Understanding the impact of different source water types on the biofilm characteristics and microbial communities of manganese removing biofilters†
Received
22nd July 2022
, Accepted 1st November 2022
First published on 4th November 2022
Abstract
There is growing regulatory interest for Mn in drinking water due to health concerns and an improved understanding of Mn accumulation within distribution systems. Biofiltration can remove Mn from drinking water through biological mechanisms, including oxidation of dissolved Mn(II) by manganese oxidizing bacteria. The influence of source water type (groundwater vs. a mixture of groundwater/surface water) on the composition of filter media biofilm was investigated in three full-scale biofilters which have a history of sustained Mn removal in New Brunswick, Canada. Each location used the same treatment design, including upstream aeration and media type and depth, which removed many variables that may make it difficult to isolate the impact of source water type specifically. The average raw water Mn, Fe, and NH3 concentrations ranged from 370–950 μg L−1, 35–2070 μg L−1, and 0.03–0.04 mg L−1 respectively. The average Mn, Fe, and NH3 removal rates ranged from 98–99%, 78–98%, and 12–54% respectively. Mn, Fe, NH3, NO2−, and CH4 oxidizing microbial communities were characterized in detail through 16S rRNA marker gene sequencing. Although there was little difference in biofilter influent water chemistry, the raw water active biomass was significantly greater (p < 0.05) in the mixed-water biofilter and the mixed-water microbial community was distinct from the groundwater microbial community (p < 0.001). Additionally, active biomass on the surface of biofilter media was significantly greater (p < 0.001) in the mixed-water biofilter, and there were significant differences in extracellular polymeric substance (EPS) composition (protein: p < 0.001, carbohydrate: p < 0.05) between source water types. Few studies have examined surface water Mn removing biofilters, which may lead utilities to apply conclusions generated from groundwater Mn removing biofilters to surface water biofilters. However, this study suggests that despite similar treatment designs, influent water quality, and Mn removal performance, the composition of the EPS and microbial community within Mn removing biofilters can be significantly affected by source water type.
Water impact
There is increasing interest in biofiltration as a sustainable method to remove manganese from drinking water for surface/mixed water sources. In this study, comparison of full scale groundwater and mixed water manganese biofiltration determined that data derived from groundwater biofilters may not be applicable to surface/mixed water biofilters, and further investigation of surface/mixed water sources specifically is needed.
|
1. Introduction
1.1 Manganese as a contaminant in drinking water
Mn in drinking water has long been known to cause staining and discoloured water when present at concentrations as low as 20 μg L−1, and concern for the health risks associated with elevated levels of Mn in drinking water (>100 μg L−1) has grown in recent years.1–4 Health Canada has recently reduced the aesthetic objective for Mn in drinking water to 20 μg L−1 and has introduced a health-based maximum acceptable concentration of 120 μg L−1.5 The World Health Organization has also suggested a provisional health-based guideline for Mn of 80 μg L−1.6 Additionally, there is growing concern for the accumulation of Mn in distribution systems and storage tanks.5,6 Mn oxides can deposit on the interior surfaces of these structures forming increasingly larger aggregates.7 These aggregates can be resuspended and released back into drinking water, resulting in increased Mn concentrations at the tap.8
1.2 Biological mechanisms of manganese removal
Groundwater biofilters have been used extensively to treat Mn in Canada, China, Argentina, and Europe and have been well studied.9–14 Particulate Mn can be removed by filtration in which solid Mn particles attach to filter media or biofilm and are then removed by backwashing.15 Dissolved Mn can be oxidized to particulate Mn by biological or physico-chemical mechanisms. Mn can be precipitated biologically by manganese oxidizing bacteria (MnOB) which oxidize soluble Mn2+ to solid Mn-oxides however, the methods of biological Mn oxidation are not well characterized. MnOB may facilitate extracellular Mn oxidation through production of extracellular polymeric substances (EPS), which can include oxidative proteins and/or polysaccharides, or by secreting oxidation inducing by-products.16 Recent evidence has suggested that Mn oxidation may also occur through enzymatic intracellular oxidation, with Mn oxidation being used as a source of energy.17 During the early start-up of new Mn biofilters, biological mechanisms are predominantly responsible for Mn oxidation and removal. However, mature Mn biofilters often feature a combination of biological Mn oxidation and autocatalytic oxidation, in which Mn oxides already present on the surface of filter media or biofilm initiate further Mn oxidation.18,19
1.3 Manganese removal in groundwater and surface water biofilters
The majority of Mn in groundwater is often present as dissolved Mn due to anoxic conditions, while Mn in surface water is often a combination of dissolved and particulate Mn.16,20 Unlike groundwater sources, surface waters can experience seasonal fluctuations resulting in a range of water temperatures and concurrent changes to the proportion and distribution of dissolved and particulate Mn in the water column.16 The form of Mn, and the concentration and type of co-occurring contaminants, can have a significant impact on the performance of different treatment methods for Mn removal.16 Hoyland et al. (2014) observed that a decrease in Mn removal was concurrent with a reduction in water temperatures, to reflect winter conditions, in a bench-scale biofilter receiving simulated surface water.21 This was followed by a return to complete Mn removal when the influent water was increased to its original temperature. Hakes et al. (2016) also observed decreased Mn removal in a full-scale surface water Mn biofilter as influent water temperatures decreased during the winter.22
In addition to differences in water quality, there are many upstream treatment processes present in surface water treatment plants that could also impact the biofilter microbial community and biological Mn oxidation. Conventional groundwater treatment is often simple, consisting of aeration before and after filtration, and can effectively remove common groundwater contaminants such as Fe and Mn.23 Alternatively, conventional surface water treatment targets the removal of turbidity and organic matter and is more complex, consisting of coagulation, flocculation, sedimentation, filtration, and the application of oxidants.24 Thus, observations made in groundwater Mn biofiltration studies may not be applicable to surface water biofilters. To date, the majority of Mn biofiltration research has focused on groundwater treatment. Studies which have examined surface water treatment plants provided only a very limited analysis of the role of MnOB in Mn oxidation.21,25,26
Burger et al. (2008) examined four established groundwater Mn biofilters in the province of New Brunswick, Canada which were shown to exhibit robust and sustainable Mn removal.10 The authors concluded that the observed Mn oxidation was likely biological however, limitations in both the biological monitoring techniques and the knowledge of MnOB available to the water treatment community at the time restricted in-depth analysis. We revisited three of these sites (Memramcook, Shediac, and Woodstock), to assess their continued effectiveness after a further 10 years of service. We also built upon the previous study by providing an enhanced understanding of both the chemical and biological characteristics of the source water and filter media biofilm using improved analytical detection capabilities and advances in biological monitoring methods. Samples for biological analysis were taken from the raw water and filter effluent water and from the filter media before and after backwashing to examine microbial communities across the biofilter and to assess the impact of backwashing on the biomass and biofilm within each plant.
