Enhanced electrocoagulation process for natural organic matter removal from surface drinking water sources: coagulant dose control & organic matter characteristics†
Received
21st May 2022
, Accepted 24th October 2022
First published on 8th November 2022
Abstract
In this study, an enhanced electro-coagulation (En-EC) technique is described for removal of natural dissolved organic matter (DOM) from surface drinking water sources. Assessment of the En-EC technique included investigating the impacts of operational factors on DOM removal efficiency, DOM characteristic variations, and energy consumption (CE). DOM removal efficiencies by En-EC were compared with removals by enhanced chemical coagulation (EnC) along with residual metal levels. The removal of DOM was assessed by tracking the % reduction (R%) of dissolved organic carbon (DOC) and its surrogate parameters (i.e. UV absorbance at 254 nm (A254), colour, fluorophore DOM fluorescence signal (fDOMs)) following the En-EC process. Experiments were conducted using samples collected from three surface drinking water sources, i.e., Murray River, Myponga River and Middle River located in South Australia, Australia. An electrocoagulation cell was assembled and equipped with capability for measuring consumed CE and electrical charge (Q). The impacts of process parameters (i.e. IEC, the process pH [pHEC], and the aluminium coagulant dose [AlEC]) on the R% and CE were also studied. The DOM characteristic variations following the process were studied using UV-vis spectroscopy, fluorescence excitation–emission (ex/em) (matrix) spectroscopy and high performance size exclusion chromatography equipped with UV and fluorescence ex/em spectrophotometer detectors. An optimum pHEC of 6 was obtained experimentally for the En-Ec process. A comparable DOM removal efficiency was observed for either AlEC in En-EC or alum in EnC. A maximum % A254 removal of 74% to 92% was observed for the studied water sources, depending on the DOM characteristics. A comparable DOM removal performance was observed for En-EC and EnC. This was a basis for adopting a previously developed decision support system for alum dosing in the EnC process, for AlEC dosing control in the En-EC process.
Water impact
Given the benefits of renewable energy, the development of eco/user-friendly technologies is receiving greater attention. An electro-coagulation process was investigated for treatment of drinking waters of highly contrasting water qualities. This study aimed to improve the understanding of the EC process for drinking water treatment in the context of maximizing the removal of DOM and understanding the characteristic dynamics of DOM with EC treatment.
|
1. Introduction
In Australia and globally, drinking water is commonly supplied through treating surface waters sourced from rivers and reservoirs.1,2 The removal of DOM (i.e., dissolved fraction of natural organic matter [NOM]) from drinking water sources is likely to become more challenging for the drinking water industry as concentrations in waters increase with climate change and extreme weather impacts on water quality.3 It is well established that conventional drinking water treatment comprising coagulation, flocculation and settling, followed by filtration and disinfection processes, more efficiently removes hydrophobic and high molecular weight (HMW) compounds than hydrophilic and low molecular weight (LMW) compounds.4,5 The residual NOM after coagulation generally retains significant disinfection by-product formation potential (DBPFP).
Enhanced coagulation (EnC) refers to achieving a prescribed removal of total organic carbon dependent upon the source water alkalinity through applying a sufficient coagulant dose and potentially, with pH control.6,7 EnC is the recommended technology by the US Environmental Protection Agency (Safe Drinking Water Act6,8) for DBP precursor (i.e. NOM/DOM) control.
Electrocoagulation (EC) is an electrolytic process that involves the in situ generation of a metallic coagulant with a supply of an electric current and a sacrificial metal electrode such as aluminium which liberates Al3+.9 EC has been reported to provide a potential advantage by removal of the need for use of chemicals that can be hazardous.10 Risks to safety are reduced as transport, storage and handling of these potentially hazardous chemicals such as acidic coagulants, alkaline reagents for pH control and oxidising chemicals are no longer needed. During the electrocoagulation process, the pH does not change significantly and the process does not require addition of lime or other alkaline chemicals to compensate for low alkalinity of some waters.9,11 EC is also associated with less secondary pollutant generation10,12 (i.e., there is no release of counter ions by EC generation of coagulant, unlike for example, the release of sulphate ions when alum is used) and lower production of sludge.13 These and other advantages10,14 have led to EC being considered as a green/eco-friendly, and potentially cost-effective technology for water and wastewater treatment.13,15–18
In the EC process, the anodic and cathodic reactions, when using aluminium as the sacrificial electrode, are as follows:
Anode: Al → Al(aq)3+ + 3e− |
Cathode: 2H2O + 2e− → H2(g) + 2OH(aq)− |
In the solution: Al(aq)3+ + 6H2O → Al(OH)3(S) + 3H3O(aq)+ |
Based on these reactions, the hydronium ions produced at the anode are compensated for by an equivalent amount of hydroxide ions produced in the cathodic reaction.9,11 Hence, this technology could reduce complexities associated with pH control for enhanced coagulation and minimize the need to account for source waters with low alkalinity but have high dissolved organic matter content. According to Rahman et al. (2021),19 the EC process presents technical advantages over the CC process in terms of chemical storage, for use in small treatment systems, there is no alkalinity depletion, adjustments can be made remotely and it is cost-effective. Further research into electrocoagulation treatment has been suggested by Rahman et al. (2021)19 on total pollutant removal through EC or advanced EC treatment so that the rural community in Sarawak could utilize peat water in their region. Lee and Gagnon (2016)20 reported differences in size and structure of the flocs generated by the EC process versus the CC process. According to Yildiz et al. (2007),21 the electrocoagulation effectiveness can be affected by the chemical composition of the electrolyte. Zaleschi et al. (2012)22 reported differences between CC and EC for COD, TN, TSS, and turbidity removal rates for wastewater. This was linked to the in situ production of the coagulant, gaseous bubble formation and hypochlorite generation in the EC process. Similarly, Ucevli and Kaya (2021)23 reported differences between CC and EC to remove COD and UVA from a greywater sample. However, Phalakornkule et al. (2010)24 reported comparable performances of the EC and CC processes for COD removal from a palm oil mill effluent sample. Jiang et al. (2002)25 reported the superiority of the EC process over CC for treating a model coloured water. However, the same study reported close performances of the EC and CC for DOC and UVA removals for a surface water sample with a DOC content of ∼6 mg L−1. Vepsäläinen et al. (2012)26 reported the effectiveness of the EC process for removing NOM from a surface water sample with a high TOC content (>18 mg L−1). For production of reuse water from wastewater and stormwaters, the use of commercially available EC systems such as WaveIonics,27,28 KASELCO Sur-Flo29 and Genesis Water Technologies (GWT)30,31 has been reported.
Despite the reported advantages of the EC process and the commercially available EC systems that are mainly used for reuse water production, knowledge gaps remain associated with the effectiveness of the EC process for producing drinking water from surface water sources. These include an understanding of EC efficiency for DOM removal along with the associated changes in the characteristics of DOM. The feasibility of feedforward model(s) for prediction of EC doses for controlled removals (maximization or targeted percentages) of DOC/TOC applicable for drinking waters of highly contrasting water qualities needs to be determined.
In this paper is described an electro-coagulation technique that is based on electrically generated aluminium cations, with pH control to maximize DOM removal from surface waters of highly contrasting qualities (referred to here as electro-coagulation [En-EC]). The effects of ICE, pHEC, and coagulant dose (AlEC) on DOM removal efficiency (R%) and energy consumption (CE) were investigated. Changes in the characteristics of DOM following the En-EC process, and residual aluminium and other metals' levels were assessed. This study investigated the comparative performances of EnC and En-EC in removing DOM. A further objective addressed in this study was to assess the feasibility of a feedforward predictive EC dosing system.
