The effects of emerging contaminants on the behaviour of Acinetobacter calcoaceticus derived from biofilms
Received
8th April 2022
, Accepted 14th November 2022
First published on 14th November 2022
Abstract
Knowledge regarding the ecotoxicological effects of emerging contaminants (EC) in drinking water distribution system (DWDS) microbiota is limited. Given the issues associated with biofilm formation in DWDS, this work studied the effects of the exposure of Acinetobacter calcoaceticus biofilms to four pharmaceutical compounds already encountered in drinking water (DW) at residual concentrations (ng L−1): caffeine – CAF, carbamazepine – CBZ, ciprofloxacin – CIP and ibuprofen – IBP. The exposure assays were performed with the compounds alone and in combination (MIX). The results obtained aim to contribute for the understanding of the role of ECs exposure for 7 days in bacterial behaviour, particularly the ability to form biofilms, susceptibility to antibiotics (CIP, levofloxacin – LEV, tetracycline – TET and trimethoprim–sulfamethoxazole – TMP-SMX), tolerance to NaOCl, motility and growth rate. It was found that IBP exposure decreased biofilm formation ability by 11%, while MIX exposure increased it by 16%. Regarding antibiotics susceptibility, CAF exposure decreased susceptibility to TPM-SMX, while CBZ and CIP exposures seem to cause an increase in the susceptibility to TMP-SMX. Furthermore, exposure to residual levels of CIP decreased the susceptibility to CIP and LEV, and IBP exposure seems to slightly decrease the susceptibility to LEV and TET. The ranges of minimum bactericidal concentration (MBC) of NaOCl have also slightly increased (for CIP and IBP exposure) or decreased (for the exposure to CBZ). Higher A. calcoaceticus growth rates were obtained after CIP exposure. These results demonstrate that the selected ECs affect the behaviour and growth dynamics of bacteria under conditions typically found in DWDS. Among the selected ECs, CIP and IBP were those causing the most significant effects. Furthermore, this study lays the groundwork for knowledge enrichment regarding bacterial behaviour in biofilms and their impact on DWDS.
Water impact
The occurrence of emerging contaminants (ECs) in drinking water (DW) is unquestionable, as well as the unavoidable biofilm formation in DW distribution systems (DWDS). However, the exposure of DW biofilms to ECs is a reality often disregarded, and the effects of this exposure may affect the water quality. It is crucial to recognize the ECs impact on DW biofilms for ECs prioritization.
|
1. Introduction
Emerging contaminants (ECs), otherwise described as micropollutants1,2 or contaminants of emerging concern,3 are organic or inorganic4,5 occurring compounds (chemicals or microorganisms) of anthropogenic or natural origin,1 found in the environment at residual concentrations (ng L−1 and μg L−1).1,4,6 Due to the extensive and abusive use of ECs, including the class of pharmaceuticals, they are continuously released into the environment. However, the processes used in water treatment plants (WTP) do not specifically target and effectively eliminate ECs from their influents,6–8 due to their complex structures and varied physicochemical properties.9 Pharmaceuticals have since been detected in a myriad of aquatic environments, including wastewater, water treatment plants (WTPs), surface waters and groundwater.10–12 As surface and groundwater are commonly used as sources of water for consumption, they end up becoming sources of ECs in drinking water (DW)10,13 and, according to the World Health Organization (WHO), their occurrence in DW could pose a threat to the environment and human health.14
Adverse ECs effects have been registered in aquatic plants,15 invertebrates16 or animals from higher trophic levels, such as fish.17,18 However, ecotoxicological effects are also observed in microbial communities, i.e., biofilms in aquatic environments,19 concerning biofilm structure, function and antibiotic resistance profiles.7,20–23 Therefore, instinctively, there is a growing concern about the possible impact resulting from phenotypic and genotypic alterations of microbial behaviour due to ECs exposure, particularly in drinking water distribution systems (DWDS), where biofilms actively threaten the quality and safety of the distributed DW.24–28 However, research in this field is still exceedingly limited, particularly in the DW scenario. Only five studies, known to date, were found to have sought to determine the extent of ECs exposure impact on DWDS microbiota.29–33
It is estimated that 95% of the total DW biomass adheres to pipe surfaces (sessile state),34 displaying a higher tolerance to environmental adversities than their suspended counterparts (planktonic state).35 Biofilms in DWDS are often associated with: (1) the incidence of water-borne diseases24 and/or proliferation of water quality-compromising bacteria (i.e., generating visual turbidity and altering organoleptic properties of DW), (2) increase of hydraulic drag, (3) promotion of biocorrosion, (4) decreased efficiency of disinfection processes25–27 and (5) facilitation of antibiotic resistance transfer.28 Given the reports on the presence of pharmaceuticals in DW,3,10,13,36,37 it is possible to understand that biofilms in DWDS and premise plumbing are constantly exposed to trace concentrations of these pharmaceutical compounds. Although the concentrations of ECs found in DW are residual, the alteration of microbial communities and biofilm characteristics may occur, affecting biofilm formation and control in DWDS. Additionally, antibiotics not only exert toxic effects on microbial communities but may also be responsible for exerting selective pressure,38–40 contributing to the continued selection and proliferation of antibiotic-resistant bacteria (ARB) and antibiotic-resistant genes (ARGs). Exposure to sub-inhibitory concentrations is the main cause of resistance promotion.41,42 However, little is known about the influence of non-antibiotic pharmaceuticals on this phenomenon.43 Non-antibiotic ECs also exert selective pressure and their presence is related to increased resistance to disinfection of microorganisms in biofilms, yet, this topic is rather unmentioned.44 Lu et al.44 reported that exposure to triclosan resulted in the spread of ARGs. Wang et al.43 observed that carbamazepine exposure resulted in an increase in SOS response, membrane permeability and in the generation of pilus, which contributes to greater efficiency in resistance transfer. These results prove that, even at environmentally relevant concentrations, antibiotics or non-antibiotics pharmaceuticals can promote the dissemination of ARGs and the acquisition of resistance by the microorganisms exposed.
The main goal of this study is to provide insight into the effects of pharmaceuticals, antibiotics and non-antibiotics, at residual concentrations (ng L−1 and μg L−1), on biofilms from Acinetobacter calcoaceticus isolated from DW. The parameters evaluated in this study aim to predict and get a better understanding of the possible effects of the selected ECs on bacteria from DW. For that, biofilms were exposed to the selected ECs under low nutrient concentrations (mimicking the DW). Then, the impact of ECs was evaluated on the biofilm cellular content, bacterial growth rate, ability to form biofilms, susceptibility to antibiotics, tolerance to disinfection by NaOCl and motility.
2. Methods
2.1. Emerging contaminants
Four ECs were selected for this study, each representing a different class of pharmaceuticals: a central nervous system stimulant (caffeine – CAF (Fluka, Steinheim, Germany)); an anticonvulsant (carbamazepine – CBZ (Acros Organics, New Jersey, USA)); an antibiotic, more specifically, a fluoroquinolone (ciprofloxacin – CIP (Fluka, Steinheim, Germany)) and a non-steroidal anti-inflammatory drug (NSAID) (ibuprofen – IBP (Alfa Aesar, Karlsruhe, Germany)). The exposure assays were conducted at residual concentrations (ng L−1), thus reflecting the conditions of DW (Table 1). The concentrations tested were selected attending the highest levels reported in DW between 2009 and 2019, as reviewed by Gomes et al.45 Furthermore, the combination of previously mentioned ECs was also tested (MIX), in which the concentration of each EC corresponds to the concentration of the individual contaminant. Stock solutions of CBZ and IBP were prepared using dimethyl sulfoxide (DMSO) (Fisher Scientific, Leicestershire, UK), while CAF and CIP stock solutions were prepared in sterile distilled water. Stock solutions were stored at −20 °C until further use. The final concentration of DMSO used is residual, varying from 0.0002 and 0.0005% (v/v).