This study also aimed to investigate the impact that source water type has on the composition of biofilm within Mn removing biofilters. When sampled by Burger et al., (2008) all three biofilters treated groundwater.10 When sampled for this study however, one biofilter, Memramcook, treated a mixture of both groundwater and surface water. This change in source water provided a unique opportunity for investigating the influence of source water type on biological Mn oxidation because the same treatment design was used in all locations. This removes many of the additional variables present in other studies due to differences in upstream treatment of filter influent water which may make it difficult to isolate the impact of source water type specifically.
2. Materials and methods
2.1 Study area
Samples were collected from three Degremont Mangazur full-scale biofiltration plants in New Brunswick, Canada. The Woodstock treatment plant was built in 1999 and was the first biological Mn biofilter built in Canada.27 Media from this plant was used to seed the filter media of the Shediac biofilter, which was built in 2005. Media from the Shediac plant was used to seed the filter media of the Memramcook biofilter, which was built in 2006. All treatment plants utilize a circular filter (2.1 m in diameter) containing Biolite, a proprietary sand-based filter media, at a filter bed depth of 1.8 m. Woodstock operated their plant at the maximum filter flow capacity of 1.9 MLD, resulting in an empty bed contact time (EBCT) of 4 minutes. Memramcook and Shediac operated their plants at lower flow rates of 0.6 and 1.0 MLD, resulting EBCTs of 12 and 8 minutes respectively. Water is pumped into the treatment plant and aerated with compressed air before entering the filter tank. Water passes through the filter under pressure and filter effluent is disinfected with chlorine before entering the distribution system. All biofilters were backwashed with raw water and the backwashing procedures are detailed in Table S1.† The Woodstock and Shediac treatment plants feature two biofiltration tanks in parallel, while the Memramcook treatment plant has only one biofiltration tank.
The Woodstock treatment plant (W-GW) draws its water from two groundwater wells and the Shediac treatment plant (S-GW) is fed from eight groundwater wells. However, the Memramcook treatment plant (M-GW/SW) can draw water from two groundwater wells as well as two surface water reservoirs. During each sampling event the water source in operation at the Memramcook treatment plant was a blend of groundwater and surface water.
2.2 Sample collection
Raw water, filter influent water, filter effluent water, and filter media samples were collected at each location once a month from May to August 2017. Raw water samples were collected prior to aeration, filter influent samples were collected after aeration from the top of the tank, and filter effluent samples were collected immediately after biofiltration, before chlorine addition. Media samples were collected before and after backwash by draining the filter and collecting media from the top 5–10 cm of the filter by scooping it into a sterile sample bottle.
2.3 Water quality analysis
Temperature, oxidation–reduction potential (ORP), pH, dissolved oxygen (DO) and conductivity were measured on site immediately after sample collection. Samples were transported to Dalhousie University for measurement of total organic carbon (TOC), colour, UV absorbance at 254 nm, alkalinity, phosphate, ammonia, manganese, iron, and cellular ATP (cATP). Additional details are provided in the ESI.†
2.4 Filter media analysis
Filter media samples were transported to Dalhousie University for analysis of accumulated Mn and Fe, total ATP (tATP), EPS, heterotrophic plate counts (HPC), and extraction of genomic DNA from filter media before and after backwash. All analyses were initiated within 24 hours of sample collection. Additional details are provided in the ESI.†
2.5 DNA extraction and analysis
Genomic DNA samples were submitted to the Integrated Microbiome Resource at Dalhousie University for sequencing on an Illumina MiSeq using primers for the V6V8 region of the 16S rRNA gene.28 Raw 16S sequencing data was processed using QIIME 2 v_ 2019.7 following the Microbiome Helper workflow.29,30 Briefly, read quality was inspected with FastQC, primers were trimmed with the cutadapt QIIME 2 plugin, sequences were denoised with DADA2, and taxonomy was assigned using the SILVA database v_132.31–34 MAFFT and FastTree QIIME 2 plugins were used to construct phylogenetic trees.35,36 Raw sequencing were deposited in the National Centre for Biotechnology Information (NCBI) Sequence Read Archive (SRA) under the BioProject accession number PRJNA897004. Statistical analysis of microbial sequencing data was conducted in R. Rarefaction curves were generated in R with the ranacapa package (v0.1.0) to confirm sufficient sampling depth.38 The following samples had insufficient read depth and were not included in further analyses: W-GW June raw water and effluent water, S-GW May to July raw water and June effluent water, M-GW/SW May and June raw water, W-GW May to July pre and post backwash media, all S-GW media samples, and M-GW/SW June pre and post backwash media. The low read depth in these samples