2. Materials and methods
2.1 Water sampling
Water samples were collected in September 2020 from the Murray River (Murray Bridge, SA, Australia) and Myponga River (Myponga, SA, Australia) and in February 2020 (after a major bushfire event), from the Middle River (Kangaroo Island, SA, Australia, see Fig. 1). Water quality data of the samples are presented in Table 1. Samples were maintained at 4 °C and experiments were conducted within four weeks of the sample collection, except for the Middle River sample, which was stored for a longer period, specifically for ∼six months before the experiments; this might have affected its characteristics. Nonetheless, this sample provided for a wider range in water quality with a very high level of organic matter and higher fDOM signals than those from the two other sample sites. To ensure validity in comparisons, the raw water samples were analysed for water quality parameters concurrently with EC treated water samples.
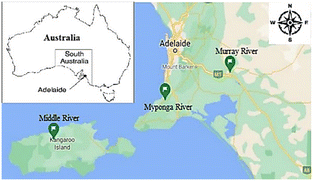 |
| Fig. 1 Location of water sources chosen as the case studies. | |
Table 1 Raw water quality parameters in selected water sources
WQ parameters |
Murray River |
Myponga River |
Middle River |
DOC-specific A254 (L mg−1 m−1).
Corrected fDOMs (RFU) for turbidity, temperature and pH impacts using a mathematical model-based approach which has been developed by us recently (not published yet).
Corrected fDOMs (RFU) for inner filter effect [IFE] impacts using a mathematical model-based approach which has been developed by us recently (not published yet).
|
pH |
7.29
|
7.26
|
7.45
|
Conductivity (μS cm−1) |
260
|
550
|
1113
|
Turbidity (NTU) |
45.7
|
12.1
|
12.6
|
TOC (mg L−1) |
6.2
|
18.2
|
21.7
|
DOC (mg L−1) |
4.8
|
17.4
|
21.5
|
A
254 (m−1) |
13.7
|
90.3
|
89.1
|
True colour (HU) |
14
|
170
|
129
|
SUVA254a |
2.9
|
5.2
|
4.1
|
fDOMs,III (RFU)b |
16.9
|
66.4
|
100.5
|
fDOMs,IV (RFU)c |
17.9
|
112.6
|
167.7
|
Zeta potential (mV) |
−18.2
|
−16.6
|
−9.7
|
2.2 The EC unit
The laboratory-scale EC unit comprised a DC power supply (GPS-18500, GwInstek, Australia), eight aluminium electrodes, a 2-litre square glass jar, a magnet stirrer and a coulomb counter that was developed. The aluminium electrodes (alloy no. 6063) were flat bar strips with dimensions of 3 × 32 × 105 mm. The configuration of the EC unit and the experimental setup are shown in Fig. 2.
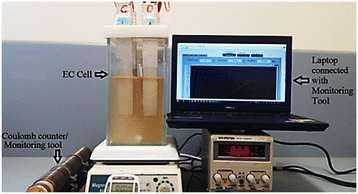 |
| Fig. 2 The EC unit configuration. | |
2.3 Jar tests
En-EC tests were conducted using two litres of water samples and a comparable procedure to the EnC jar testing by alum. The En-EC process comprised four stages including electro-coagulation, flocculation, settling and filtration. The electro-coagulation (first stage) process time was chosen in the range of 10–150 minutes depending on the AlEC and IEC trialled in the experiments. This EC stage is equivalent to the 1-minute rapid mixing stage in the EnC process. The processing times for flocculation and settling (14 and 15 minutes, respectively) were the same as those used for EnC jar tests. The filtration stage was simulated using Whatman® grade 1 filter paper for both En-EC and EnC processes. The pH and conductivity were monitored during the processes using a multi-meter (MultiLab 4010-3W, YSI Inc., USA) equipped with a pH probe. HCl (0.1 M) and NaOH (0.1 M) solutions were used to adjust the pH to a target value prior to and during the EC process.
To choose the AlEC for En-EC process trials for each water sample, it was initially assumed that a DOM removal performance would be achieved by Al3+ released through the En-EC process compared with the same amount of Al3+ from addition of an alum salt solution. This assumption was assessed experimentally as a part of this study (see section 3.5). For this, an approach was taken, as reported in our previous study for alum dose selection for EnC in jar tests,32 to select AlEC for the En-EC jar tests. Hence, the AlEC doses for the six jars in each experiment were determined through the EnD of alum (as Al equivalents) predicted using WTC_Coag™ (https://www.unisaventures.com.au/technologies/wtc-coagtm-coagulant-dose-prediction-application) software7 (then termed as EnDmEnCo,p) and using the raw water quality parameters (i.e., turbidity, colour, and A254) as the parameter inputs to this software for determination of ∼25%, 50%, 75%, 100%, 150%, and 250% of the EnDmEnCo,p. These % dose values provided for a dose range starting from just prior to the rapid DOM removal rate, to the dose where levelling off [plateauing] of DOM removal occurred [i.e. a low DOM removal rate region, where the DOC removal rate is ≤0.015 mg DOC per mg of alum]7 from which an optimum dose (∼EnD) could be experimentally confirmed from these EnC experiments. Based on the assumption of similarity of En-EC and EnC performances for DOM removals, it was expected that the WTC_Coag software dose determination procedure could also be applicable to provide a similar dose range for the En-EC jar test experiments. However, the prediction of AlEC dose based on WTC_Coag software (or alum dose for EnC) for the Myponga River and Middle River samples was lower than what would be needed for enhanced coagulation as the DOC and A254 (cm−1) levels were outside of the software's operational range (i.e., ≲14 mg L−1 DOC and/or <0.55 cm−1A254, see Table 1). Nonetheless, for the investigation reported here, the dose range tested was very wide, being 25% to 250% of the software's output EnD, and then likely to include the actual enhanced dose (for maximizing the removal of DOC).
Using these AlEC doses determined for each experiment and using the Faraday constant (i.e., ∼96
500 C per eq., representing the amount of electric charge (Q) as coulombs (C) carried by one mole of electrons or an equivalent of any electro-active chemical species in electrochemical reactions [like Al3+ in the En-EC process]), the required Q (C) to achieve the selected AlEC dose through the En-EC process can be then calculated (for example, to release each 1 mg L−1 Al3+ dose, we needed to apply 10.7 C L−1 electricity on the electrodes).33
From the Q (C) values determined to achieve the selected AlEC dose for each experiment, the EC stage (stage 1) process time can be calculated using the formula, Q (C) = IEC (amperes) × t (s) (for example, in the case of 10.7 C L−1 and for a two litre jar experiment, we need an ∼214 s EC time if the IEC is maintained at 100 mA).33
The impact of IEC on the En-EC process was investigated over the range of 25 to 750 mA with the AlEC and pHEC maintained under constant conditions. For this, the AlEC was selected near 50% of the EnD value predicted by WTC_Coag software. This was selected as at higher AlEC, the major portion of coagulable DOC content would be removed and the impacts of other operational parameters might not be distinguishable. A constant pHEC value of 6 was selected for IEC experiments, which was previously reported as a suitable process pH value for an alum coagulant in EnC regarding DOM removal performance.34 However, in practice, the pH will be dependent on the alkalinity of raw water, the applied dose of coagulant (not pre-hydrolysed) and the use of any pH control reagent/s.