Table 1 Selected emerging contaminants: concentrations found in DWDS and other specific information
EC |
Abbreviation |
Class of contaminant |
Registered concentration (ng L−1) |
Ref. |
Caffeine |
CAF |
Central nervous system stimulant |
158.7 |
13
|
Carbamazepine |
CBZ |
Anticonvulsant |
586 |
3
|
Ciprofloxacin |
CIP |
Antibiotic |
679.7 |
97
|
Ibuprofen |
IBP |
Non-steroidal anti-inflammatory drug |
223.6 |
13
|
2.2. Microorganism and culture media
Acinetobacter calcoaceticus, previously isolated from DW,46 was used as DW bacteria model. A. calcoaceticus was grown overnight in batch cultures on 25 mL of R2A broth (0.5 g L−1 peptone (Oxoid, Hampshire, England); 0.5 g L−1 glucose (CHEM-LAB, Zedelgem, Belgium); 0.1 g L−1 of hepta-hydrated magnesium sulphate (Merck, Darmstadt, Germany); 0.3 g L−1 sodium pyruvate (Fluka, Steinheim, Germany); 0.5 g L−1 yeast extract (Merck, Darmstadt, Germany); 0.5 g L−1 of casein hydrolysate (Oxoid, Hampshire, England); 0.5 g L−1 of soluble starch (Sigma) and 0.4 g L−1 of di-potassium phosphate trihydrate (Applichem Panreac, Darmstadt, Germany)) at 25 °C and under 120 rpm agitation. Bacterial cells were later harvested by centrifugation (Eppendorf centrifuge 5810R, Germany) at 3700 g for 15 min, and resuspended in R2A broth.
2.3. Biofilm exposure to emerging contaminants
2.3.1. Substratum.
PVC was chosen as a representative material of DWDS pipes. Before use, PVC (Neves & Neves, Portugal) coupons (0.9 × 1 cm) were sterilized and later mounted into 48-wells microtiter plates, as described by Gomes et al.30
2.3.2. Biofilm formation and exposure to emerging contaminants.
The experimental exposure setup comprised two main steps: (1) biofilm pre-establishment (Fig. 1.A and B) and (2) biofilm exposure to ECs (Fig. 1.C). Firstly, bacterial cells (from section 2.2) were adjusted to 107 cells per mL with sterile R2A broth (reflecting the number of heterotrophic bacteria in DWDS47), and primordial surface colonization was performed in the presence of selected ECs (in DWDS equivalent concentrations) for 24 h, at 25 °C and 120 rpm agitation (Fig. 1.A). The total number of cells per mL was confirmed through epifluorescence microscopy (LEICA DM LB2 epifluorescence microscope connected to a Leica DFC300 FX camera -Leica Microsystems Ltd, Heerbrugg) by staining with 4′,6-diamidino-2-phenylindole (DAPI).48 Then, the cell suspension was replaced by 1 mL of sterile R2A broth, prepared with ECs or DMSO solutions, as shown in Fig. 1.B, to renew nutrients and reinforce bacterial growth. After an additional 12 h of biofilm pre-establishment period, bacterial suspensions were replaced by the solutions of ECs prepared in synthetic tap water – STW according to Gomes et al.30 (100 mg L−1 of NaHCO3 (Fisher Scientific, Leicestershire, UK); 13 mg L−1 of MgSO4·7H2O (Merck, Darmstadt, Germany); 0.7 mg L−1 of K2HPO4 (Applichem Panreac, Darmstadt, Germany); 0.3 mg L−1 of KH2PO4 (CHEMLAB, Zedelgem, Belgium); 0.01 mg L−1 of (NH4)2SO4 (Labkem, Barcelona, Spain); 0.01 mg L−1 of NaCl (Merck, Darmstadt, Germany); 0.001 mg L−1 of FeSO4·7H2O (VWR PROLABO, Leuven, Belgium); 1 mg L−1 of NaNO3 (Labkem, Barcelona, Spain); 27 mg L−1 of CaSO4 (Labkem, Barcelona, Spain) and 1 mg L−1 of humic acids (Sigma-Aldrich, Steinheim, Germany)). The colonized coupons were then incubated at 25 °C and 120 rpm, for five additional days (Fig. 1.C). Biofilms were formed for 7 days. This period of time was described as adequate to form mature and stable (steady-state) A. calcoaceticus biofilms.49 This process required a washing step before STW replacement, to remove the remaining nutrients and weakly bonded and non-adhered bacteria. The use of STW aims to create a nutrient-deficient environment mimicking the conditions found in DWDS. In this work, two controls were performed: CONT- biofilms grown in the culture medium and exposed only to STW and SOLV- biofilms exposed to DMSO at 0.0005% (v/v) (solvent control). Exposure to ECs for 7 days was reproduced in two independent assays, with duplicates.
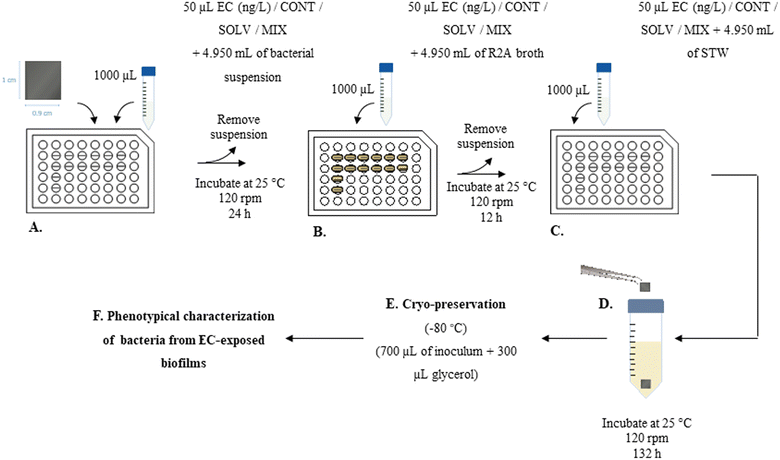 |
| Fig. 1 Experimental set-up: biofilm formation and exposure (A – microplate inoculation by adding bacterial suspension at adjusted concentration and the corresponding EC; B – exchange and renewal of nutrients; C – exposure to ECs, under environmental conditions (low nutrient availability); D – harvesting A. calcoaceticus from exposed biofilms; E – cryo-preservation of bacteria from ECs-exposed biofilms; F – phenotypic characterization of A. calcoaceticus from EC-exposed biofilms). | |
2.4. Characterization of bacteria from EC-exposed biofilms
At the end of the exposure period, coupons were carefully removed from the wells and harvested through vigorous agitation for 2 min in a vortex at the maximum velocity, to promote dissociation of the cells from the PVC surface into the sterile R2A broth. The resulting bacterial suspension was incubated at 25 °C and 120 rpm, overnight, and then cryo-preserved at −80 °C. For that, 700 μL of each inoculum was added to sterile cryotubes containing 300 μL of glycerol (Fig. 1.E). Then, cryopreserved exposed bacteria (50 μL) were inoculated in 10 mL of R2A broth or Muller Hinton (MH) broth (for section 2.4.2), and incubated at 25 °C and 120 rpm, overnight, for further characterization of A. calcoaceticus from EC-exposed biofilms (Fig. 1.F), specifically in terms of growth rate (section 2.4.1), susceptibility to antibiotics (section 2.4.2), NaOCl minimum bactericidal concentration (section 2.4.3), ability to produce biofilms (section 2.4.4) and motility (section 2.4.5). To evaluate the impact of cryopreservation and subsequent culturing procedure on the evaluation of ECs effect on bacterial behaviour, all the methodologies were implemented directly from biofilm harvested cells. Cryopreservation and subsequent culturing procedures did not alter the results obtained in comparison to the use of bacteria directly form biofilms.
2.4.1.
A. calcoaceticus growth rate.
A. calcoaceticus growth rate was estimated by monitoring the optical density (OD) of bacterial suspensions (with an initial cell concentration of 107 cells per mL in R2A) for 24 h in intervals of 30 min, at 600 nm, using a microtiter plate reader (SPECTROstar Nano, BGM LABTECH).50 Data were plotted as log (OD) over time and the exponential phase was identified, which allowed the determination of a specific growth rate (h−1, corresponding to the slope of the exponential phase), as well as the doubling time (td, expressed in h) that is given by eqn (1): |  | (1) |
The assays were performed in 96 well microtiter plates, in three independent assays (with triplicates), for each one of the independent exposure assays performed (section 2.3.2).