may be due to insufficient biological material and/or inhibition to the polymerase chain reaction caused by high concentrations of Fe and humic substances on the filter media.39 The remaining samples were subsequently rarefied to a depth of 1197 amplicon sequence variants (ASVs) per sample for diversity analysis. Alpha diversity was analyzed with Shannon's Index for diversity and Simpson's Index for evenness using the phyloseq package (v1.30.0).40 Group comparisons of diversity and evenness were performed using a pairwise Wilcoxon rank sum test with a Benjamini–Hochberg correction for FDR. Beta diversity was analyzed using a Bray–Curtis dissimilarity matrix and plotted using principal coordinates analysis (PCoA).40 Group comparisons were made using the adonis test in the vegan package (v2.5-6).41 The proportion of total features shared between sample groups and the average abundance of those shared features was determined using the ampvis2 (v2.6.4) package.42 Figures were generated using the tidyverse (v1.3.0), ggthemes (v4.2.0), and cowplot (v1.1.0) packages.43–45
2.6 Statistical analysis
Statistical analysis of filter media and raw, influent, and effluent water was performed in R using Wilcoxon rank sum tests, with Benjamini–Hochberg correction for false discovery rate (FDR) for comparison of independent groups.37
3. Results and discussion
3.1 Chemical characterization of water quality and contaminant removal across filters
During this study, raw water, filter influent, and filter effluent samples were collected from the M-GW/SW, S-GW, and W-GW water treatment plants over the summer of 2017. Additional measurements taken during this study but not the previous study included temperature, DO, PO4, dissolved Mn, and dissolved Fe in both the raw water and filter effluent (Table 1). There was little change in the majority of the filter influent and effluent water quality parameters at each water treatment plant compared to the study conducted by Burger et al. (2008) (Table 1).10 All three treatment plants achieved approximately 100% Mn removal and Mn effluent concentrations well below current drinking water guidelines (Fig. 1A).5 This is consistent with the findings of Burger et al. (2008), indicating highly sustainable, long-lasting, and robust Mn removal over a ten-year period.10 The concentration of Fe, both total and dissolved, was low in the raw water of M-GW/SW and W-GW (Table 1). The Fe concentration in the S-GW raw water was much greater and present mostly as particulate Fe. The average Fe removal efficiencies were greater than 78%, 98%, and 87% for M-GW/SW, S-GW, and W-GW respectively, and all three treatment plants achieved Fe effluent concentrations to meet current drinking water guidelines.5 The average raw water total Fe concentration and the accumulation of Fe on the surface of filter media were both greater in the S-GW treatment plant than in the M-GW/SW or W-GW treatment plants. Although Burger et al. (2008) did measure NH3 and Fe in the raw and effluent water at each plant, only the NH3 and Fe concentrations in the raw water at the Shediac treatment plant were above the detection limits of 60 μg L−1 and 200 μg L−1 respectively. This study employed improved detection limits of 10 μg L−1 and 7 μg L−1 respectively, which allowed for the detection of NH3 and Fe in the raw water of all three treatment plants.10
Table 1 Average (±S.D.) water quality parameters for raw, filter influent, and filter effluent water from the M-GW/SW, S-GW, and W-GW biofiltration plants from this study and the work of Burger et al. (2008)10
|
Sample location |
M-GW/SW |
S-GW |
W-GW |
This study |
Burger et al. 2008 (ref. 10) |
This study |
Burger et al. 2008 (ref. 10) |
This study |
Burger et al. 2008 (ref. 10) |
Value was above the detection limit for only one sampling event.
|
pH |
Raw water |
7.08 ± 0.52 |
6.46 |
7.05 ± 0.52 |
7.5 |
7.19 ± 0.34 |
7.39 |
Influent water |
6.94 ± 0.44 |
— |
6.95 ± 0.17 |
— |
6.81 ± 0.47 |
— |
Effluent water |
7.02 ± 0.26 |
6.46 |
7.06 ± 0.37 |
7.63 |
6.79 ± 0.25 |
7.33 |
ORP (mV) |
Raw water |
27 ± 108 |
343 |
136 ± 189 |
338 |
233 ± 147 |
368 |
Influent water |
72 ± 226 |
— |
126 ± 198 |
— |
238 ± 149 |
— |
Effluent water |
208 ± 139 |
370 |
245 ± 174 |
332 |
313 ± 118 |
480 |
Conductivity (μS cm−1) |
Raw water |
274 ± 8 |
170 |
421 ± 16 |
361 |
395 ± 8 |
364 |
Influent water |
272 ± 6 |
— |
425 ± 13 |
— |
394 ± 10 |
— |
Effluent water |
259 ± 18 |
170 |
416 ± 17 |
364 |
391 ± 10 |
356 |
Temperature (°C) |
Raw water |
9.0 ± 0.3 |
— |
9.8 ± 0.5 |
— |
10.3 ± 0.9 |
— |
Influent water |
9.7 ± 1.9 |
— |
11.3 ± 1.4 |
— |
11.5 ± 1.8 |
— |
Effluent water |
9.2 ± 0.9 |
— |
10.3 ± 0.8 |
— |
10.2 ± 0.8 |
— |
DO (mg L−1) |
Raw water |
5.1 ± 2.0 |
— |
7.2 ± 1.7 |
— |
6.6 ± 2.1 |
— |
Influent water |
5.6 ± 1.4 |
— |
5.9 ± 1.5 |
— |
8.7 ± 1.6 |
— |
Effluent water |
5.4 ± 2.6 |
— |
7.7 ± 1.7 |
— |
9.7 ± 2.8 |
— |
Alkalinity (mg L−1) |
Raw water |
113 ± 8 |
14 |
134 ± 5 |
30 |
143 ± 3 |
37 |
Effluent water |
107 ± 4 |
14 |
127 ± 4 |
30 |
139 ± 3 |
36 |
TOC (mg L−1) |
Raw water |
1.8 ± 2.1 |
1 |
2.2 ± 2.9 |
0.9 |
1.5 ± 0.1 |
1.5 |
Effluent water |
0.7 ± 0.3 |
0.9 |
1.3 ± 1.5 |
0.9 |
1.5 ± 0.1 |
1.7 |
UV 254 |
Raw water |
0.01 ± 0.0 |
0.01 |
0.02 ± 0.01 |
0.01 |
0.03 ± 0.0 |
0.25 |
Effluent water |
0.01 ± 0.0 |
0.01 |
0.0 ± 0.0 |