The pHEC impact on the process was investigated in the range of 5–9 for two of the three water samples, i.e. Murray River and Myponga River (with the widest diversity of DOM characteristics based on SUVA values). This was conducted under constant coagulant dose (i.e., the same ∼50% EnD predicted by WTC_Coag) and IEC (the optimised value attained during the IEC study [previous experimental stage]) conditions.
The coagulant dose influence on the process was investigated for each sample using six different AlEC doses which were determined as previously explained using the WTC_Coag software.7,32
Some of these coagulant doses were also selected for comparison with a conventional jar test procedure using alum (as a 28% alum [aluminium sulphate] solution, IXOM Operations Pty Ltd) following the same procedure described by Daraei et al. (2021).32 The EnC dose experiments (jar tests) were conducted at constant pH (i.e., the same pH which was found as the optimum pH for En-EC and was used for the En-EC jar tests).
2.4 Water quality analyses
Turbidity was measured using a turbidimeter (model 2100n, Hach, USA). Zeta potentials were measured for particles and flocs using a Zetasizer (Nano-ZS ZEN 3600, Malvern Panalytical, UK). TOC/DOC measurements were conducted using a TOC analyser (Model 820, Sievers Instruments, USA). A YSI EXO2 Sonde (Xylem, USA) equipped with an EXO fDOM probe was used to acquire fluorophore DOM fluorescence signals (fDOMs) at excitation/emission paired wavelengths of 365 ± 5 nm/480 ± 40 nm (fDOMs, RFU). The EXO2 Sonde is a multi-parameter (seven ports) instrument that was equipped with a pH probe, a conductivity–temperature probe and a turbidity probe. All probes were calibrated according to the manufacturer's instructions using required buffers and standard solutions.35,36
A spectrophotometer (Model UV-120, Miostech, Australia) was used to measure UV-vis absorbances over the range of 200–700 nm. From these data, the absorbance at 254 nm (A254 [m−1]) and true colour (HU) at 456 nm were acquired. These data were used for inner filter effect (IFE) correction in fluorescence excitation–emission (ex/em) matrix (EEM) spectra as well. Fluorescence excitation–emission (ex/em) spectra were collected using a fluorescence spectrometer (LS55, PerkinElmer, USA) in the range of 200–600 nm ex/em with a 1 cm quartz cuvette. The EEM data were corrected using MATLAB software as described by Murphy et al. (2013).37 The corrected EEM data were then analysed using a peak picking (PP) technique as described in several studies,38–40 fluorescence regional integration (FRI) as described by Chen et al. (2003)41 and parallel factor analysis (PARAFAC [PAR]) as described by Murphy et al. (2013).37 A high-performance size exclusion chromatograph (2690 Separation Module and Waters 996 photodiode array detector, Waters, USA) equipped with either a UV-detector (HPSEC-UVA260), or a humics fluorescence-detector (HPSEC-Fl-Hu, ex/em, 280/330 nm) and a protein fluorescence-detector (HPSEC-Fl-Pr, ex/em, 320/430 nm) was used to collect apparent molecular weight (AMW) profiles, as described by Chow et al. (2008).42 The AMW data were then used to calculate the weight-averaged molecular weight (WAMW) index according to Chow et al. (2008)42 and De Oliveira et al. (2018).35 The HPSEC data were also analysed using a peak fitting (PF) technique with a developed script in MATLAB software for this purpose.
Concentrations of residual aluminium as well as other metals, in treated waters, were determined using an inductively coupled plasma optical emission spectrometer (ICP-OES, Avio 500 ICP-OES, Perkin Elmer, USA) and an inductively coupled plasma mass spectrometer (ICP-MS, Model 7500c, Agilent Technologies, USA). Samples were pre-treated by filtration using 0.45 μm polyether-sulfone membrane filters (Sartorius, Germany) prior to DOC, UV-vis and fEEM data collection. HPSEC analyses were performed on samples after filtration for turbidity removal using 0.22 μm polyether-sulfone membrane filters (Sartorius, Germany).42
2.5 Precision of techniques [coefficient of variation (CV)]
The precisions of water quality analyses and treatment techniques were determined using two water samples collected separately from the same water sources (Murray and Myponga rivers). These had contrasting water qualities, i.e. the Murray River samples having high turbidity but low DOM content and Myponga River samples with high DOM content but low turbidity (see Table A2.1†). The determined CV values of water quality analyses are presented in Table A2.2a† for the Myponga River samples and in Table A2.2b† for the Murray River. The CV values for the treatment techniques (EC and CC) are presented in Table A2.3.† For DOC analysis performed at our laboratory during this study, the mean CV was 2.1%.
3. Results and discussion
3.1 Water quality of samples
Analyses of the water samples confirmed the high contrast between them, for organic matter concentration and characteristics, and turbidity. The sample from the Myponga River had DOM content with the highest SUVA254 value. The Middle River sample had the highest DOM content but had a lower SUVA254 value than the Myponga River sample. In contrast with these two samples, the Murray River sample had low DOC content and SUVA254 value but higher turbidity content (see Table 1). The EnD was estimated as 5.6 mg L−1 Al (i.e., equivalent to 60.1 C L−1 [coulombs per liter]); 16.4 mg L−1 Al (i.e., equivalent to 176 C L−1); and 16 mg L−1 Al (i.e., equivalent to 171.5 C L−1) for the Murray River, Myponga River and Middle River samples using WTC_Coag software (see section 2.3) and raw water quality parameters (see Table 1), respectively.
3.2 Impact of operational parameters on the En-EC process
3.2.1 Impact of IEC on the En-EC process.
The impact of IEC on R% (measured as A254 removal) and CE (W h per kg of released Al3+) during the En-EC process was assessed and the results are presented in Fig. 3a and b, respectively. For the range of IEC values studied, the IEC had a minimal impact on the R% (Fig. 3a) whilst a higher IEC can cause a significantly higher CE (Fig. 3b) and voltage (v). Optimum IEC values (determined from the ∼levelling off point of CE and voltage versus IEC diagrams, see Fig. 3b) of ∼0.10 A (amperes) for the Myponga River sample in comparison with the value of ∼0.05 A for the Murray River sample can be explained by the higher water conductivity (about twice) of the Myponga River sample which allowed it to achieve higher IEC values at lower voltage and CE (see Table 1 and Fig. 3). Similarly, a higher optimum IEC value of ≳0.2 A for the Middle River sample in comparison with ∼0.1 A for the Myponga River sample can be explained by the higher water conductivity (>2 times) of the Middle River sample which allowed it to achieve higher IEC values at lower voltage and CE. The optimum IEC showed a direct correlation with the raw water sample conductivity.
