on plastic surfaces from the sixth to eighth week could be detected (Fig. 3A).
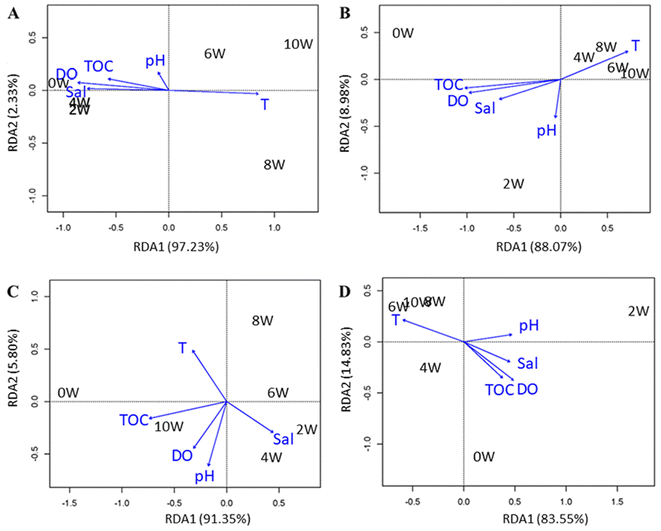 |
| Fig. 5 (A) Redundancy analysis (RDA) on the correlation between the biomass and environmental parameters. (B) The correlation between surface functional groups and environmental parameters. (C) The correlation between the contact angle and environmental parameters. (D) The correlation between crystallinity and environmental parameters. Environmental parameters include T (temperature), Sal (salinity), DO (dissolved oxygen), pH, and TOC (total organic carbon). | |
The correlation between changes in surface functional groups and environmental parameters was analyzed (Fig. 5B). The changes in functional groups on the surface of plastic debris were mainly positively correlated with temperature, which was the main factor to change the surface structure of plastic debris in seawater.
The correlation between the contact angle and environmental parameters was analyzed (Fig. 5C). It can be seen that the main environmental factor varied with the prolongation of the exposure time, indicating that the environmental conditions comprehensively affected the hydrophilicity of plastic debris. In addition, the formation of biofilms could also have an impact on the hydrophobicity of plastic debris. According to research, the decrease in the hydrophobicity of plastic surface may be mainly due to microbial activities, rather than long-term abiotic effects.32 Therefore, we believe that with the growth of biofilm during the cultivation process, the factors affecting the change of contact angle were also indirectly changing.
The correlation between crystallinity and environmental parameters was analyzed (Fig. 5D). Changes in crystallinity were mainly positively correlated with temperature. This indicated that temperature may have a greater impact on the variation of the plastic internal structure, comparing with other environmental factors.
4 Conclusions
The surface morphology and physicochemical properties of commercial PP plastic debris samples were changed after being exposed to a coastal environment for ten weeks. The surface of pre-aged plastic debris was more prone to form cracks and pitting than pristine plastic debris. Then, the surface of the pre-aged plastic debris was more likely to be attached by organisms than the pristine plastic debris. The process of biofouling can increase the density of plastics.15,24 When the density of plastic debris was higher than that of ambient water, the sedimentation of plastic debris would occur.24 However, due to temperature reduction and changes in environmental factors such as microbial products, defouling activities might occur on the surface of plastic debris, resulting in a decrease in density.24,33 In addition, the movement of plastic debris is not only caused by the movement of plastic debris itself, but is also driven by ocean currents.24 As for the changes in physicochemical properties (including the functional groups, hydrophobicity, and crystallinity), the plastic samples all changed significantly in the early stage (2–4 weeks) after they were placed in a coastal environment but they did not change much in the later stage. The change in the initial stage was probably due to environmental oxidation and leaching of low molecular weight components. As the culture time was increased, the differences between the physicochemical properties of the pristine and pre-aged plastic debris at the initial stage were eliminated. Due to the similar composition, the complexity of the aging environment and the difference among the parallel samples, the difference in physicochemical properties between the pristine and pre-aged plastic debris was not present. Based on the results of RDA, we found that temperature was a key factor which influenced the aging of plastics in a coastal environment. In summary, further research on the aging characteristics of plastics with aging history in a coastal environment is still needed in the future to gain an in-depth understanding on the aging characteristics of plastics and their threats to the environment. Given the strong persistence and stability of plastics in a coastal environment, it is still important and necessary to manage plastic waste properly and reduce the discharge of plastic into the coastal environment.
Author contributions
Shijia Guan: conceptualization, experimental execution, formal analysis, writing – original draft, writing – review and editing; Xiaohan Zhang: experimental execution, formal analysis; Yongzheng Ma: conceptualization, funding acquisition, supervision, writing – review and editing; Zhiguang Niu: conceptualization, funding acquisition, supervision, writing – review and editing.
Conflicts of interest
The authors declare that they have no known competing financial interests or personal relationships that could have appeared to influence the work reported in this paper.