0.01 |
0.03 ± 0.0 |
0.03 |
Colour (NTU) |
Raw water |
3 ± 5 |
0 |
22 ± 7 |
0 |
5 ± 5 |
1 |
Effluent water |
3 ± 3 |
0 |
1 ± 3 |
0 |
6 ± 8 |
0 |
NH3 (mg L−1) |
Raw water |
0.04 ± 0.02 |
<0.06 |
0.03 ± 0.01 |
0.13 |
0.04 ± 0.01 |
<0.06 |
Effluent water |
0.02 ± 0.02 |
<0.06 |
0.01 ± 0.01 |
<0.06 |
0.04 ± 0.03 |
<0.06 |
PO4 (mg L−1) |
Raw water |
0.05 ± 0.01 |
— |
0.15 ± 0.08 |
— |
0.46 ± 0.68 |
— |
Effluent water |
0.07 ± 0.06 |
— |
0.11 ± 0.06 |
— |
0.08 ± 0.06 |
— |
cATP (pg mL−1) |
Raw water |
15 ± 12 |
— |
6 ± 17 |
— |
4 ± 1 |
— |
Effluent water |
137 ± 103 |
— |
1 ± 0 |
— |
4 ± 1 |
— |
Dissolved Mn (μg L−1) |
Raw water |
419a |
— |
410 ± 430 |
— |
860 ± 860 |
— |
Effluent water |
3a |
— |
<0.8 |
— |
0.9 ± 0.9 |
— |
Total Mn (μg L−1) |
Raw water |
370 ± 40 |
860 |
910 ± 100 |
860 |
950 ± 250 |
1390 |
Effluent water |
8.6 ± 4.9 |
<30 |
<0.8 |
<30 |
1.3 ± 1.3 |
<30 |
Dissolved Fe (μg L−1) |
Raw water |
35a |
— |
213a |
— |
<7 |
— |
Effluent water |
<7 |
— |
<7 |
— |
<7 |
— |
Total Fe (μg L−1) |
Raw water |
35 ± 12 |
<200 |
2070 ± 3040 |
260 |
130a |
<200 |
Effluent water |
<7 |
<200 |
<7 |
<200 |
<7 |
<200 |
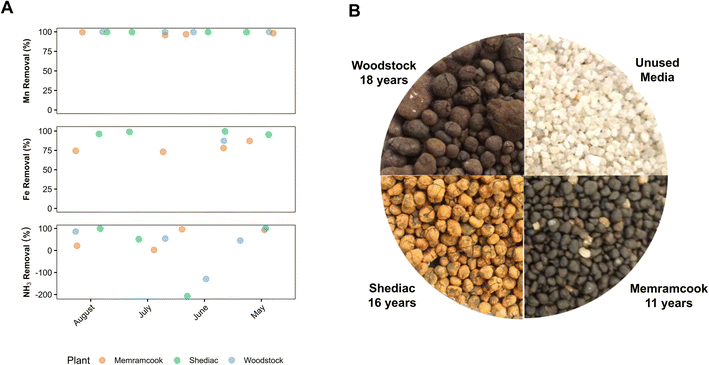 |
| Fig. 1 (A) Time series data of total Mn removal, total Fe removal, and NH3 removal across the M-GW/SW, S-GW, and W-GW water treatment plants. (B) Unused Biolite media and used media collected from the top of the biofilters at the M-GW/SW, S-GW, and W-GW treatment plants. The number of years each biofilter has been in service is indicated. | |
The average size of the filter media granules from the M-GW/SW, S-GW, and W-GW treatment plants were 1.8 mm ± 0.2 mm, 2.1 mm ± 0.3 mm, and 2.0 mm ± 0.7 mm respectively. The size of the filter media at all three treatment plants was observed to increase relative to the unused media due to the continued accumulation of Mn and Fe oxide coatings on the surface of filter media (Fig. 1B, Table S2†). The accumulation of Mn on the surface of filter media was greater in the W-GW biofilter (76 mg g−1 dw) than in the M-GW/SW or S-GW biofilters (55 mg g−1 dw for both), while the accumulation of Fe was greater in the S-GW treatment plant (52 mg g−1 dw) than the M-GW/SW or W-GW treatment plants (18 mg g−1 dw and 0.4 mg g−1 dw). A previous study by Breda et al. (2016) on the start-up of new Mn and Fe removing biofilters also observed that Mn oxides were not removed by backwashing but instead continued to accumulate on the surface of biofilter media, as is the case in these filters, and work by Bruins et al. (2015) confirmed formation of biogenic Mn oxides.18,46 Although biological oxidation has been shown to play a significant role in the start-up of new Mn biofilters, Mn oxidation in mature biofilters can be a combination of both biological and physico-chemical mechanisms.14,23 Physico-chemical mechanisms can include auto-catalytic oxidation of dissolved Mn(II) by existing manganese oxides however, in the absence of a strong oxidant these sites become exhausted which prevents further auto-catalytic oxidation.16 Biologically produced Mn oxides, which continue to accumulate on the surface of biofilter media, may regenerate the sites necessary for auto-catalytic oxidation within these treatment plants.14
3.2 Biological characterization of source water quality and filter media biofilm
Burger et al. (2008) identified only one potential MnOB, a member of the genus Leptothrix, present in the filter media of the M-GW/SW water treatment plant.10 Due to advances in biological monitoring techniques and the identification of a greater number of known MnOB, this study was able to identify not only Leptothrix, but 21 other genera which contain potential MnOB in the raw and filter effluent water from the M-GW/SW, S-GW, and W-GW treatment plants (Fig. 2A).9,13,47–59Leptothrix was not detected in the filter media biofilm of either the M-GW/SW or W-GW treatment plants, however, 11 other genera which contain potential MnOB were identified (Fig. 2B).9,13,47,49,51,52,54,57,58 Several potential MnOB were present in the filter media communities of both the M-GW/SW and W-GW water treatment plants including Crenothrix, Hyphomicrobium, Legionella, Reyranella, and Sphingomonas.9,13,54,57 It is difficult to know the composition of the filter media community in the S-GW water treatment plant due to the insufficient read depth of these samples. However, some insight may be gained by looking at the microbial community of the filter effluent water. Several potential MnOB were present in all three filter effluent samples, but not present in raw water samples, including genera present in both the M-GW/SW and W-GW water treatment plants: Bosea, Brevundimonas, Rhodococcus, Sphingopyxis, and Flavobacterium.13,49,51,52,57 These genera may be enriched in the S-GW filter media community and enter the filter effluent after becoming dislodged by hydraulic forces as water flows through the filter. This trend was observed in the M-GW/SW treatment plant, where Methylomonas was absent from all raw water samples but abundant in all filter effluent and filter media samples, and in the W-GW treatment plant, where Candidatus nitrotoga and Methyloglobulus were absent from, or present at very low abundance (<0.1%) in, all raw water samples, but abundant in all filter effluent and filter media samples.