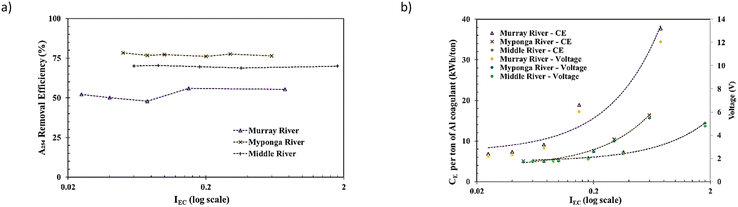 |
| Fig. 3 The influence of IEC on a) organic matter removal measured as A254% reduction and b) CE during En-EC (pH 6 and coagulant dose of 2 mg L−1 Al [i.e. equivalent to ∼42.9 C L−1] for the Murray River sample, 8.4 mg L−1 Al [i.e. equivalent to ∼180 C L−1] for the Myponga River sample and 5 mg L−1 Al [i.e., equivalent to ∼108 C L−1] for the Middle River sample). | |
This indicates that the coagulant dose is the determinant parameter for R% during the En-EC process rather than the voltage applied on the electrodes or IEC [the rate of releasing Al3+]. To achieve a faster process (higher IEC, less process time), a higher applied voltage and CE would be required. The rate of increase in CE or voltage for increasing IEC would become higher due to the occurrence of lower faradaic performance at higher applied IEC and voltage levels.33,43,44 This generally means a less energy efficient EC process at IEC levels higher than the optimum. This lower energy efficiency might be exacerbated by selection of a higher level of IEC.
3.2.2 Impact of pHEC on the En-EC process.
The results for the effects of pHEC on R% and CE during the En-EC process are shown in Fig. 4a and b, respectively. This was conducted for two of the samples, with the lowest and highest SUVA254 values. The results indicate that the effect of pHEC on the CE is insignificant for the pH range studied, particularly in the case of Myponga River. The obtained CE values were 6.1 ± 0.3 W h per kg of released Al3+ (mean ± SD) and 5.58 ± 0.02 W h per kg of released Al3+ (mean ± SD) for Murray River and Myponga River, respectively, over the range of pH values studied. The slightly decreasing trend in CE with a decrease in the pHEC might be due to the impact of higher hydronium ion content with the highest ionic conductivity,45 which can slightly increase the electric conductivity (and consequently decrease the voltage and CE) in the Murray River sample with a lower level of initial conductivity. This impact for the Myponga River with a higher level of initial conductivity may not be distinguishable. The results indicate that a pHEC of more than 6 can significantly decrease R% during En-EC. Similar results and an operational optimum pH of around 6 have been previously reported for alum to remove dissolved organic matter during EnC.7,46
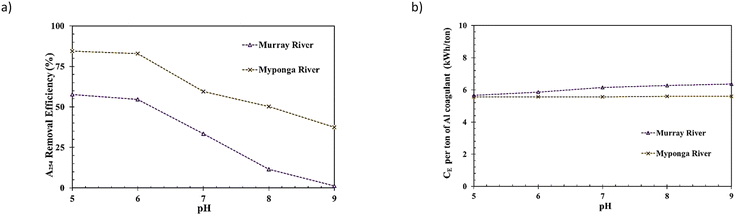 |
| Fig. 4 The influence of pHEC on a) R% and b) CE during En-EC (IEC = 0.05 A and 0.10 A, coagulant dose of 2 mg L−1 Al [i.e. equivalent to ∼42.9 C L−1] for the Murray River sample and 8.4 mg L−1 Al [i.e. equivalent to ∼180 C L−1] for the Myponga River sample). | |
3.2.3 Impact of AlEC dose on the En-EC process.
3.2.3.1 Impact of AlEC dose on CE.
The impacts of Al dose on the CE (W h per L of treated sample) are shown in Fig. 5a–c for the Murray River, Myponga River and Middle River samples, respectively. The results indicate that the CE (W h per L of treated sample) is directly and linearly correlated with the AlEC dose. This shows that the Faraday efficiencies for all experiments and trialled IEC and voltage are similar and might be near 100%. A mean value of 5.83 ± 0.21 W h per kg of released Al3+ for all 18 jar tests performed on the three samples (5.91 ± 0.10, 5.60 ± 0.17 and 5.98 ± 0.10 for Murray River, Myponga River and Middle River, respectively) and its low variation (i.e., SD less than 4% of the mean) might be further evidence of the similar and high Faraday efficiency. These average CE values were determined for the three samples in an energy efficient condition (with low [∼0] overvoltage and faradaic losses). The observed relationship between CE and alum dose indicates the necessity for a dose control system for saving both energy and sacrificial electrode mass during the En-EC process.
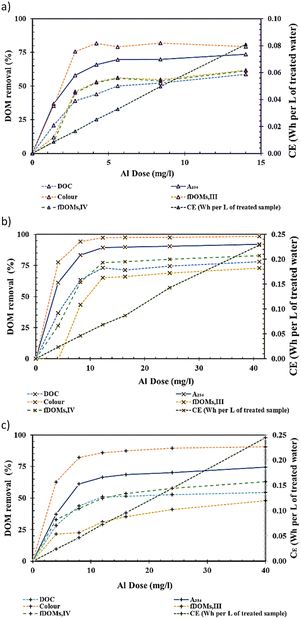 |
| Fig. 5 The influence of AlEC dose (as Al) on DOM removal performance (measured as % of DOC, A254, colour and fDOM removal) and CE (measured as W h per L of treated samples) during En-EC for the a) Murray River (pH, 6, IEC, 0.05 A), b) Myponga River (pH, 6, IEC, 0.1 A) and c) Middle River (pH, 6, IEC, 0.18 A) samples. | |
3.2.3.2 Impact of AlEC dose on DOM removal performance.
The impacts of AlEC dose on DOM removal (measured as percentages of DOC, A254, colour or fDOMs removals) for Murray River, Myponga River and Middle River are shown in Fig. 5a–c, respectively. A trend of high to lower removal rates (removal % rate per applied AlEC dose) was observed for all DOM proxy parameters measured, i.e. DOC, A254, colour and fDOMs, following En-EC (see Fig. 5). Also, the presence of a recalcitrant (non-coagulable) DOM fraction (also measured as DOC), CDOM (measured as A254) and fDOM (measured as fDOMs) was evident from the levels remaining even after a very high alum dose was applied (i.e., 250% of EnDmEnCo,p), as shown in Fig. 5. These are in line with previous reports on the EnD process by Kastl et al. (2004)47 and van Leeuwen et al. (2005).48
Maximum DOC removals of ∼58%, ∼78% and ∼54% were observed for Murray River, Myponga River and Middle River, respectively. The different removal efficiencies (coagulability) observed for the different water sources can be attributed to different organic characteristics e.g., SUVA254, of the three samples studied (see Table 1). The Myponga River sample had a higher SUVA254 than the other two samples (i.e., 5.2 m L mg−1, see Table 1) since it contains a higher ratio of coagulable organic matter which can be removed compared to the Murray River and Middle River samples with lower SUVA254. A higher SUVA value indicates that the composition of organic compounds present is largely of aquatic humic substances which are higher in molecular weight and have a relatively higher content of hydrophobic and aromatic components.49 This fraction of the DOM pool is known to be more coagulable while water samples with lower SUVA254 have a higher DOM composition of lower molecular weight compounds, more hydrophilic in nature and less able to be removed by the coagulation process.49,50
3.2.3.3 Impact of AlEC dose on DOM removal performance.
The DOC removal results for the three samples studied are in the range of previously described fractions of DOC in natural waters by Kastl et al. (2004),47i.e. humic acid groups (20–50%), nonpolar groups (20–50%), and non-sorbable groups (20–50%). The coagulable DOC fraction consists of humic acid groups (i.e., independent from the process pH) and the coagulable part of the non-polar group fraction at the given process pH (i.e. dependent on the process pH). This means ∼40–80% of the DOC content has been described as a coagulable fraction by Kastl et al. (2004)47 depending on the pH of the process. The experimentally obtained 54–78% values for % coagulable DOC fall in this ∼40–80% range.