2.4.2. Susceptibility to antibiotics.
A. calcoaceticus susceptibility to antibiotics was determined by the disk diffusion method, according to the Clinical and Laboratory Standards Institute (CLSI) guidelines.51 The antibiotics selected for this study are commonly used for the treatment of A. calcoaceticus infections51 and are listed and described in Table 2. Briefly, an aliquot of 100 μL of bacterial suspension (6 × 108 cells per mL) was spread on MH agar plates and 10 μL of antibiotic, at proper concentration, were placed on sterile paper discs. Control tests were performed using the antibiotic solvent, DMSO. Plates were further incubated at 37 °C for 24 h. Each exposure condition was tested in three independent assays (with duplicates), for each one of the two independent exposure assays (section 2.3.2). After the incubation period, inhibition halos (mm) were measured and the results are presented as average ± standard deviation (SD).
Table 2 Antibiotics used for the evaluation of antibiotic susceptibility of exposed A. calcoaceticus, according to the CLSI standards for antibiogram testing by the disc diffusion method
Antibiotic |
Abbreviation |
CLSI guidelines (μg/disc) |
Ciprofloxacin |
CIP |
5 |
Levofloxacin |
LEV |
5 |
Tetracycline |
TET |
20 |
Trimethoprim-sulfamethoxazole |
TMP-SMX |
1.25/23.75 |
2.4.3. The minimum bactericidal concentration of NaOCl.
To evaluate the susceptibility of bacteria from EC-exposed biofilms to sodium hypochlorite – NaOCl, the minimum bactericidal concentration (MBC) was determined according to Gomes et al.32 Different concentrations of NaOCl (Sigma-Aldrich, Steinheim, Germany) (1–10 mg of free chlorine/L) were used to determine the minimum concentration of free chlorine necessary to inactivate the growth of A. calcoaceticus in STW – (MBC). Briefly, free chlorine concentrations were adjusted using a test kit by Hanna Instruments (using a photometer and the N,N.diethyl-p-phenylenediamine (DPD) method), as described by Gomes et al.32 Tests were performed in 96-well microplates, where 20 μL of prepared NaOCl solution (or 20 μL of STW in case of the negative control) and 180 μL of bacterial suspension (at 106 cells per mL in STW) were added to each well. STW was used to simulate the low availability of nutrients found in DWDS. Microplates were incubated at 25 °C and 120 rpm for 24 h. After incubation, 180 μL of each well was discarded and 180 μL of sodium thiosulfate (0.5% w/v) (Labkem, Spain) was added to neutralize NaOCl. Subsequently, 20 μL of this suspension was transferred to R2A agar and incubated at 25 °C for 48 h. The MBC of NaOCl corresponded to the lowest concentration at which no A. calcoaceticus growth was observed. Three independent assays were performed (with duplicates), for each one of the two independent exposure assays (section 2.3.2).
2.4.4.
A. calcoaceticus biofilm production: Biomass quantification.
To assess A. calcoaceticus ability to form biofilms after exposure to ECs, the crystal violet assay was used as a quantitative method, as described by Stepanović et al.52 Briefly, 200 μL of a bacterial suspension at 107 cells per mL (in R2A broth) were inserted into 96 well microtiter plates. The plates were then incubated at 25 °C and 120 rpm for 24 h. Sterile R2A medium was used as the negative control. After incubation, bacterial suspensions were carefully discarded and wells were washed with 200 μL of STW to remove non-adhered bacteria. Afterwards, 250 μL of absolute ethanol (Fisher, Leicestershire, England) was used to fix the biofilms (incubating for 15 minutes and leaving to dry for 5 min at room temperature). Crystal violet (Merck, Darmstadt, Germany) (1% (v/v)) was then added (200 μL) to stain biofilms for 5 minutes. After this period, the crystal violet solution was discarded and acetic acid (33% (v/v)) was used to elute leftover crystal violet from the staining process. Absorbance was measured using a microtiter plate reader (SPECTROstar Nano, BGM LABTECH), at 570 nm. This test was carried out in three independent assays (with five replicates), for each one of the two independent exposure assays (section 2.3.2) performed.
2.4.5. Motility assay.
To determine the effect induced by exposure to ECs on A. calcoaceticus motility, twitching, swarming and swimming motility were tested according to Gomes et al.48 Briefly, 15 μL of each exposed bacterial suspension (107 cells per mL in saline solution) was placed in the centre of plates composed by 1% tryptone, 0.25% NaCl and 0.3% (swimming), 0.7% (swarming) or 1.5% (twitching) agar (w/v).53,54 Plates were incubated at 25 °C, and motility halos were measured after 48 h of incubation (mm). These tests were performed in three independent assays (with duplicates), for each one of the two independent exposure assays (section 2.3.2) performed.
2.5. Statistical analysis
Statistical analysis of acquired data was accomplished by applying one-way analysis of variance (ANOVA), and comparisons between and within experimental groups were performed using the Tukey test. These calculations were based on a confidence level ≥95% (i.e., for a P value lesser than 0.05, the result is considered statistically significant). Data were processed using Microsoft Excel, version 16.53.
3. Results and discussion
3.1. Effect of ECs exposure on A. calcoaceticus growth rate
Biofilm formation accounts for the majority of microbial load in DWDS, however, bulk phase growth in DW also occurs along DWDS under nutrient deficiency conditions.55 The environmental history and physiological state56 are known to affect the bacteria growth rate. The hypothesis of an increased and faster regrowth of suspended bacteria in the bulk water due to ECs exposure would represent critical issues for the management of DW quality. Fig. 2 presents the specific growth rate (μ − h−1) and doubling time (td − h) of A. calcoaceticus from non-exposed and ECs-exposed biofilms. Higher specific growth rates were found for A. calcoaceticus biofilms exposed to ECs, specifically for CIP, which caused a significant impact on the specific growth rate and doubling time (P < 0.05), increasing μ by 90.5%, and td by 51.2%. This phenomenon has been often described as an adaptation to improve stress tolerance since several studies demonstrated that although toxic substances may inhibit microbial growth, the long-term exposure to sub-inhibitory concentrations of a toxic substance may result in an improvement in microbial ability to grow in the presence of these substances or even metabolize them.57 Shaker et al.58 reported that Acinetobacter baumannii can degrade fluoroquinolones (CIP and LEV). Kim et al.59 demonstrated that the previous adaptation of E. coli to formic acid also resulted in a reduction in the doubling time. Moreover, Tuan-Anh et al.60 also described an increased growth rate for pathogenic E. coli exposed to sub-inhibitory concentrations of azithromycin.
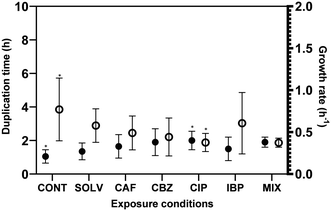 |
| Fig. 2 Effect of ECs exposure on growth rate (●) and doubling time (○) of A. calcoaceticus (25 °C, 500 rpm). The values reported are the means ± standard deviation of three independent assays with triplicates, for each one of the two independent exposure assays. CONT – control, Solv – DMSO solvent control, CAF – caffeine, CBZ – carbamazepine, CIP – ciprofloxacin, IBP – ibuprofen, MIX – mixture of ECs, μ is the specific growth rate (h−1), td is the doubling time (h), * corresponds to results with statistically significant differences in comparison to the CONT (P < 0.05). | |
3.2. Effect of ECs exposure on A. calcoaceticus NaOCl susceptibility
A better understanding of the extent of biocidal action of disinfectants is extremely important to apply more adequate disinfection strategies and deliver efficient microbial control within DWDS.61 The inefficiency of these treatments, is of utmost concern, since it hampers the biological control of DW, constitutes a biological hazard and contributes to the spread of antibiotic resistance, and selection and proliferation of ARB.62,63 The susceptibility of A. calcoaceticus from EC-exposed biofilms to free chlorine was evaluated in terms of MBC. Results are presented in Fig. 3.