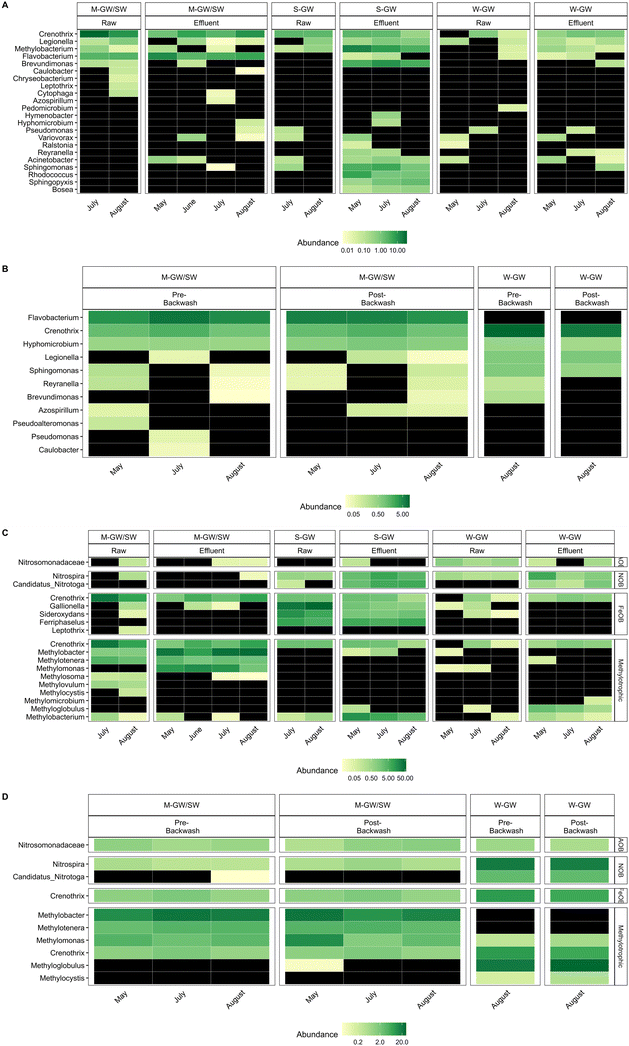 |
| Fig. 2 Heatmap of the relative abundances of genera known to contain MnOB in (A) the raw water and filter effluent and (B) pre-backwash and post-backwash filter media biofilm of the M-GW/SW, S-GW, and W-GW water treatment plants. Heatmap of the relative abundances of a family known to contain AOB and genera known to contain NOB, FeOB, methylotrophic and methanotrophic bacteria in (C) the raw water and filter effluent and (D) pre-backwash and post-backwash filter media biofilm of the M-GW/SW, S-GW, and W-GW water treatment plants. | |
The microbial community was further investigated to look beyond MnOB, and Mn removal performance, and examine the impact that differences in raw water chemistry can have on community development as a whole. In addition to MnOB, we also detected the presence of genera known to include Fe oxidizing bacteria (FeOB) and nitrite oxidizing bacteria (NOB), and a family known to include ammonia oxidizing bacteria (AOB) in the raw water, filter effluent water, and filter media biofilm of the M-GW/SW, S-GW, and W-GW treatment plants (Fig. 2C and D).59–66 The bacterial community within the raw water and filter effluent of the S-GW treatment plant was dominated by the high abundance of several potential FeOB genera including Gallionella, Sideroxydans, and Ferriphaselus.60,61,64 These same genera were also present in the raw water and filter effluent of the M-GW/SW and W-GW treatment plants, but at much lower abundances, and they did not make up a significant portion of the bacterial community. Correspondingly, Fe was present at a much greater concentration in the raw water of the S-GW treatment plant than either the M-GW/SW or W-GW water treatment plants (Table 1). The majority of Fe in the influent water of all three filters was present as particulate Fe and thus there was likely little biological Fe oxidation within the filters. Rather, we suggest the high abundance of FeOB in the S-GW water may be due their ability to tolerate high Fe oxide environments.
The concentration of NH3 in the raw water of each plant was low (Table 1), however some reduction in NH3 was observed across the filters (Fig. 1A), which is consistent with NH3 oxidation and the presence of AOB in the filter media biofilm.65 Although neither NO2− nor NO3− were measured during this study, the abundance of NOB, particularly in the W-GW biofilm, suggests that NO2− oxidation could also have taken place within the filters.63,66 Co-removal of Mn and NH3 has been observed extensively in groundwater biofilters which include Nitrospira and Candidatus Nitrotoga, the two NOB genera observed in this study.58,67,68 Surface water filters which support the growth of Nitrospira have also been shown to remove both Mn and NH3.26
The water and filter media biofilm microbial communities of the M-GW/SW water treatment plant were dominated by a high abundance and diversity of methylotrophic and methanotrophic bacteria (Fig. 2C and D).62,69–72 Methylotrophs are bacteria that can use single carbon compounds, or multi-carbon compounds that contain no carbon–carbon bonds, as an energy source. Methanotrophs are a subset of methylotrophs which can only use single carbon compounds as energy sources, most commonly methane. Several of these methylotrophic and methanotrophic genera were either absent from or present at very low abundance in the S-GW and W-GW water treatment plants, but have been observed in groundwater and surface water Mn removing biofilters previously.12,13,73–76 Two methanotrophs, Methyloglobulus and Methylocystis, were present in the filter media biofilm of the W-GW treatment plant, but not the M-GW/SW treatment plant.69,70Methyloglobulus has previously been observed in groundwater Mn removing biofilters.75,76 Although both the M-GW/SW and W-GW microbial communities contain methanotrophic genera, there is a distinct phylogenetic and metabolic divergence between the two treatment plants. Methyloglobulus is a type Ia methanotroph, which is phylogenetically distant from the M-GW/SW methanotrophs which are all type Ib.62,69 Additionally, Methylocystis is a type II methanotroph, which uses a different metabolic pathway to consume methane than either type Ia or type Ib methanotrophs.70 There was no significant difference in raw water TOC between the three water treatment plants. However, the measurement of TOC alone does not account for potential differences in the forms of carbon that can be used as a nutrient source, including assimilable organic carbon and biodegradable dissolved organic carbon.77 Furthermore, this study used a non-purgeable method to measure organic carbon, which involves sparging air through the water sample to remove volatile compounds.78 Thus, the TOC values reported here would not account for any potential differences in methane concentration between the three water treatment plants. The high abundance and diversity of methylotrophic and methanotrophic bacteria in the M-GW/SW water treatment plant, coupled with the phylogenetic and metabolic divergence between methanotrophs present in both the M-GW/SW and W-GW treatment plant, suggests that although the concentration of TOC was not different, the composition of carbon molecules within the water sources was different. Specifically, the abundance of reduced carbon compounds or compounds with no carbon–carbon bonds, particularly methane, was greater in the raw water of the M-GW/SW treatment plant than in the W-GW or S-GW treatment plants.