Comparable removal trends were observed for other DOM proxy parameters (i.e., A254, colour and fDOMs) for the Murray, Myponga and Middle River waters, but with differences in their overall removals i.e., ∼74%, 92% and 75% in the case of A254, 79%, 99% and 91% for colour removals, 62%, 73% and 48% for ffDOMs,III removals and 61%, 83% and 63% for fDOMs,IV removals. These data show the higher coagulability of humic substances51 and chromomeric DOM (CDOM) fractions in the DOM pool which is well established for the EnC process.47 Similar trends of changes in fDOMs with alum dosing by the EC process compared with CC shows the potential of the fDOMs for tracking DOM content following the EC process. This also indicates the potential of the fDOMs for EC alum dose prediction, as previously reported for chemical coagulation using A254 & colour parameters by van Leeuwen et al. (2009)7 and DOC signals by Daraei et al. (2021).32
3.2.3.4 Impact of AlEC dose on turbidity and zeta potential.
The changes in turbidity and zeta potential were monitored for the raw and treated water samples following En-EC. The results are presented for Murray River, Myponga River and Middle River in Fig. 6a–c, respectively. A high removal rate for turbidity was observed with the increase in Al dose prior to the EnD point. This was followed by a levelling off (plateauing) point near EnD of AlEC and fluctuation which might be due to destabilization of flocs/turbidity by a higher dose than EnD.52,53 Maximum % removals of 97%, 93% and 94% were obtained for these water samples, respectively. In the tests conducted, we observed larger sized flocs and sludge volumes but with lower settleability for the EC produced flocs in comparison with those from the chemical coagulation process.
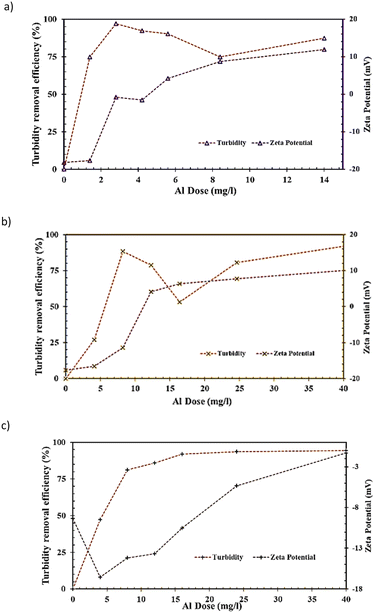 |
| Fig. 6 The influence of AlEC dose (as Al) on turbidity removal and zeta potential changes during En-EC for a) Murray River (pH, 6, IEC, 0.05 A), b) Myponga River (pH, 6, IEC, 0.1 A) and c) Middle River (pH, 6, IEC, 0.18 A). | |
In the case of the zeta potential, the results show a general increasing trend with increasing AlEC dose, which is a well-established feature.54 Almost similar zeta potentials (∼+3, +5 and +5 mV for Murray River, Myponga River and Middle River) were observed near the EnD (i.e., 4.7, 14.1 and 12.7 mg L−1 for the same samples, respectively, see Fig. 6). It is important to note that the turbidity is the controlling factor for AlEC dose in the Murray River sample whereas the DOM is the dominant factor that influences the AlEC dose in the case of the Myponga River and Middle River samples.
3.3 Residual Al and other metals/heavy metals following En-EC
ICP-OES followed by ICP-MS showed insignificant levels of residual AlEC and other measured metal and heavy metal species, for both En-EC and EnC processes (see Table S1, ESI†). The residual aluminium levels for all the samples were low (<20 μg L−1 using a MS detector but non-detectable [nd] using an OES detector) except in the case of a Murray River treated sample with the highest AlEC dose of ∼14 mg L−1, which showed an ∼1 mg L−1 AlEC residual (this was observed in both OES and MS detectors). The residuals of other measured metals, heavy metals and elements (i.e., Ag, As, B, Ba, Be, Ca, Cd, Co, Cr, Cu, Fe, K, Mg, Mn, Na, Ni, P, Pb, S, Sb, Se, Si, Sr, Ti, U, V and Zn) following either En-EC or EnC were comparable to their levels in the corresponding raw water samples (see Table S1, ESI†).
3.4 Tracking organic matter characteristics following En-EC
3.4.1 SUVA254, sp. colour and sp. fDOMs.
The results of tracking SUVA254, sp. colour and sp. fDOMs with increasing Al3+ by En-EC are shown in Fig. 7. The SUVA254 and sp. colour levels decreased with the En-EC process corresponding to the CC process. However, the sp. fDOMs showed a different trend with increasing AlEC dose during coagulation. This may be partially due to the different impacts of interfering factors such as turbidity and IFE on the fDOMs in raw versus treated waters considering their differences in turbidity and DOM concentration and characteristics. This indicates a further challenge for online fDOMs monitoring for tracking the DOM characteristics with the EC process.
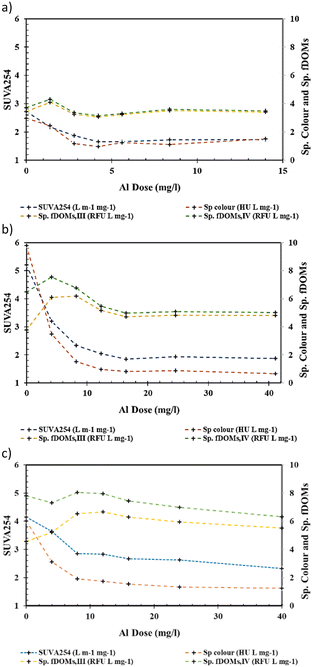 |
| Fig. 7 The influence of Al dose on DOM optical characteristics (measured as SUVA254, sp. colour and sp. fDOMs) following En-EC for the a) Murray River (pH, 6, IEC, 0.05 A), b) Myponga River (pH, 6, IEC, 0.1 A) and c) Middle River (pH, 6, IEC, 0.18 A) samples. | |
3.4.2 Fluorescence EEM spectra.
Spectra of EEM data after blank correction, masking and smoothing primary and secondary Rayleigh peaks, IFE correction, and conversion of signal intensity to standardized Raman units (RU) are shown in Fig. S1a and Table S2, ESI.† The corrected fEEM data were then used for extraction of several fluorescence indices through the PP, FRI and PARAFAC approaches. The results are presented in Fig. S1b, ESI.† Details of the PARAFAC model development for the EEM spectra of the raw and treated water samples, the selection procedure of the number of the model components, and the PARAFAC model statistical validation are presented in Appendix 1, ESI.† The EEM data presented in Fig. S1† show the relationship between fDOMs removal and alum coagulant dose in all ex/em wavelength regions. The PP and FRI results showed a level off in EEM fDOMs removal near the EnD as well as a difference in the removal rate for the extracted indices (see Fig. S1 and Table S2, ESI†).
3.4.3 HPSEC data for tracking DOM characteristic changes.
HPSEC-UVA260 based AMW profiles/distributions as well as the AMW based indices e.g., WAMW and decomposed molecular size component (PF peaks) changes before and following En-EC, are shown in Fig. S2a.† The removal rate of HPSEC components with a higher MW range was higher following electro-coagulation which agrees with previous reports.55 Similar results were obtained for HPSEC-Fl-Hu based AMW data and are presented in Fig. S2b, ESI.†
3.5 Comparison of En-EC with EnC
The removal of DOM measured as % removals of A254 and true colour was used to compare the En-EC process with EnC. The results are shown in Fig. 8. En-EC and EnC showed comparable performances for the samples studied. Differences of 10–15% were observed for A254 and true colour values for the Murray River sample (see Fig. 8a). This sample had a relatively low DOM content, particularly after treatment with alum doses at Al ≥ EnD. The CV data for treated Murray River water [A254, EC: 7.8%, CC: 0.9%; colour, EC: 17%, CC: 7%] indicate that the differences detailed above are not significant [see Table A2.3, ESI†].