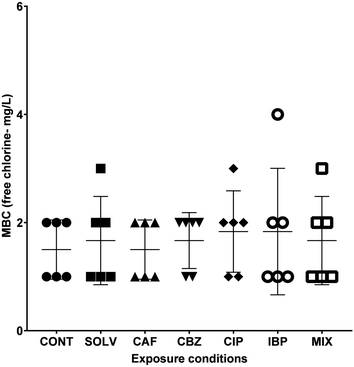 |
| Fig. 3 The minimum bactericidal concentration of NaOCl (mg L−1) after A. calcoaceticus exposure to ECs for 7 days. The MBC values from three independent assays, for each one of the two independent exposure assays, are represented by each dot. The mean and standard deviation to each exposure condition is also presented. CONT – control, Solv – DMSO solvent control, CAF – caffeine, CBZ – carbamazepine, CIP – ciprofloxacin, IBP – ibuprofen, MIX – mixture of ECs. | |
Complete inactivation of A. calcoaceticus (CONT) occurred for concentrations between 1.0 and 2.0 mg L−1, and the exposure to DMSO caused a slight increase in the MBC range (1.0–3.0 mg L−1), however the alterations are not statistically significant. The results reveal that CIP exposure (when compared to non-exposed biofilms – CONT) and IBP (when compared to DMSO solvent control) may cause a slight increase in A. calcoaceticus tolerance to NaOCl, with MBC values at concentrations of 1.0–3.0 mg L−1 of free chlorine and 1.0–4.0 mg L−1 of free chlorine, respectively. On the other hand, CBZ exposure may be responsible for the increase of A. calcoaceticus susceptibility to NaOCl. However, the statistical analysis did not validate these differences as significant. Similarly, Gomes et al.29 determined that Burkholderia cepacia became more susceptible to NaOCl after biofilm exposure to CBZ at 258 ng L−1 for 24 h. However, although an increase in susceptibility could be beneficial for microbial control in DWDS, chlorine disinfection can select antibiotic-resistant bacteria and increase the relative abundance of ARGs.64 In DWDS, the minimum recommended residual free chlorine concentration is 0.2 mg L−1 as per WHO standards,65 and it is expected not to exceed 2 mg L−1, due to taste concerns.66 Since CIP and IBP exposure further expanded A. calcoaceticus susceptibility range, the presence of these ECs in DW could be of concern regarding the efficacy of free chlorine on bacterial control in bulk water. Furthermore, the Environmental Protection Agency (EPA) indicates that chlorine levels up to 4 mg L−1 are considered safe in DW, as harmful health effects are unlikely to occur.67 Therefore, to efficiently inhibit the growth of A. calcoaceticus from IBP-exposed biofilms a minimum concentration of 4 mg L−1 of free chlorine may be necessary. The use of 4 mg L−1 of free chlorine in DW would not compromise its safety. However, at this free chlorine concentration, some DW organoleptic properties, such as taste, may be compromised, as well as the formation of harmful disinfection by-products.68
3.3. Effect of ECs exposure on A. calcoaceticus antibiotic susceptibility
The development of antimicrobial resistance due to trace concentrations of ECs has been reported in various environments.39,69,70 And, A. calcoaceticus tends to acquire and disseminate antibiotic resistance determinants,71 making this a concern for public health. Increased antibiotic resistance may result in enhanced virulence, pathogenicity, disease outbreaks and transmission, as the consumer is directly exposed to antibiotic-resistant pathogens through consumption of contaminated water.72
The CLSI standards define the breakpoints for which a particular bacterial strain is considered resistant (R) or susceptible (S) to an antibiotic. According to CLSI standards,73 inhibition halos diameter ≥ 0.21, ≥ 0.17, ≥ 0.15 and ≥ 0.16 mm, for CIP, LEV, TET and TMP-SMX, respectively, indicate that Acinetobacter spp. is considered susceptible to each of these antibiotics. Inhibition halos correspond to the area in MH agar plate where bacteria were not able to grow due to the action of antibiotics. Fig. 4 suggests that A. calcoaceticus is susceptible to all antibiotics analysed, regardless of the exposure conditions. A 7 days of exposure to ECs (antibiotics and non-antibiotics), at residual concentrations (ng L−1), affected the tolerance of A. calcoaceticus from EC-exposed biofilms to CIP, LEV and TMP-SMX. Regarding the impact of the non-antibiotic ECs (CAF, CBZ and IBP) tested, it is possible to point out some changes in A. calcoaceticus tolerance to specific antibiotics. For instance, the exposure to CBZ resulted in a slight but statistically significant (P < 0.05) increase in A. calcoaceticus susceptibility to TMP-SMX. Other works have referred that the exposure to CBZ at 50
000 ng L−1 has enhanced the conjugative transfer of multi-resistance genes within and across bacterial genera, increased cell membrane permeability, pilus generation, and expression of genes related to these processes were significantly upregulated.74 However, in the present work, the decrease in the tolerance to TMP-SMX after biofilm exposure to CBZ could not be correlated with such observations. In the case of exposure to the contaminant IBP, it seems to cause a slight increase in the tolerance to LEV and TET, reducing the inhibition halo by approximately 4 and 6%, respectively. Moreover, a recent study has demonstrated that non-antibiotic pharmaceuticals, including ibuprofen at clinically and environmentally relevant concentrations (0.005, 0.01, 0.05, 0.1, 0.5, 1.0, 5.0 and 50.0 mg L−1) can promote the spread of antibiotic resistance through conjugation.75
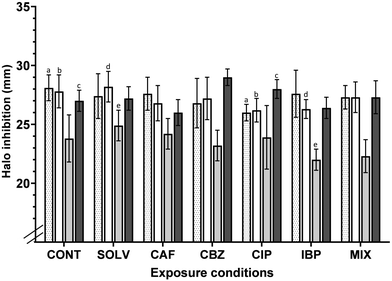 |
| Fig. 4 Effect of the exposure to ECs on A. calcoaceticus susceptibility to CIP ( ), LEV (□), TET ( ) and TMP-SMX ( ), represented by the inhibition halo diameter (mm). The values reported are the means ± standard deviation of six independent assays, resulting from two independent exposure assays. CONT – control, Solv – DMSO solvent control, CAF – caffeine, CBZ – carbamazepine, CIP – ciprofloxacin, IBP – ibuprofen, LEV – levofloxacin, MIX – mixture of ECs, TET -tetracycline, TMP-SMX – trimethoprim – sulfamethoxazole, a–e – corresponds to conditions that have statistically significant differences from each other (P < 0.05). | |
The impact of the exposure of bacteria to sub-inhibitory concentrations on the acquisition of determinants of antimicrobial resistance has been extensively described in the literature.76,77 Bacteria will become tolerant to antibiotics when reduced concentrations are used. In the present study, the exposure to the fluoroquinolone CIP at environmentally relevant concentrations increased the A. calcoaceticus tolerance to some antibiotics, in particular to the fluoroquinolones LEV and CIP (P < 0.05), reducing the halo diameter by around 5% in comparison to the control counterparts. Other works, demonstrated that clinical isolates resistant to LEV are usually also resistant to CIP,78 highlighting the known cross-resistance among quinolones79 in clinical environments. Murray et al.80 described CIP as the antibiotic that may pose the highest selection risk in the environment. The authors found that the minimal selective concentration (i.e. the lowest concentration of CIP at which resistance is predicted to be selected) of CIP was between 0.004 and 10.77 μg L−1.80 So, the concentration (679.7 ng L−1; representative to those found in DW) tested is found in the range of CIP minimal selective concentration described by Murray et al.80 However, the results demonstrated an increase in the susceptibility to TMP-SMX after exposure to CIP (P < 0.05). These results propose that individual exposure to pharmaceuticals, non-antibiotics and antibiotics, influenced A. calcoaceticus susceptibility to antibiotics.
Several mechanisms may be involved in antibiotic resistance, such as (1) production of enzymes that inactivate the antibiotic molecule, (2) mutation in the site where the antibiotic acts, (3) reduction of absorption, (4) efflux mechanisms that remove the antibiotic from the interior of the cells, (5) excessive production of the antibiotic target compounds, or (6) creation of an alternative metabolic pathway (that performs a similar function to that inactivated by the antibiotic or by limiting cellular requirements for the metabolites produced in this inhibited pathway).38,81,82 However, tolerance is a concept that differs from resistance. Resistance is the genetic ability of bacteria to encode genes that counteract the inhibitory effect of antimicrobial agents.83 Tolerance refers to the ability (of genetic origin or not) of a microorganism to survive when exposed to high concentrations of an antimicrobial agent.84 Therefore, to confirm ECs induced changes in the genomic structure that lead to the development of A. calcoaceticus tolerance to the antibiotics tested, a further genetic or molecular analysis should be conducted. The results demonstrated that regardless the exposure conditions, A. calcoaceticus remained susceptible to all the antibiotics tested. However, in some cases, their susceptibility was reduced or increased, which may result from an adaptation process to the presence of stressing molecules under conditions with low availability of nutrients. Bacterial exposure to stress may trigger metabolic and physiological mechanisms to overcome unfavourable conditions.85 This adaptation may confer bacteria with different strategies to survive under these unfavourable conditions, specifically through a rapid signalling response (i.e. quorum-sensing), which allows a rapid adaptation to harsh conditions.85 Furthermore, the provenance of A. calcoaceticus from biofilms may also affect the phenotypical response to antibiotics, since it is known that the biofilm cell density and/or their physiological state play a role in the phenotypic-based drug resistance.86,87
3.4. Effects of ECs exposure on A. calcoaceticus ability to form a biofilm
Biofilm formation, an intrinsic and adaptive mechanism of resistance, is essential for the survival of bacteria facing adverse environmental stimuli.88 Given the presence of a multitude of contaminants in DW and issues related to biofilm formation, an increase in A. calcoaceticus ability to form biofilms due to ECs exposure may be detrimental to DW quality. On the contrary, a decrease in biofilm formation is favourable to DWDS and reduces the risk for the distributed water. A. calcoaceticus from EC-exposed biofilms were characterized in terms of biofilm formation ability through crystal violet staining. Fig. 5 presents the results obtained.