One of the more intriguing findings in this study is the prevalence of Crenothrix at high abundance in all three water treatment plants (Fig. 2C and D). This genus is unique in that it is methanotrophic, but also contains known FeOB and MnOB.62 It has been observed in groundwater filters which also contain other methanotrophic bacteria and found in groundwater filters which exhibit co-removal of Fe, Mn, and NH3.12,13,67,74 The pervasiveness of Crenothrix in this study may be due to its ability to exploit multiple ecological niches, acting as a FeOB in the S-GW water treatment plant, a methanotroph in the M-GW/SW water treatment plant, and a MnOB in all three water treatment plants.
3.3 Impact of backwashing on filter media biofilm
The filter media of each treatment plant was sampled at the end of a filter run immediately before backwashing, and then sampled again immediately after backwashing, to assess the impact of backwashing on the biofilms within each plant. The biofilms of each plant are composed of biomass, measured by quantifying tATP, and HPC, and an extracellular matrix, measured by quantifying proteins and polysaccharides. In the M-GW/SW biofilter the average tATP and concentration of polysaccharides in the EPS were reduced after backwashing by 50% and 89% respectively, indicating a loss of biomass and one component of the EPS. In the S-GW biofilter however, the average tATP and HPC were reduced after backwashing by 81% and 90% respectively, indicating a loss of biomass but not EPS (Fig. 3A–C). Little change was observed in either biomass or EPS during backwashing at the W-GW treatment plant. The concentration of protein in the EPS was consistent before and after backwashing at all three treatment plants (Fig. 3D). The backwashing protocols used at all three biofiltration plants were similar, except for the water rinse cycle (Table S1†). The water rinse cycle at the M-GW/SW biofilter was more than 6 times longer and more than 3.5 times longer than the same cycle at the S-GW and W-GW biofilters respectively.
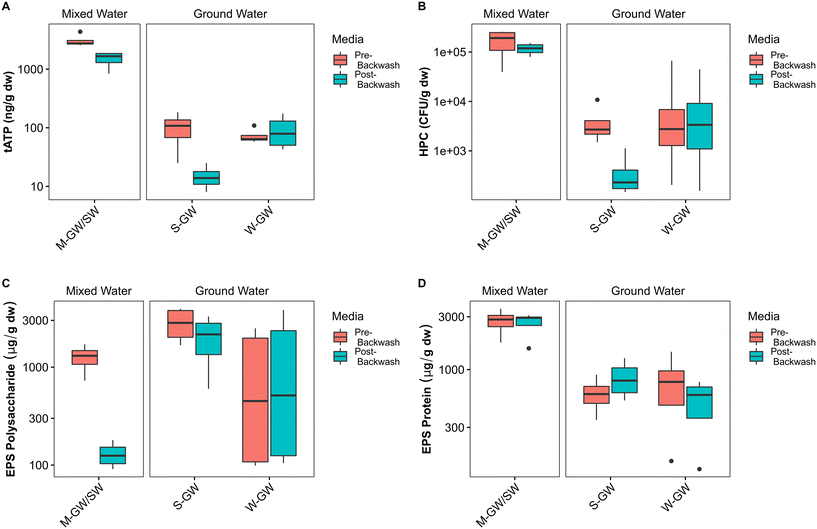 |
| Fig. 3 Pre-backwash and post-backwash (A) tATP, (B) HPC, (C) EPS polysaccharide, and (D) EPS protein concentrations at the M-GW/SW, S-GW, and W-GW treatment plants. Data are presented on a log 10 scale. The horizontal line within the box indicates the median, the upper and lower bounds of the box indicate the third and first quartiles, respectively, and the whiskers extend to the largest value no greater than 1.5 times the interquartile range. Values beyond the whiskers are outliers. | |
At the M-GW/SW treatment plant the loss of biomass was observed during normal filter operation as well, where the average cATP increased by more than 1000% across the biofilter (Fig. 4A). The concentration of cATP in the raw, filter influent, and filter effluent water of surface water biofilters commonly range from 100 to 101 pg mL−1.26,79–81 This is consistent with the average raw water cATP concentration at the M-GW/SW biofilter (15 pg mL−1 ± 12 pg mL−1), but the average filter effluent cATP concentration was an order of magnitude greater (140 pg mL−1 ± 100 pg mL−1). Additionally, the proportion of total features (i.e., unique DNA sequences) shared between the pre-backwash community and the filter effluent community, and the average relative abundance of those features, were both greater than the average relative abundance and proportion of total features shared by the raw water and filter effluent communities or the raw water and pre-backwash communities (Fig. 4B). However, little change in cATP was observed across the S-GW or W-GW biofilters, and the proportion of total features shared between the filter effluent and pre-backwash communities at the W-GW treatment plant, and the relative abundance of those features, were low. These data suggest that the relatively gentle hydraulic effects of normal filter operation released a greater proportion of microorganisms from the filter media of the M-GW/SW biofilter than the S-GW or W-GW biofilters. The biomass present in the M-GW/SW biofilter is more labile, and thus more susceptible to separation from the filter media under the increased hydraulic effects of backwashing. This, coupled with the extended water rinse cycle in use at the M-GW/SW treatment plant relative to the S-GW and W-GW treatment plants, may have flushed the detached biomass and EPS from the filter, resulting in the observed reductions in tATP, HPC, and EPS polysaccharides after backwashing. Despite this release of biomass, the proportion of features shared between the pre-backwash and post-backwash communities, and the average abundance of those features, remained high. The treatment plant was also able to maintain Mn removal.