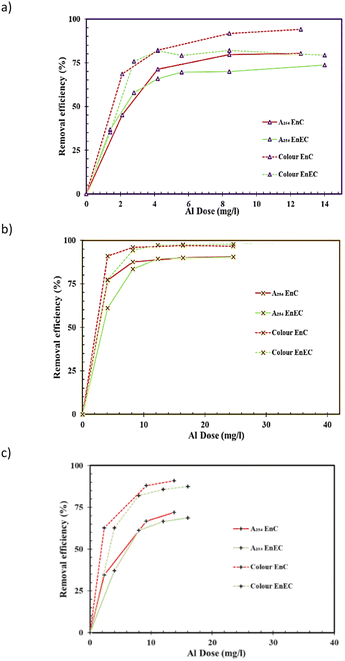 |
| Fig. 8 The comparison of En-EC with EnC for DOM removal performance (measured as % of A254 and colour removal) in a) the Murray River (pH, 6, IEC, 0.05 A), b) Myponga River (pH, 6, IEC, 0.10 A) and c) Middle River (pH, 6, IEC, 0.18 A) samples. | |
Several factors have been linked to the EC efficiency in removing pollutants including the electrode material, EC process time, EC cell voltage, EC cell electric current, electrolyte concentration and type, pH, temperature, distances between electrodes, electrode shape, EC cell structure and configuration of electrode electrical connections.21,26,56–64 In this study, the performance of the EC process for DOM removal (using an aluminium electrode, at the optimum pH and ambient temperature) was found to be related to the generated coagulant dose. This was also found to be similar to the comparative chemical coagulant dosing (CC) process. The optimum energy consumption for the water samples studied with particular electric conductivities was found to be related to the electric current density which could readily enable control of the EC process as well.
The observed similarity of the performances of the two processes for DOM removal was considered as a basis for a potential feedforward dose control system for the En-EC process. A previously developed alum dose control model32 was applied for this purpose. The adaption was performed by converting the predicted aluminium dose, based on raw water DOC and turbidity, to an equivalent Q (C) value. This then transforms the Q (C) value to the process time and IEC (as previously described, see section 2.3). To test the applicability of this for the samples studied, the EnD was determined from data of DOC removals for the En-EC process following the same methodology previously detailed for the EnC process.32 Each electro jar test for a particular surface water sample provided seven DOC data points (from six electro jars plus raw water data, see Fig. 5) and these were fitted to an exponential decay function using Table Curve© software 2D Windows v4.00 (Hearne Software Pty Ltd, Melbourne, Victoria). The first derivatives of the fitted model to the jar test data were generated, and used to extract the EnD data.32 The experimentally attained EnD values for the three samples were then compared to the predictions obtained through the DOC model software (EnDDOC,P). EnDDOC,P values of 6.1, 13.5 and 16.3 mg L−1 Al were obtained for the Murray River, Myponga River and Middle River water samples, respectively, where the experimentally attained EnDDOC values were 4.7, 14.1 and 12.7 mg L−1 for the same samples, respectively. A further study incorporating a larger water sample set (and from more diverse sources) is suggested to verify these models for application of En-EC for targeted DOC removal predictions. An image of the WTC-DOC_ECoag software is presented in Fig. S3.†
4. Conclusion
The findings of this study provided evidence for the potential application of the En-EC process for controlled and enhanced DOM removal from surface waters, including those where DOM levels are very high. DOM is a precursor in the formation of DBPs where chlorine-based disinfection is applied and thus, based on this study, the technology has potential to be applied for control of DBP formation. The En-EC process showed comparable performance to the chemical EnC process for removal of DOM. The pH of water impacted the DOM removal performance in the En-EC process comparable to the EnC process. Due to the compensative anodic and cathodic reactions, the control of pH in the En-EC process is less challenging, being less subject to change. The energy consumption rate during the process is inversely related to the EC process time. The optimum rate of in situ electro-production of coagulant through anodic reaction (i.e., related to the IEC value) is found to be directly dependent on the sample's conductivity. A cost–benefit analysis is needed to determine the practicality of En-EC as an alternative for the EnC process. However, the proposed En-EC process might be more favourable in the case of raw waters with higher conductivity (enabling rapid electrode reaction for free ion release and having a lower energy consumption rate) and low alkalinity (which does not need to be compensated for in the En-EC process). Importantly, the En-EC process showed a similar efficacy to EnC for DOM removal based on characteristics (SUVA254, ratio of humic-like to protein-like fractions and WAMW). Further investigation is suggested on the basis of the EC process being a potential eco-friendly process applicable for surface water treatment at DWTPs.
Conflicts of interest
There are no conflicts to declare.
Acknowledgements
This research was funded by the Australian Government through the Australian Research Council (ARC, LP160100217); we thank all the academic and industry partners (i.e., SEQWater, Melbourne Water and Xylem Analytics Australia) for their contributions. The authors gratefully acknowledge the guidance of Dr Kathleen Murphy of Chalmers University on the PARAFAC model development. The authors also gratefully acknowledge the technical support provided by Dr Henry Senko, Mr Gregory O'Neil and Mr Tim Golding of the UniSA STEM. This is an extended version of a CEST2021 conference oral presentation (https://cest.gnest.org/e/cest-2021?room_id=4&date=2021-09-03%2000%<?pdb_no 3A00?>3A00<?pdb END?>%<?pdb_no 3A00?>3A00<?pdb END?>), prepared for publication as an invited research full paper in the special issue, in Environmental Science: Water Research and Technology.
References
- National Water Commission (Australia), National performance report 2016-17: Urban water utilities, https://www.bom.gov.au/water/npr/docs/2016-17/nationalPerformanceReport2016_17UrbanWaterUtilitiesHigherRes.pdf, accessed 27/5/ 2022.
- G. Bwire, D. A. Sack, A. Kagirita, T. Obala, A. K. Debes, M. Ram, H. Komakech, C. M. George and C. G. Orach, The quality of drinking and domestic water from the surface water sources (lakes, rivers, irrigation canals and ponds) and springs in cholera prone communities of Uganda: an analysis of vital physicochemical parameters, BMC Public Health, 2020, 20, 1–18 CrossRef PubMed.
- N. H. Tran, H. H. Ngo, T. Urase and K. Y. H. Gin, A critical review on characterization strategies of organic matter for wastewater and water treatment processes, Bioresour. Technol., 2015, 193, 523–533 CrossRef CAS.
- S. Hussain, J. Awad, B. Sarkar, C. W. Chow, J. Duan and J. van Leeuwen, Coagulation of dissolved organic matter in surface water by novel titanium (III) chloride: Mechanistic surface chemical and spectroscopic characterisation, Sep. Purif. Technol., 2019, 213, 213–223 CrossRef CAS.
- S. Tak and B. P. Vellanki, Natural organic matter as precursor to disinfection byproducts and its removal using conventional and advanced processes: state of the art review, J. Water Health, 2018, 16, 681–703 CrossRef.