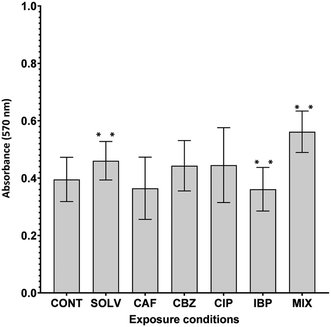 |
| Fig. 5 Biofilm formation (24 h) by A. calcoaceticus from ECs-exposed biofilms. Results were obtained from absorbance measurements at 570 nm and are presented as the average of three independent assays for each of the two independent exposure assays performed. CONT – non-exposed A. calcoaceticus, Solv – DMSO solvent control, CAF – caffeine, CBZ – carbamazepine, CIP – ciprofloxacin, IBP – ibuprofen, MIX – ECs mixture, ** corresponds to results with statistically significant differences in comparison to Solv (P < 0.05)). | |
The exposure of A. calcoaceticus to solvent (Solv) DMSO, increased biofilm formation capacity (P < 0.05) in comparison to non-exposed A. calcoaceticus. Therefore, the effect of ECs prepared with DSMO (CBZ, IBP and MIX) was evaluated by comparing with the Solv. Statistically significant changes (P < 0.05) were detected after IBP and MIX exposure. IBP exposure for 7 days induced a decrease of approximately 11% in the A. calcoaceticus ability to form biofilm. Alternatively, Gomes et al.30 determined that Stenotrophomonas maltophilia exposure to IBP (3 ng L−1) had no remarkable effect on its ability to form biofilms at environmentally relevant concentrations. However, remarkable effects were found for concentrations 100 times higher than that of DWDS (300 ng L−1), a value closer to the tested in the present work (IBP at 223.6 ng L−1).30 Moreover, Reśliński et al.89 also demonstrated that the presence of IBP at 20 mg L−1 reduced the cellular density of biofilms formed by E. coli and S. aureus. Furthermore, in this study, MIX exposure increased biofilm formation by 16%. Therefore, the simultaneous presence of the selected ECs caused an increase in biofilm formation, which may constitute a critical scenario since the bacteria exposed may have a higher propensity to colonize other parts of DWDS surfaces.
3.5. Effect of ECs exposure on A. calcoaceticus motility
Microbial adhesion to DWDS surfaces is indispensable for biofilm formation. This phenomenon depends on physical, chemical and biological interactions between bacteria and DWDS pipe surfaces, where motility takes part in the early stages of biofilm formation,29 including the adhesion process.48 Bacterial motility corresponds to their ability to move on a liquid medium or solid surfaces, whose mechanisms vary among species, being adjusted for their respective habitats.90 Cells can change their modes of motility when stimulated or faced with unfavourable conditions (e.g., nutrients or pollutants), to move to optimal environments for their growth and proliferation.91–93 Furthermore, motility is not only related to biofilm formation, but may also be a crucial attribute to the virulence of pathogenic bacteria,90 since motility also facilitates the interaction of bacteria with host cells.
A. calcoaceticus expresses swimming, swarming and twitching types of motility.46,94–96 Therefore, the impact of ECs exposure on the motility of A. calcoaceticus from exposed biofilms was evaluated and the results are presented in Fig. 6.
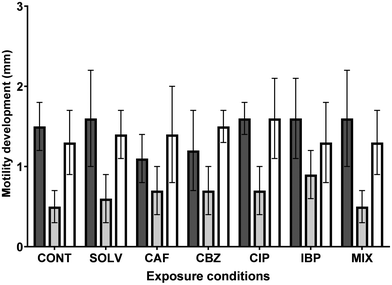 |
| Fig. 6 Motility (swarming – ■, swimming – ■ and twitching – □) of A. calcoaceticus from ECs-exposed biofilms (exposure to ECs for 7 days) is expressed as the diameter of colony growth in different media. The values reported are the means ± standard deviation of three independent assays, for each one of the two independent exposure assays performed. CONT – control, Solv – DMSO solvent control, CAF – caffeine, CBZ – carbamazepine, CIP – ciprofloxacin, , IBP – ibuprofen, MIX – mixture of ECs. | |
Despite slight changes in motility expression observed in bacteria from EC-exposed biofilms, no remarkable alterations (P > 0.05) were detected. Consequently, the increase in biofilm formation does not correlate with the changes caused in bacterial motility.
4. Conclusions
The present work provides pioneer insights on the changes in A. calcoaceticus behaviour after biofilm exposure to selected ECs. The selected ECs are potential threats to DW quality and to DWDS management. Even though in a few particular cases, ECs exposure could prove to be beneficial for biofilm management in DWDS, based on the decrease in biofilm formation caused by IBP and the reduction in the tolerance to NaOCl caused by CBZ exposure. However, ECs exposure could also be responsible for DW quality control failures (by promoting biofilm regrowth within DWDS walls and water phase, as well as favour antimicrobial tolerance). In particular, IBP and CIP are the ECs that caused the most significant alterations in A. calcoaceticus behaviour, specifically their impact could be of concern regarding A. calcoaceticus susceptibility to some antibiotics and/or NaOCl. Moreover, this work highlights that advanced monitoring of ECs occurrence in aquatic environments, assessment of their fate and ecotoxicological risks, and implementation of pollution control policies, including emission control (point-source) and advanced water treatments, are necessary to prevent undesired consequences from the presence of such contaminants.
Conflicts of interest
There are no conflicts of interest to declare.
Acknowledgements
This work was financially supported by: LA/P/0045/2020 (ALiCE) and UIDP/00511/2020 (LEPABE), funded by national funds through FCT/MCTES (PIDDAC); Projects Biocide_for_Biofilm—PTDC/BII-BTI/30219/2017—POCI-01-0145-FEDER-030219; Germirrad—POCI-01-0247-FEDER-072237 funded by FEDER funds through COMPETE2020 – Programa Operacional Competitividade e Internacionalização (POCI) and by national funds (PIDDAC) through FCT/MCTES; Project “HealthyWaters – Identification, Elimination, Social Awareness and Education of Water Chemical and Biological Micropollutants with Health and Environmental Implications”, with reference NORTE-01-0145-FEDER-000069, supported by Norte Portugal Regional Operational Programme (NORTE 2020), under the PORTUGAL 2020 Partnership Agreement, through the European Regional Development Fund (ERDF).
References
- Y. Luo, W. Guo, H. H. Ngo, L. D. Nghiem, F. I. Hai, J. Zhang, S. Liang and X. C. Wang, A review on the occurrence of micropollutants in the aquatic environment and their fate and removal during wastewater treatment, Sci. Total Environ., 2014, 473, 619–641 CrossRef.
-
N. M. Kumar, M. C. Sudha, T. Damodharam and S. Varjani, in Cur. Devel. Biotechnol. Bioeng., Elsevier, 2020, pp. 41–62 Search PubMed.
- S. T. Glassmeyer, E. T. Furlong, D. W. Kolpin, A. L. Batt, R. Benson, J. S. Boone, O. Conerly, M. J. Donohue, D. N. King and M. S. Kostich, Nationwide reconnaissance of contaminants of emerging concern in source and treated drinking waters of the United States, Sci. Total Environ., 2017, 581, 909–922 CrossRef.