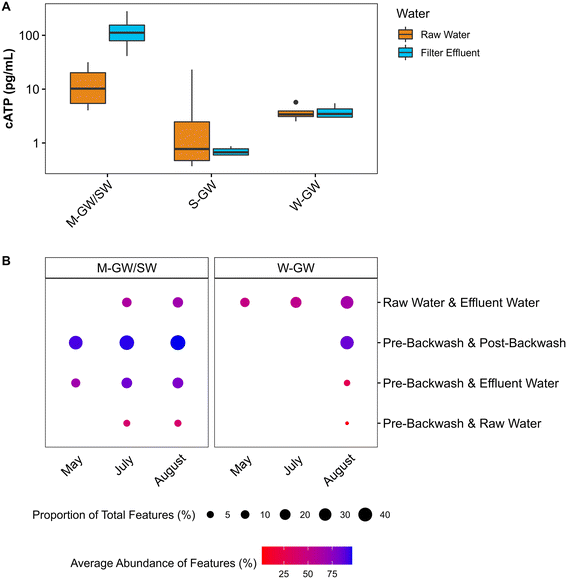 |
| Fig. 4 (A) Raw and filter effluent water cATP concentrations at the M-GW/SW, S-GW, and W-GW treatment plants. Data are presented on a log 10 scale. The horizontal line within the box indicates the median, the upper and lower bounds of the box indicate the third and first quartiles, respectively, and the whiskers extend to the largest value no greater than 1.5 times the interquartile range. Values beyond the whiskers are outliers. (B) Proportion of total features shared between sample groups and the average abundance of those shared features. | |
3.4 Impact of source water type on biofiltration
Few studies examine Mn removal in surface water biofilters, and even fewer directly compare Mn removal in groundwater and surface water biofilters. Although the M-GW/SW water treatment plant does not receive surface water exclusively, the similarities in water treatment design shared between the M-GW/SW, S-GW, and W-GW water treatment plants provide a valuable opportunity to compare the impact of source water type on Mn removing biofilters.
There were few apparent differences in the chemical composition of filter influent water across the three water treatment plants, however conductivity (p < 0.01), alkalinity (p < 0.01), dissolved Mn (p < 0.05), and total Mn (p < 0.01) were all significantly greater in the groundwater treatment plants (S-GW and W-GW) than the mixed-water treatment plant (M-GW/SW) (Fig. 5A–D). Typically, the concentrations of salts and dissolved metals are higher in groundwaters because CO2-bearing and often anoxic groundwater is surrounded by rock and soil on a time scale long enough for dissolution to occur.20 Surface waters often have higher concentrations of DO and have greater microbial activity, which further decreases the concentrations of these constituents. Therefore, alkalinity, conductivity, and the concentration of dissolved Mn is regularly greater in groundwaters compared to surface waters. A recent study comparing groundwater and surface water biofilters by Hu et al. (2020) also observed a greater concentration of total Mn in groundwater compared to surface water.76
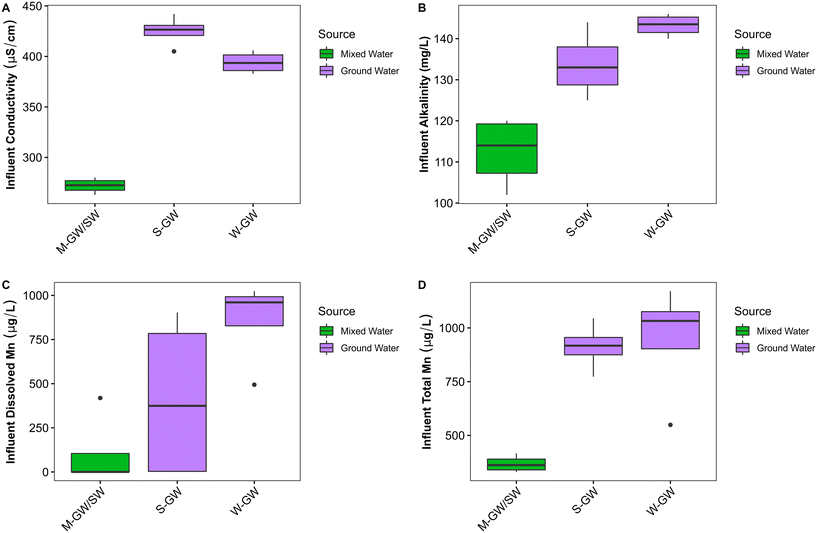 |
| Fig. 5 Filter influent water quality for groundwater and mixed-water treatment plants. (A) Conductivity (p < 0.01), (B) alkalinity (p < 0.01), (C) dissolved Mn (p < 0.05), and (D) total Mn (p < 0.01) were significantly greater in the raw water of groundwater treatment plants than the mixed-water treatment plant. Wilcoxon rank sum test with a Benjamini–Hochberg correction. The horizontal line within the box indicates the median, the upper and lower bounds of the box indicate the third and first quartiles, respectively, and the whiskers extend to the largest value no greater than 1.5 times the interquartile range. Values beyond the whiskers are outliers. | |
There were several differences in the biological composition of the raw water between the groundwater treatment plants and the mixed-water treatment plant. The concentration of cATP was significantly greater in the raw water of the mixed-water treatment plant than the groundwater treatment plants (p < 0.05), suggesting greater biological activity within the mixed-water source (Fig. 4A). This is supported by data from Hu et al. (2020) which found the live cell count was greater in surface water treatment plants than in groundwater treatment plants.76 The high abundance and diversity of methylotrophic and methanotrophic bacteria in the raw water of the mixed-water treatment plant but not the groundwater treatment plants indicate the mixed water source may have a greater concentration of methane and other single carbon compounds. A study by Pharand et al. (2014) suggested that the concentration and composition of biodegradable organic matter can have a significant impact on cATP.82 Thus, the differences in biological activity observed between water sources may be influenced by differences in biologically available carbon compounds. The richness (p < 0.05), diversity (p < 0.05), and evenness (p < 0.05) of the mixed-water community was significantly lower than the groundwater community, and the mixed-water community as a whole was significantly different from the groundwater community (p < 0.001) (Fig. S1A and B†). A previous study by Hu et al. (2020) also found a significant difference between the filter media communities of groundwater and surface water filters.76 The groundwater community examined by Hu et al. (2020) was heavily enriched with the NOB Nitrospira, which is also present at greater abundance in the groundwater communities than in the mixed-water communities of this study.76
Biological differences between the mixed-water source and groundwater sources were also identified in the filter media biofilm. Both measures of active biomass within the filters, tATP (p < 0.001) and HPC (p < 0.001), were significantly greater in the mixed-water biofilm than in the groundwater biofilm (Fig. 3A and B). Biomass growth is limited by the concentration of available nutrients passing through the filter, including carbon, nitrogen, and phosphorus. While there was no significant difference between TOC, NH3, or PO4 in the raw water of the groundwater plants and mixed-water plant, the high abundance and diversity of methanotrophic and methylotrophic bacteria in the M-GW/SW filter media biofilm suggests the concentration of single carbon-containing compounds within the raw water is much greater in the mixed-water treatment plant than in the groundwater treatment plants. This variation may contribute to the greater biomass observed in the mixed-water filter media biofilm. The EPS composition also differed by source water type. The polysaccharide fraction of the EPS (p < 0.05) was significantly greater in the groundwater biofilm, and the protein fraction of the EPS (p < 0.001) was significantly greater in the mixed-water biofilm (Fig. 5C and D). Genera known to produce polysaccharides in biofilm EPS include Pseudomonas, Staphylococcus, Bacillus, and Vibrio.83Pseudomonas was present in a single sample of each groundwater source and a single sample of the mixed-water biofilm, and Streptococcus was also present in a single sample of the of the mixed-water biofilm. However, the abundance of both genera was very low in all samples (0.01–0.1%) and it is unlikely they were responsible for the majority of polysaccharides present in the biofilm.