- Safe Drinking Water Act, US EPA, https://www.epa.gov/sdwa, accessed June 2021.
- J. van Leeuwen, M. Holmes, U. Kaeding, R. Daly and D. Bursill, Development and implementation of the software mEnCo© to predict coagulant doses for DOC removal at full-scale WTPs in South Australia, J. Water Supply: Res. Technol.--AQUA, 2009, 58, 291–298 CrossRef CAS.
- US EPA Office of Water, Enhanced coagulation and enhanced precipitative softening guidance manual, EPA 815-R-99-012, May 1999 Search PubMed.
- S. Garcia-Segura, M. M. S. Eiband, J. V. de Melo and C. A. Martínez-Huitle, Electrocoagulation and advanced electrocoagulation processes: A general review about the fundamentals, emerging applications and its association with other technologies, J. Electroanal. Chem., 2017, 801, 267–299 CrossRef CAS.
- J. N. Hakizimana, B. Gourich, M. Chafi, Y. Stiriba, C. Vial, P. Drogui and J. Naja, Electrocoagulation process in water treatment: A review of electrocoagulation modeling approaches, Desalination, 2017, 404, 1–21 CrossRef CAS.
-
N. Yasri, J. Hu, M. G. Kibria and E. P. Roberts, in Multidisciplinary Advances in Efficient Separation Processes, ACS Publications, 2020, pp. 167–203 Search PubMed.
- M. Reilly, A. P. Cooley, D. Tito, S. A. Tassou and M. K. Theodorou, Electrocoagulation treatment of dairy processing and slaughterhouse wastewaters, Energy Procedia, 2019, 161, 343–351 CrossRef CAS.
- C. H. Neoh, Z. Z. Noor, N. S. A. Mutamim and C. K. Lim, Green technology in wastewater treatment technologies: integration of membrane bioreactor with various wastewater treatment systems, Chem. Eng. J., 2016, 283, 582–594 CrossRef CAS.
-
K. S. Hashim, R. AlKhaddar, A. Shaw, P. Kot, D. Al-Jumeily, R. Alwash and M. H. Aljefery, in Advances in Water Resources Engineering and Management, Springer, 2020, pp. 219–235 Search PubMed.
- K. S. Hashim, R. Al Khaddar, N. Jasim, A. Shaw, D. Phipps, P. Kot, M. O. Pedrola, A. W. Alattabi, M. Abdulredha and R. Alawsh, Electrocoagulation as a green technology for phosphate removal from River water, Sep. Purif. Technol., 2019, 210, 135–144 CrossRef CAS.
-
D. Ghernaout, Electrocoagulation process: Achievements and green perspectives, Colloid and Surface Science, 2018, vol. 3, pp. 1–5 Search PubMed.
- D. Ghernaout, Disinfection via electrocoagulation process: Implied mechanisms and future tendencies, EC Microbiol., 2019, 15, 79–90 Search PubMed.
- N. Meunier, P. Drogui, C. Montané, R. Hausler, G. Mercier and J.-F. Blais, Comparison between electrocoagulation and chemical precipitation for metals removal from acidic soil leachate, J. Hazard. Mater., 2006, 137, 581–590 CrossRef CAS.
- N. A. Rahman, C. J. Jol, A. A. Linus and V. Ismail, Emerging application of electrocoagulation for tropical peat water treatment: a review, Chem. Eng. Process., 2021, 165, 108449 CrossRef CAS.
- S. Y. Lee and G. A. Gagnon, Comparing the growth and structure of flocs from electrocoagulation and chemical coagulation, J. Water Process. Eng., 2016, 10, 20–29 CrossRef.
- Y. Ş. Yildiz, A. S. Koparal, Ş. İrdemez and B. Keskinler, Electrocoagulation of synthetically prepared waters containing high concentration of NOM using iron cast electrodes, J. Hazard. Mater., 2007, 139, 373–380 CrossRef CAS PubMed.
- L. Zaleschi, C. Teodosiu, I. Cretescu and M. A. Rodrigo, A comparative study of electrocoagulation and chemical coagulation processes applied for wastewater treatment, Environ. Eng. Manage. J., 2012, 11(8), 1517–1525 CrossRef CAS.
- O. Ucevli and Y. Kaya, A comparative study of membrane filtration, electrocoagulation, chemical coagulation and their hybrid processes for greywater treatment, J. Environ. Chem. Eng., 2021, 9, 104946 CrossRef CAS.
- C. Phalakornkule, J. Mangmeemak, K. Intrachod and B. Nuntakumjorn, Pretreatment of palm oil mill effluent by electrocoagulation and coagulation, ScienceAsia, 2010, 36, 142–149 CrossRef CAS.
- J.-Q. Jiang, N. Graham, C. André, G. H. Kelsall and N. Brandon, Laboratory study of electro-coagulation–flotation for water treatment, Water Res., 2002, 36, 4064–4078 CrossRef CAS.
- M. Vepsäläinen, M. Pulliainen and M. Sillanpää, Effect of electrochemical cell structure on natural organic matter (NOM) removal from surface water through electrocoagulation (EC), Sep. Purif. Technol., 2012, 99, 20–27 CrossRef.
-
R. A. Caschette, Development of an electrocoagulation based treatment train for produced water with high concentrations of organic matter, Master of Science, Dept of Civil and Environmental Engineering, Colorado State University, 2016 Search PubMed.
-
L. Doty, Electrocoagulation (EC) and chitosan enhanced sand filtration (CESF) treatment technologies for dredge return water: Two case studies on the lower Duwamish Waterway in Seattle, Washington.
- H. A. Moreno-Casillas, D. L. Cocke, J. A. Gomes, P. Morkovsky, J. Parga and E. Peterson, Electrocoagulation mechanism for COD removal, Sep. Purif. Technol., 2007, 56, 204–211 CrossRef CAS.
- S. R. Sakhel and S.-U. Geissen, Predesign cost estimation of a potential wastewater treatment plant for Jordan petroleum refinery-Electrocoagulation, Environ. Processes, 2022, 9, 1–30 CrossRef.
- E. Magnisali, Q. Yan and D. V. Vayenas, Electrocoagulation as a revived wastewater treatment method-practical approaches: a review, J. Chem. Technol. Biotechnol., 2022, 97, 9–25 CrossRef CAS.
-
H. Daraei, J. Awad, E. Bertone, R. A. Stewart, C. W. K. Chow, J. Duan and J. van Leeuwen, A model based on DOC data for determination of alum dosing for drinking water treatment, MODSIM2021, 24th International Congress on Modelling and Simulation, Modelling and Simulation Society of Australia and New Zealand, The University of Sydney, 2021 Search PubMed.
- C. Van Genuchten, K. Dalby, M. Ceccato, S. Stipp and K. Dideriksen, Factors affecting the Faradaic efficiency of Fe (0) electrocoagulation, J. Environ. Chem. Eng., 2017, 5, 4958–4968 CrossRef CAS.
- J. Xie, D. Wang, J. van Leeuwen, Y. Zhao, L. Xing and C. W. Chow, pH modeling for maximum dissolved organic matter removal by enhanced coagulation, J. Environ. Sci., 2012, 24, 276–283 CrossRef CAS.
- G. F. De Oliveira, E. Bertone, R. A. Stewart, J. Awad, A. Holland, K. O'Halloran and S. Bird, Multi-parameter compensation method for accurate in situ fluorescent dissolved organic matter monitoring and properties characterization, Water, 2018, 10, 1146 CrossRef.