- B. Petrie, R. Barden and B. Kasprzyk-Hordern, A review on emerging contaminants in wastewaters and the environment: current knowledge, understudied areas and recommendations for future monitoring, Water Res., 2015, 72, 3–27 CrossRef CAS.
- C. Peña-Guzmán, S. Ulloa-Sánchez, K. Mora, R. Helena-Bustos, E. Lopez-Barrera, J. Alvarez and M. Rodriguez-Pinzón, Emerging pollutants in the urban water cycle in Latin America: A review of the current literature, J. Environ. Manage., 2019, 237, 408–423 CrossRef.
- C. F. Couto, L. C. Lange and M. C. Amaral, Occurrence, fate and removal of pharmaceutically active compounds (PhACs) in water and wastewater treatment plants—A review, J. Water Process. Eng., 2019, 32, 100927 CrossRef.
- N. Corcoll, V. Acuña, D. Barceló, M. Casellas, H. Guasch, B. Huerta, M. Petrovic, L. Ponsatí, S. Rodríguez-Mozaz and S. Sabater, Pollution-induced community tolerance to non-steroidal anti-inflammatory drugs (NSAIDs) in fluvial biofilm communities affected by WWTP effluents, Chemosphere, 2014, 112, 185–193 CrossRef CAS PubMed.
- M. Petrović, M. D. Hernando, M. S. Díaz-Cruz and D. Barceló, Liquid chromatography–tandem mass spectrometry for the analysis of pharmaceutical residues in environmental samples: a review, J. Chromatogr. A, 2005, 1067, 1–14 CrossRef.
- P. Houeto, A. Carton, M. Guerbet, A.-C. Mauclaire, C. Gatignol, P. Lechat and D. Masset, Assessment of the health risks related to the presence of drug residues in water for human consumption: Application to carbamazepine, Regul. Toxicol. Pharmacol., 2012, 62, 41–48 CrossRef CAS.
- P. E. Stackelberg, E. T. Furlong, M. T. Meyer, S. D. Zaugg, A. K. Henderson and D. B. Reissman, Persistence of pharmaceutical compounds and other organic wastewater contaminants in a conventional drinking-water-treatment plant, Sci. Total Environ., 2004, 329, 99–113 CrossRef CAS PubMed.
- J. L. Wilkinson, P. S. Hooda, J. Barker, S. Barton and J. Swinden, Ecotoxic pharmaceuticals, personal care products, and other emerging contaminants: A review of environmental, receptor-mediated, developmental, and epigenetic toxicity with discussion of proposed toxicity to humans, Crit. Rev. Environ. Sci. Technol., 2016, 46, 336–381 CrossRef CAS.
- C. Afonso-Olivares, M. E. Torres-Padrón, Z. Sosa-Ferrera and J. J. Santana-Rodríguez, Assessment of the presence of pharmaceutical compounds in seawater samples from coastal area of Gran Canaria Island (Spain), Antibiotics, 2013, 2, 274–287 CrossRef CAS.
- A. Kot-Wasik, A. Jakimska and M. Śliwka-Kaszyńska, Occurrence and seasonal variations of 25 pharmaceutical residues in wastewater and drinking water treatment plants, Environ. Monit. Assess., 2016, 188, 661 CrossRef CAS.
-
WHO, Pharmaceuticals in drinking water Public health and environment, water, sanitation, hygiene and health, WHO Geneva, 2011 Search PubMed.
- S. Ceschin, A. Bellini and M. Scalici, Aquatic plants and ecotoxicological assessment in freshwater ecosystems: a review, Environ. Sci. Pollut. Res., 2021, 28, 4975–4988 CrossRef CAS PubMed.
- L. Prosnier, M. Loreau and F. D. Hulot, Modeling the direct and indirect effects of copper on phytoplankton–zooplankton interactions, Aquat. Toxicol., 2015, 162, 73–81 CrossRef CAS PubMed.
- K. A. Kidd, P. J. Blanchfield, K. H. Mills, V. P. Palace, R. E. Evans, J. M. Lazorchak and R. W. Flick, Collapse of a fish population after exposure to a synthetic estrogen, Proc. Natl. Acad. Sci. U. S. A., 2007, 104, 8897–8901 CrossRef CAS.
- J. Weinberger and R. Klaper, Environmental concentrations of the selective serotonin reuptake inhibitor fluoxetine impact specific behaviors involved in reproduction, feeding and predator avoidance in the fish Pimephales promelas (fathead minnow), Aquat. Toxicol., 2014, 151, 77–83 CrossRef CAS.
-
P. E. Rosenfeld and L. G. H. Feng, in Risks Hazard. Wastes, ed. P. E. Rosenfeld and L. G. H. Feng, William Andrew Publishing, Boston, 2011, pp. 215–222, DOI:10.1016/B978-1-4377-7842-7.00016-7.
- J. Subirats, X. Timoner, A. Sànchez-Melsió, J. L. Balcázar, V. Acuña, S. Sabater and C. M. Borrego, Emerging contaminants and nutrients synergistically affect the spread of class 1 integron-integrase (intI1) and sul1 genes within stable streambed bacterial communities, Water Res., 2018, 138, 77–85 CrossRef CAS.
- N. Corcoll, M. Casellas, B. Huerta, H. Guasch, V. Acuña, S. Rodríguez-Mozaz, A. Serra-Compte, D. Barceló and S. Sabater, Effects of flow intermittency and pharmaceutical exposure on the structure and metabolism of stream biofilms, Sci. Total Environ., 2015, 503, 159–170 CrossRef.
- E. J. Rosi-Marshall, D. W. Kincaid, H. A. Bechtold, T. V. Royer, M. Rojas and J. J. Kelly, Pharmaceuticals suppress algal growth and microbial respiration and alter bacterial communities in stream biofilms, Ecol. Appl., 2013, 23, 583–593 CrossRef PubMed.
- L. Proia, V. Osorio, S. Soley, M. Köck-Schulmeyer, S. Pérez, D. Barceló, A. M. Romaní and S. Sabater, Effects of pesticides and pharmaceuticals on biofilms in a highly impacted river, Environ. Pollut., 2013, 178, 220–228 CrossRef CAS PubMed.
- L. C. Simões and M. Simões, Biofilms in drinking water: problems and solutions, RSC Adv., 2013, 3, 2520–2533 RSC.
-
J. Block, in Biofilms— Science and Technology, Springer, 1992, pp. 469–485 Search PubMed.
-
E. R. Martinez, PhD PhD dissertation, Universitat Politècnica de València, 2016 Search PubMed.
-
S. Aggarwal and C. K. Gomez-Smith, in Encyclopedia of Water: Science, Technology, and Society, ed. P. Maurice, Wiley, 2019, ch. 178, pp. 1–17, DOI:10.1002/9781119300762.wsts0193.
- N. M. Hull, F. Ling, A. J. Pinto, M. Albertsen, H. G. Jang, P. Y. Hong, K. T. Konstantinidis, M. LeChevallier, R. R. Colwell and W. T. Liu, Drinking water microbiome project: is it time?, Trends Microbiol., 2019, 27, 670–677 CrossRef CAS PubMed.
- I. Gomes, D. Madureira, L. C. Simões and M. Simões, The effects of pharmaceutical and personal care products on the behavior of Burkholderia cepacia isolated from drinking water, Int. Biodeterior. Biodegrad., 2019, 141, 87–93 CrossRef CAS.
- I. B. Gomes, L. C. Simões and M. Simões, The effects of emerging environmental contaminants on Stenotrophomonas maltophilia isolated from drinking water in planktonic and sessile states, Sci. Total Environ., 2018, 643, 1348–1356 CrossRef CAS.
- H. Wang, C. Hu, Y. Shen, B. Shi, D. Zhao and X. Xing, Response of microorganisms in biofilm to sulfadiazine and ciprofloxacin in drinking water distribution systems, Chemosphere, 2019, 218, 197–204 CrossRef CAS PubMed.
- I. Gomes, M. M. Querido, J. P. Teixeira, C. C. Pereira, L. C. Simões and M. Simões, Prolonged exposure of Stenotrophomonas maltophilia biofilms to trace levels of clofibric acid alters antimicrobial tolerance and virulence, Chemosphere, 2019, 235, 327–335 CrossRef CAS.