Acknowledgements
This study was supported by the Fuzhou Science and Technology Bureau of China (2021-S-136).
References
- R. Geyer, J. R. Jambeck and K. L. Law, Production, use, and fate of all plastics ever made, Sci. Adv., 2017, 3, 25–29 CrossRef PubMed.
-
Plastics Europe, Plastic – the facts 2021 an analysis of European plastics production, demand and waste data, 2021 Search PubMed.
- M. Lv, B. Jiang, Y. Xing, H. Ya, T. Zhang and X. Wang, Recent advances in the breakdown of microplastics: strategies and future prospectives, Environ. Sci. Pollut. Res., 2022, 29, 65887–65903 CrossRef CAS PubMed.
- S. Zhang, J. Wang, P. Yan, X. Hao, B. Xu, W. Wang and M. Aurangzeib, Non-biodegradable microplastics in soils: a brief review and challenge, J. Hazard. Mater., 2021, 409, 124525 CrossRef CAS PubMed.
- A. ter Halle, L. Ladirat, X. Gendre, D. Goudouneche, C. Pusineri, C. Routaboul, C. Tenailleau, B. Duployer and E. Perez, Understanding the fragmentation pattern of marine plastic debris, Environ. Sci. Technol., 2016, 50, 5668–5675 CrossRef CAS PubMed.
- M. Dong, Q. Zhang, X. Xing, W. Chen, Z. She and Z. Luo, Raman spectra and surface changes of microplastics weathered under natural environments, Sci. Total Environ., 2020, 739, 139990 CrossRef CAS PubMed.
- A. L. Andrady and J. E. Pegram, Weathering of polystyrene foam on exposure in air and in seawater, J. Appl. Polym. Sci., 1991, 42, 1589–1596 CrossRef CAS.
- S. A. Jabarin and E. A. Lofgren, Photooxidative effects on properties and structure of high-density polyethylene, J. Appl. Polym. Sci., 1994, 53, 411–423 CrossRef CAS.
- M. Santo, R. Weitsman and A. Sivan, The role of the copper-binding enzyme - laccase - in the biodegradation of polyethylene by the actinomycete Rhodococcus ruber, Int. Biodeterior. Biodegrad., 2013, 84, 204–210 CrossRef CAS.
- S. Zahra, S. S. Abbas, M. T. Mahsa and N. Mohsen, Biodegradation of low-density polyethylene (LDPE) by isolated fungi in solid waste medium, Waste Manage., 2010, 30, 396–401 CrossRef CAS PubMed.
- J. E. Pegram and A. L. Andrady, Outdoor weathering of selected polymeric materials under marine exposure conditions, Polym. Degrad. Stab., 1989, 26, 333–345 CrossRef CAS.
- J. Brandon, M. Goldstein and M. D. Ohman, Long-term aging and degradation of microplastic particles: comparing in situ oceanic and experimental weathering patterns, Mar. Pollut. Bull., 2016, 110, 299–308 CrossRef CAS PubMed.
- M. Niaounakis, E. Kontou, S. Pispas, M. Kafetzi and D. Giaouzi, Aging of packaging films in the marine environment, Polym. Eng. Sci., 2019, 59, E432–E441 CrossRef CAS.
- J. P. Harrison, M. Schratzberger, M. Sapp and A. M. Osborn, Rapid bacterial colonization of low-density polyethylene microplastics in coastal sediment microcosms, BMC Microbiol., 2014, 14, 1–15 CrossRef PubMed.
- M. Long, B. Moriceau, M. Gallinari, C. Lambert, A. Huvet, J. Raffray and P. Soudant, Interactions between microplastics and phytoplankton aggregates: impact on their respective fates, Mar. Chem., 2015, 175, 39–46 CrossRef CAS.
- C. Tu, T. Chen, Q. Zhou, Y. Liu, J. Wei, J. J. Waniek and Y. Luo, Biofilm formation and its influences on the properties of microplastics as affected by exposure time and depth in the seawater, Sci. Total Environ., 2020, 734, 139237 CrossRef CAS PubMed.
- E. R. Zettler, T. J. Mincer and L. A. Amaral-Zettler, Life in the ‘plastisphere’: microbial communities on plastic marine debris, Environ. Sci. Technol., 2013, 47, 7137–7146 CrossRef CAS PubMed.
- W. Zhang, S. Zhang, J. Wang, Y. Wang, J. Mu, P. Wang, X. Lin and D. Ma, Microplastic pollution in the surface waters of the Bohai Sea, China, Environ. Pollut., 2017, 231, 541–548 CrossRef CAS PubMed.
- N. Wu, Y. Zhang, X. Zhang, Z. Zhao, J. He, W. Li, Y. Ma and Z. Niu, Occurrence and distribution of microplastics in the surface water and sediment of two typical estuaries in Bohai Bay, China, Environ. Sci.: Processes Impacts, 2019, 21, 1143–1152 RSC.