-
YSI Incorporated, a Xylem brand, EXO2: EXO User Manual PLATFORM, ADVANCED WATER QUALITY MONITORING, 2017.
- K. R. Murphy, C. A. Stedmon, D. Graeber and R. Bro, Fluorescence spectroscopy and multi-way techniques. PARAFAC, Anal. Methods, 2013, 5, 6557–6566 RSC.
- H. Hudori, M. Y. Rosadi, T. Yamada, S. A. Bhat and F. Li, Effect of the recycling process on drinking water treatment: evaluation based on fluorescence EEM analysis using the Peak-Picking technique and Self-Organizing Map, Water, 2021, 13, 3456 CrossRef CAS.
- J. B. Fellman, E. Hood and R. G. Spencer, Fluorescence spectroscopy opens new windows into dissolved organic matter dynamics in freshwater ecosystems: A review, Limnol. Oceanogr., 2010, 55, 2452–2462 CrossRef CAS.
- E. M. Carstea, A. Baker, M. Bieroza and D. Reynolds, Continuous fluorescence excitation–emission matrix monitoring of river organic matter, Water Res., 2010, 44, 5356–5366 CrossRef CAS.
- W. Chen, P. Westerhoff, J. A. Leenheer and K. Booksh, Fluorescence excitation− emission matrix regional integration to quantify spectra for dissolved organic matter, Environ. Sci. Technol., 2003, 37, 5701–5710 CrossRef CAS PubMed.
- C. W. Chow, R. Fabris, J. V. Leeuwen, D. Wang and M. Drikas, Assessing natural organic matter treatability using high performance size exclusion chromatography, Environ. Sci. Technol., 2008, 42, 6683–6689 CrossRef CAS PubMed.
- Z. Gu, Z. Liao, M. Schulz, J. R. Davis, J. C. Baygents and J. Farrell, Estimating dosing rates and energy consumption for electrocoagulation using iron and aluminum electrodes, Ind. Eng. Chem. Res., 2009, 48, 3112–3117 CrossRef CAS.
- M. Ingelsson, N. Yasri and E. P. Roberts, Electrode passivation, faradaic efficiency, and performance enhancement strategies in electrocoagulation—A review, Water Res., 2020, 187, 116433 CrossRef CAS PubMed.
- C. Zhong, Y. Deng, W. Hu, J. Qiao, L. Zhang and J. Zhang, A review of electrolyte materials and compositions for electrochemical supercapacitors, Chem. Soc. Rev., 2015, 44, 7484–7539 RSC.
- A. Matilainen, M. Vepsäläinen and M. Sillanpää, Natural organic matter removal by coagulation during drinking water treatment: a review, Adv. Colloid Interface Sci., 2010, 159, 189–197 CrossRef CAS PubMed.
- G. Kastl, A. Sathasivan, I. Fisher and J. Van Leeuwen, Modeling DOC removal enhanced coagulation, J. – Am. Water Works Assoc., 2004, 96, 79–89 CrossRef CAS.
- J. Van Leeuwen, R. Daly and M. Holmes, Modeling the treatment of drinking water to maximize dissolved organic matter removal and minimize disinfection by-product formation, Desalination, 2005, 176, 81–89 CrossRef CAS.
- J. Edzwald, Coagulation in drinking water treatment: particles, organics and coagulants, Water Sci. Technol., 1993, 27, 21–35 CrossRef CAS.
- S. W. Krasner and G. Amy, Jar-test evaluations of enhanced coagulation, J. – Am. Water Works Assoc., 1995, 87, 93–107 CrossRef CAS.
- C. W. Chow, R. Fabris, M. Drikas and M. Holmes, A case study of treatment performance and organic character, J. Water Supply: Res. Technol.--AQUA, 2005, 54, 385–395 CrossRef CAS.
- U. Nobbmann, A. Morfesis, J. Billica and K. Gertig, The role of zeta potential in the optimization of water treatment, Nanotech, 2010, 3, 605–607 CAS.
- S. Muyibi and A. Alfugara, Treatment of surface water with Moringa Oleifera seed extract and alum–a comparative study using a pilot scale water treatment plant, Int. J. Environ. Stud., 2003, 60, 617–626 CrossRef CAS.
- E. Sharp, J. Banks, J. Billica, K. Gertig, R. Henderson, S. Parsons, D. Wilson and B. Jefferson, Application of zeta potential measurements for coagulation control: Pilot-plant experiences from UK and US waters with elevated organics, Water Sci. Technol.: Water Supply, 2005, 5, 49–56 CAS.
- J. Awad, J. van Leeuwen, C. W. K. Chow, R. J. Smernik, S. J. Anderson and J. W. Cox, Seasonal variation in the nature of DOM in a river and drinking water reservoir of a closed catchment, Environ. Pollut., 2017, 220, 788–796 CrossRef CAS PubMed.
- J. N. Hakizimana, B. Gourich, C. Vial, P. Drogui, A. Oumani, J. Naja and L. Hilali, Assessment of hardness, microorganism and organic matter removal from seawater by electrocoagulation as a pretreatment of desalination by reverse osmosis, Desalination, 2016, 393, 90–101 CrossRef CAS.
- M. Chafi, B. Gourich, A. Essadki, C. Vial and A. Fabregat, Comparison of electrocoagulation using iron and aluminium electrodes with chemical coagulation for the removal of a highly soluble acid dye, Desalination, 2011, 281, 285–292 CrossRef CAS.
- B. Merzouk, B. Gourich, K. Madani, C. Vial and A. Sekki, Removal of a disperse red dye from synthetic wastewater by chemical coagulation and continuous electrocoagulation. A comparative study, Desalination, 2011, 272, 246–253 CrossRef CAS.
- J. Pinedo-Hernández, R. Paternina-Uribe and J. Marrugo-Negrete, Alternative electrocoagulation for livestock wastewater treatment, Port. Electrochim. Acta, 2016, 34, 277–285 CrossRef.
- Q.-Y. Feng, X.-D. Li, Y.-J. Cheng, M. Lei and Q.-J. Meng, Removal of humic acid from groundwater by electrocoagulation, J. China Univ. Min. Technol., 2007, 17, 513–520 CrossRef CAS.
- S. Kumari and R. N. Kumar, How effective aerated continuous electrocoagulation can be for tetracycline removal from river water using aluminium electrodes?, Chemosphere, 2022, 305, 135476 CrossRef CAS PubMed.
- Y.-M. Chen, W.-M. Jiang, Y. Liu and Y. Kang, Quantitative contribution study and comparison between electrocoagulation, anode-electrocoagulation and chemical coagulation using polymer-flooding sewage, Chemosphere, 2020, 250, 126128 CrossRef CAS PubMed.
- T. Harif, M. Khai and A. Adin, Electrocoagulation versus chemical coagulation: Coagulation/flocculation mechanisms and resulting floc characteristics, Water Res., 2012, 46, 3177–3188 CrossRef CAS PubMed.
- E. Mohora, S. Rončević, B. Dalmacija, J. Agbaba, M. Watson, E. Karlović and M. Dalmacija, Removal of natural organic matter and arsenic from water by electrocoagulation/flotation continuous flow reactor, J. Hazard. Mater., 2012, 235, 257–264 CrossRef PubMed.
|
This journal is © The Royal Society of Chemistry 2023 |
Click here to see how this site uses Cookies. View our privacy policy here.