- Y. Zhang, Y. Zhang, L. Liu, L. Zhou and Z. Zhao, Impacts of antibiotics on biofilm bacterial community and disinfection performance on simulated drinking water supply pipe wall, Environ. Pollut., 2021, 288, 117736 CrossRef CAS.
- H.-C. Flemming, S. Percival and J. Walker, Contamination potential of biofilms in water distribution systems, Water Sci. Technol.: Water Supply, 2002, 2, 271–280 CAS.
- W. Lin, Z. Yu, X. Chen, R. Liu and H. Zhang, Molecular characterization of natural biofilms from household taps with different materials: PVC, stainless steel, and cast iron in drinking water distribution system, Appl. Microbiol. Biotechnol., 2013, 97, 8393–8401 CrossRef CAS.
- P. E. Stackelberg, J. Gibs, E. T. Furlong, M. T. Meyer, S. D. Zaugg and R. L. Lippincott, Efficiency of conventional drinking-water-treatment processes in removal of pharmaceuticals and other organic compounds, Sci. Total Environ., 2007, 377, 255–272 CrossRef CAS.
-
R. Tauber, Quantitative analysis of pharmaceuticals in drinking water from ten Canadian cities, Enviro-Test Laboratories/Xenos Division Ontario, 2003 Search PubMed.
- M. Pazda, J. Kumirska, P. Stepnowski and E. Mulkiewicz, Antibiotic resistance genes identified in wastewater treatment plant systems–A review, Sci. Total Environ., 2019, 697, 134023 CrossRef CAS PubMed.
- F. Baquero, J.-L. Martínez and R. Cantón, Antibiotics and antibiotic resistance in water environments, Curr. Opin. Biotechnol., 2008, 19, 260–265 CrossRef CAS.
- J. García, M. J. García-Galán, J. W. Day, R. Boopathy, J. R. White, S. Wallace and R. G. Hunter, A review of emerging organic contaminants (EOCs), antibiotic resistant bacteria (ARB), and antibiotic resistance genes (ARGs) in the environment: Increasing removal with wetlands and reducing environmental impacts, Bioresour. Technol., 2020, 123228 CrossRef PubMed.
- Y. Zhang, A. Z. Gu, M. He, D. Li and J. Chen, Subinhibitory concentrations of disinfectants promote the horizontal transfer of multidrug resistance genes within and across genera, Environ. Sci. Technol., 2017, 51, 570–580 CrossRef CAS PubMed.
- C. Ching, E. S. F. Orubu, I. Sutradhar, V. J. Wirtz, H. W. Boucher and M. H. Zaman, Bacterial antibiotic resistance development and mutagenesis following exposure to subinhibitory concentrations of fluoroquinolones in vitro: a systematic review of the literature, Antimicrob. Resist., 2020, 2(3), dlaa068 CrossRef PubMed.
- Y. Wang, J. Lu, L. Mao, J. Li, Z. Yuan, P. L. Bond and J. Guo, Antiepileptic drug carbamazepine promotes horizontal transfer of plasmid-borne multi-antibiotic resistance genes within and across bacterial genera, ISME J., 2019, 13, 509–522 CrossRef CAS.
- J. Lu, Y. Wang, J. Li, L. Mao, S. H. Nguyen, T. Duarte, L. Coin, P. Bond, Z. Yuan and J. Guo, Triclosan at environmentally relevant concentrations promotes horizontal transfer of multidrug resistance genes within and across bacterial genera, Environ. Int., 2018, 121, 1217–1226 CrossRef CAS PubMed.
- I. B. Gomes, J.-Y. Maillard, L. C. Simões and M. Simões, Emerging contaminants affect the microbiome of water systems—strategies for their mitigation, npj Clean Water, 2020, 3, 39 CrossRef CAS.
- L. C. Simões, M. Simões and M. J. Vieira, Biofilm interactions between distinct bacterial genera isolated from drinking water, Appl. Environ. Microbiol., 2007, 73, 6192–6200 CrossRef.
- E. I. Prest, F. Hammes, M. van Loosdrecht and J. S. Vrouwenvelder, Biological stability of drinking water: controlling factors, methods, and challenges, Front. Microbiol., 2016, 7, 45 Search PubMed.
- I. Gomes, M. Simões and L. C. Simões, The effects of sodium hypochlorite against selected drinking water-isolated bacteria in planktonic and sessile states, Sci. Total Environ., 2016, 565, 40–48 CrossRef CAS PubMed.
- I. B. Gomes, M. Lemos, L. Mathieu, M. Simões and L. C. Simões, The action of chemical and mechanical stresses on single and dual species biofilm removal of drinking water bacteria, Sci. Total Environ., 2018, 631-632, 987–993 CrossRef CAS.
- B. G. Hall, H. Acar, A. Nandipati and M. Barlow, Growth rates made easy, Mol. Biol. Evol., 2013, 31, 232–238 CrossRef.
-
J. B. Patel, F. Cockerill and P. A. Bradford, Performance standards for antimicrobial susceptibility testing: twenty-fifth informational supplement, 2015 Search PubMed.
- S. Stepanović, D. Vuković, I. Dakić, B. Savić and M. Švabić-Vlahović, A modified microtiter-plate test for quantification of staphylococcal biofilm formation, J. Microbiol. Methods, 2000, 40, 175–179 CrossRef.
- M. T. Butler, Q. Wang and R. M. Harshey, Cell density and mobility protect swarming bacteria against antibiotics, Proc. Natl. Acad. Sci. U. S. A., 2010, 107, 3776–3781 CrossRef CAS.
- H. G. Stickland, P. W. Davenport, K. S. Lilley, J. L. Griffin and M. Welch, Mutation of nfxB causes global changes in the physiology and metabolism of Pseudomonas aeruginosa, J. Proteome Res., 2010, 9, 2957–2967 CrossRef CAS.
- R. Boe-Hansen, H.-J. Albrechtsen, E. Arvin and C. Jørgensen, Bulk water phase and biofilm growth in drinking water at low nutrient conditions, Water Res., 2002, 36, 4477–4486 CrossRef CAS.
- E. Atolia, S. Cesar, H. A. Arjes, M. Rajendram, H. Shi, B. D. Knapp, S. Khare, A. Aranda-Díaz, R. E. Lenski, K. C. Huang and K. T. Hughes, Environmental and physiological factors affecting high-throughput measurements of bacterial growth, MBio, 2020, 11, e01320–e01378 CrossRef.
- Y.-S. Tan, R.-K. Zhang, Z.-H. Liu, B.-Z. Li and Y.-J. Yuan, Microbial adaptation to enhance stress tolerance, Front. Microbiol., 2022, 13, 888746 CrossRef.
- R. A. E. Shaker, Y. I. Nagy, M. E. Adly, R. A. Khattab and Y. M. Ragab,
Acinetobacter baumannii, Klebsiella pneumoniae and Elizabethkingia miricola isolated from wastewater have biodegradable activity against fluoroquinolone, World J. Microbiol. Biotechnol., 2022, 38, 187 CrossRef CAS.
- S. Kim, S. N. Lindner, S. Aslan, O. Yishai, S. Wenk, K. Schann and A. Bar-Even, Growth of E. coli on formate and methanol via the reductive glycine pathway, Nat. Chem. Biol., 2020, 16, 538–545 CrossRef CAS.
- T. Tuan-Anh, H. T. Tuyen, N. N. Minh Chau, N. D. Toan, T. H. Triet, L. M. Triet, N. H. T. Trang, N. T. N. To, J. Bartholdson Scott, H. C. The, D. P. Thanh, H. Clapham and S. Baker, Pathogenic Escherichia coli possess elevated growth rates under exposure to sub-inhibitory concentrations of azithromycin, Antibiotics, 2020, 9, 735 CrossRef CAS.
- Z. Tsvetanova, Quantification of the bacterial community of drinking water-associated biofilms under different flow velocities and changing chlorination regimes, Appl. Water Sci., 2020, 10, 3 CrossRef CAS.
- S. Khan, T. K. Beattie and C. W. Knapp, Relationship between antibiotic-and disinfectant-resistance profiles in bacteria harvested from tap water, Chemosphere, 2016, 152, 132–141 CrossRef CAS.
- C. Bertelli, S. Courtois, M. Rosikiewicz, P. Piriou, S. Aeby, S. Robert, J.-F. Loret and G. Greub, Reduced chlorine in drinking water distribution systems impacts bacterial biodiversity in biofilms, Front. Microbiol., 2018, 9, 2520 CrossRef PubMed.