- W. Li, Y. Zhang, N. Wu, Z. Zhao, W. Xu, Y. Ma and Z. Niu, Colonization characteristics of bacterial communities on plastic debris influenced by environmental factors and polymer types in the Haihe Estuary of Bohai Bay, China, Environ. Sci. Technol., 2019, 53, 10763–10773 CrossRef CAS PubMed.
- R. Wang, K. G. Neoh, Z. Shi, E. T. Kang, P. A. Tambyah and E. Chiong, Inhibition of Escherichia coli and Proteus mirabilis adhesion and biofilm formation on medical grade silicone surface, Biotechnol. Bioeng., 2012, 109, 336–345 CrossRef CAS PubMed.
- D. Lobelle and M. Cunliffe, Early microbial biofilm formation on marine plastic debris, Mar. Pollut. Bull., 2011, 62, 197–200 CrossRef CAS PubMed.
- C. De Tender, L. I. Devriese, A. Haegeman, S. Maes, J. Vangeyte, A. Cattrijsse, P. Dawyndt and T. Ruttink, Temporal dynamics of bacterial and fungal colonization on plastic debris in the North Sea, Environ. Sci. Technol., 2017, 51, 7350–7360 CrossRef CAS PubMed.
- N. Wu, Y. Zhang, W. Li, J. Wang, X. Zhang, J. He, J. Li, Y. Ma and Z. Niu, Co-effects of biofouling and inorganic matters increased the density of environmental microplastics in the sediments of Bohai Bay coast, Sci. Total Environ., 2020, 717, 134431 CrossRef CAS PubMed.
- V. Fernández-González, J. M. Andrade-Garda, P. López-Mahía and S. Muniategui-Lorenzo, Impact of weathering on the chemical identification of microplastics from usual packaging polymers in the marine environment, Anal. Chim. Acta, 2021, 1142, 179–188 CrossRef PubMed.
- T. Artham, M. Sudhakar, R. Venkatesan, C. Madhavan Nair, K. V. G. K. Murty and M. Doble, Biofouling and stability of synthetic polymers in sea water, Int. Biodeterior. Biodegrad., 2009, 63, 884–890 CrossRef CAS.
- C. D. Rummel, A. Jahnke, E. Gorokhova, D. Kühnel and M. Schmitt-Jansen, Impacts of biofilm formation on the fate and potential effects of microplastic in the aquatic environment, Environ. Sci. Technol. Lett., 2017, 4, 258–267 CrossRef CAS.
- K. Rajakumar, V. Sarasvathy, A. Thamarai Chelvan, R. Chitra and C. T. Vijayakumar, Natural weathering studies of polypropylene, J. Polym. Environ., 2009, 17, 191–202 CrossRef CAS.
- A. Tidjani, Comparison of formation of oxidation products during photo-oxidation of linear low density polyethylene under different natural and accelerated weathering conditions, Polym. Degrad. Stab., 2000, 68, 465–469 CrossRef CAS.
- R. M. Donlan, Biofilms: microbial life on surfaces, Emerging Infect. Dis., 2002, 8, 881–890 CrossRef PubMed.
- E. McGivney, L. Cederholm, A. Barth, M. Hakkarainen, E. Hamacher-Barth, M. Ogonowski and E. Gorokhova, Rapid physicochemical changes in microplastic induced by biofilm formation, Front. Bioeng. Biotechnol., 2020, 8, 1–14 CrossRef PubMed.
- S. Krause, M. Molari, E. V. Gorb, S. N. Gorb, E. Kossel and M. Haeckel, Persistence of plastic debris and its colonization by bacterial communities after two decades on the abyssal seafloor, Sci. Rep., 2020, 10, 1–15 CrossRef PubMed.
- A. J. Pete, P. J. Brahana, M. Bello, M. G. Benton and B. Bharti, Biofilm formation influences the wettability and settling of microplastics, Environ. Sci. Technol. Lett., 2022 DOI:10.1021/acs.estlett.2c00728.
Footnote |
† Electronic supplementary information (ESI) available: The geographic location and environmental parameters of the sampling site in Bohai Bay, China (Table S1); photos of the harvested plastic debris (Table S2); the spectrum of the UV lamp used for pre aging (Fig. S1); the photo of the harvested nylon bag containing plastic samples (Fig. S2); the FTIR spectra of pristine (a) and pre-aged (b) plastic debris during field exposure (Fig. S3); the X-ray diffraction curves of pristine and pre-aged plastic debris (Fig. S4); the X-ray diffraction curves of pristine (a) and pre-aged (b) plastic debris during field exposure (Fig. S5); the RDA map with auxiliary marking (take Fig. 5A as an example) (Fig. S6); the interpretation of the RDA map (Paragraph S1). See DOI: https://doi.org/10.1039/d2em00432a |
|
This journal is © The Royal Society of Chemistry 2023 |
Click here to see how this site uses Cookies. View our privacy policy here.