- J. Chen, W. Li, J. Zhang, W. Qi, Y. Li, S. Chen and W. Zhou, Prevalence of antibiotic resistance genes in drinking water and biofilms: The correlation with the microbial community and
opportunistic pathogens, Chemosphere, 2020, 259, 127483 CrossRef CAS PubMed.
-
WHO, Guidelines for drinking-water quality, 2011 Search PubMed.
-
CDC, Chlorine residual testing fact sheet, CDC SWS Project, 2014, https://www.cdc.gov/safewater/publications_pages/chlorineresidual.pdf Search PubMed.
-
EPA, National primary drinking water regulations, US Environmental Protection Agency, 2009 Search PubMed.
- X.-F. Li and W. A. Mitch, Drinking water disinfection byproducts (DBPs) and human health effects: multidisciplinary challenges and opportunities, Environ. Sci. Technol., 2018, 52, 1681–1689 CrossRef CAS.
- I. Alderton, B. R. Palmer, J. A. Heinemann, I. Pattis, L. Weaver, M. J. Gutiérrez-Ginés, J. Horswell and L. A. Tremblay, The role of emerging organic contaminants in the development of antimicrobial resistance, Emerging Contam., 2021, 7, 160–171 CrossRef CAS.
- Y. Shen, R. D. Stedtfeld, X. Guo, G. D. Bhalsod, S. Jeon, J. M. Tiedje, H. Li and W. Zhang, Pharmaceutical exposure changed antibiotic resistance genes and bacterial communities in soil-surface- and overhead-irrigated greenhouse lettuce, Environ. Int., 2019, 131, 105031 CrossRef CAS PubMed.
-
Y. Motarjemi, G. Moy and E. Todd, Encyclopedia of food safety, Academic Press, 2013 Search PubMed.
- E. Sanganyado and W. Gwenzi, Antibiotic resistance in drinking water systems: Occurrence, removal, and human health risks, Sci. Total Environ., 2019, 669, 785–797 CrossRef CAS PubMed.
-
CLSI, Performance standards for antimicrobial susceptibility testing: twenty-fifth informational supplement, 2015 Search PubMed.
- Y. Wang, J. Lu, L. Mao, J. Li, Z. Yuan, P. L. Bond and J. Guo, Antiepileptic drug carbamazepine promotes horizontal transfer of plasmid-borne multi-antibiotic resistance genes within and across bacterial genera, ISME J., 2019, 13, 509–522 CrossRef CAS PubMed.
- Y. Wang, J. Lu, S. Zhang, J. Li, L. Mao, Z. Yuan, P. L. Bond and J. Guo, Non-antibiotic pharmaceuticals promote the transmission of multidrug resistance plasmids through intra- and intergenera conjugation, ISME J., 2021, 15, 2493–2508 CrossRef CAS PubMed.
- G. G. Zhanel, D. J. Hoban and G. K. Harding, Subinhibitory antimicrobial concentrations: A review of in vitro and in vivo data, Can. J. Infect. Dis., 1992, 3, 193–201 CAS.
- C. Ching, E. S. F. Orubu, V. J. Wirtz and M. H. Zaman, Bacterial antibiotic resistance development and mutagenesis following exposure to subminimal inhibitory concentrations of fluoroquinolones in vitro: a systematic literature review protocol, BMJ Open, 2019, 9, e030747 CrossRef PubMed.
- T. A. Davies, R. Goldschmidt, S. Pfleger, M. Loeloff, K. Bush, D. F. Sahm and A. Evangelista, Cross-resistance, relatedness and allele analysis of fluoroquinolone-resistant US clinical isolates of Streptococcus pneumoniae (1998-2000), J. Antimicrob. Chemother., 2003, 52, 168–175 CrossRef CAS PubMed.
- C. C. Sanders, Mechanisms responsible for cross-resistance and dichotomous resistance among the quinolones, Clin. Infect. Dis., 2001, 32(Suppl 1), S1–S8 CrossRef CAS PubMed.
- A. K. Murray, I. Stanton, W. H. Gaze and J. Snape, Dawning of a new ERA: Environmental Risk Assessment of antibiotics and their potential to select for antimicrobial resistance, Water Res., 2021, 200, 117233 CrossRef CAS.
- T. Y. Tan, Use of molecular techniques for the detection of antibiotic resistance in bacteria, Expert Rev. Mol. Diagn., 2003, 3, 93–103 CrossRef CAS.
- E. Marti, E. Variatza and J. L. Balcazar, The role of aquatic ecosystems as reservoirs of antibiotic resistance, Trends Microbiol., 2014, 22, 36–41 CrossRef CAS.
- Z. A. Khan, M. F. Siddiqui and S. Park, Current and emerging methods of antibiotic susceptibility testing, Diagnostics, 2019, 9, 49 CrossRef CAS.
- I. Levin-Reisman, I. Ronin, O. Gefen, I. Braniss, N. Shoresh and N. Q. Balaban, Antibiotic tolerance facilitates the evolution of resistance, Science, 2017, 355, 826–830 CrossRef CAS PubMed.
- M. Marmion, G. Macori, M. Ferone, P. Whyte and A. G. M. Scannell, Survive and thrive: Control mechanisms that facilitate bacterial adaptation to survive manufacturing-related stress, Int. J. Food Microbiol., 2022, 368, 109612 CrossRef CAS PubMed.
- P. Perumal, S. Mekala and W. L. Chaffin, Role for cell density in antifungal drug resistance in Candida albicans biofilms, Antimicrob. Agents Chemother., 2007, 51, 2454–2463 CrossRef CAS.
- A. E. Kirby, K. Garner and B. R. Levin, The relative contributions of physical structure and cell density to the antibiotic susceptibility of bacteria in biofilms, Antimicrob. Agents Chemother., 2012, 56, 2967–2975 CrossRef CAS.
- E. K. Chu, O. Kilic, H. Cho, A. Groisman and A. Levchenko, Self-induced mechanical stress can trigger biofilm formation in uropathogenic Escherichia coli, Nat. Commun., 2018, 9, 1–10 CrossRef CAS.
- A. Reśliński, S. Dąbrowiecki and K. Głowacka, The impact of diclofenac and ibuprofen on biofilm formation on the surface of polypropylene mesh, Hernia, 2015, 19, 179–185 CrossRef.
- S. Nakamura and T. Minamino, Flagella-driven motility of bacteria, Biomolecules, 2019, 9, 279 CrossRef CAS.
- C. Jimenez-Sanchez, L. Y. Wick and J.-J. Ortega-Calvo, Chemical effectors cause different motile behavior and deposition of bacteria in porous media, Environ. Sci. Technol., 2012, 46, 6790–6797 CrossRef CAS.
- G. L. Mino, M. Koehl, N. King and R. Stocker, Finding patches in a heterogeneous aquatic environment: pH-taxis by the dispersal stage of choanoflagellates, Limnol. Oceanogr. Lett., 2017, 2, 37–46 CrossRef.
- G. H. Wadhams and J. P. Armitage, Making sense of it all: bacterial chemotaxis, Nat. Rev. Mol. Cell Biol., 2004, 5, 1024–1037 CrossRef CAS PubMed.
- J. Henrichsen, Not gliding but twitching motility of Acinetobacter calcoaceticus, J. Clin. Pathol., 1984, 37, 102 CrossRef CAS PubMed.
- J. Hentuchsen and J. Blom, Correlation between twitching motility and possession of polar fimbriae in Acinetobacter calcoaceticus, Acta Pathol. Microbiol. Scand., Sect. B: Microbiol., 1975, 83, 103–115 Search PubMed.
-
I. Biswas, A. Machen and J. Mettlach, in Acinetobacter baumannii, Springer, 2019, pp. 177–187 Search PubMed.
- Q.-J. Wang, C.-H. Mo, Y.-W. Li, P. Gao, Y.-P. Tai, Y. Zhang, Z.-L. Ruan and J.-W. Xu, Determination of four fluoroquinolone antibiotics in tap water in Guangzhou and Macao, Environ. Pollut., 2010, 158, 2350–2358 CrossRef.
|
This journal is © The Royal Society of Chemistry 2023 |
Click here to see how this site uses Cookies. View our privacy policy here.