A limited number of studies have examined Mn removing biofilters using surface water sources, which may lead utilities to apply conclusions generated from groundwater Mn removing biofilters to surface water biofiltration plants. However, results presented in this study suggest that data from groundwater biofilters may not be applicable to surface or mixed-water biofilters. Little difference was observed between the water chemistry of the groundwater and mixed-water biofilters using routinely measured water quality parameters, and yet the amount of active biomass, the EPS composition, and the microbial communities of the source water were all significantly different between the two source water types. Furthermore, this dissimilarity was apparent when only a portion of the source water for the M-GW/SW treatment plant was derived from surface water. It is likely that an even greater divergence between groundwater treatment plants and treatment plants which receive surface water exclusively. The additional upstream treatment methods used in surface water treatment plants, such as coagulation and sedimentation, could also influence water quality, biofilter community structure, and sustained, effective biological Mn removal. Given the limited data set and the combination of both ground and surface water at the M-GW/SW treatment plant, caution should be taken when trying to extrapolate these conclusions to ground and surface water treatment plants in general. However, this work highlights the impact source water type can have on the filter media biofilm of Mn removing biofilters and the need for further studies which examine surface water or mixed water plants specifically.
4. Conclusions
• A return to the Memramcook, Shediac, and Woodstock treatment plants after a further 10 years of service confirmed that these biofilters continued to achieve approximately 100% Mn removal, indicating biofiltration is a highly sustainable, long-lasting, and robust treatment option for Mn.
• Previous work by Burger et al., (2008)10 identified one MnOB in one of the three treatment plants and concluded that additional MnOB were likely present in the biofilters. The current study was able to confirm this hypothesis through the identification of 21 and 11 additional potential MnOB, in the raw water and filter media biofilms respectively, across all three treatment plants, thus providing a more complete picture of biological Mn oxidation within the biofilters.
• Removal of Fe and NH3 was also confirmed for all three biofilters and bacteria capable of Fe, NH3, NO2−, and methane oxidation were identified in the raw water and filter media biofilms.
• The more labile biofilm present at the Memramcook treatment plant, including both biomass and EPS, was susceptible to removal from the biofilter during backwashing and routine biofilter operation.
• Little difference was observed between the influent water chemistry of the groundwater and mixed-water biofilters using routinely measured water quality parameters (temperature, ORP, pH, DO, TOC, colour, UV 254). However, the amount of active biomass and the microbial communities of the source water, as well as the amount of active biomass and the EPS composition of the filter media biofilms, were all significantly different between the two source water types.
• This work highlights the impact source water type can have on the filter media biofilm of Mn removing biofilters and the need for further studies which examine surface water or mixed-water Mn removing biofilters specifically.
Conflicts of interest
There are no conflicts of interest to declare.
Acknowledgements
This work was funded through the NSERC/Halifax Water Industrial Research Chair program (IRCPJ: 349838-16), an NSERC Discovery Grant (RGPIN-2018-03780), and an NSERC CGS-D scholarship. The authors would like to acknowledge the staff and water professionals at the water treatment plants in the village of Memramcook and the towns of Shediac and Woodstock, New Brunswick, Canada. The authors would also like to acknowledge the technical assistance of Meaghan MacGillivray and Jessica Campbell at the Centre for Water Resources Studies at Dalhousie University.
References
-
P. M. Kohl and S. J. Medlar, Occurrence of manganese in drinking water and manganese control, Project #2863, AwwaRF, Denver, 2006 Search PubMed.
- M. Sidoryk-Wegrzynowicz and M. Aschner, Manganese toxicity in the central nervous system: the glutamine/glutamate-γ-aminobutyric acid cycle, J. Intern. Med., 2013, 273(5), 466–477 CrossRef CAS.
- P. Chen, S. Chakraborty, S. Mukhopadhyay, E. Lee, M. Paoliello, A. Bowman and M. Aschner, Manganese homeostasis in the nervous system, J. Neurochem., 2015, 134(4), 601–610 CrossRef CAS PubMed.
- L.-A. Dion, M. Bouchard, S. Sauve, B. Barbeau, A. Tucholka, P. Major, G. Gilbert, D. Mergler and D. Saint-Amour, MRI pallidal signal in children exposed to manganese in drinking water, Neurotoxicology, 2016, 53, 124–131 CrossRef CAS PubMed.
-
Health Canada, Guidelines for Canadian drinking water quality: Guideline technical document - manganese, Health Canada, Ottawa, 2019 Search PubMed.
-
World Health Organization (WHO), Manganese in drinking-water: Background document for development of WHO guidelines for drinking-water quality, document number: WHO/HEP/ECH/WSH/2021.5, WHO, Geneva, 2020 Search PubMed.
- L. Sly, M. Hodgkinson and V. Arunpairojana, Deposition of manganese in a drinking water distribution system, Appl. Environ. Microbiol., 1990, 56(3), 628–639 CrossRef CAS.
- G. Li, X. Ma, R. Chen, Y. Yu, H. Tao and B. Shi, Field studies of manganese deposition and release in drinking water distribution systems: Insight into deposit control, Water Res., 2019, 163, 114897 CrossRef CAS PubMed.
- P. Mouchet, From conventional to biological removal of iron and manganese in France, J. - Am. Water Works Assoc., 1992, 84(4), 158–167 CrossRef CAS.
- M. S. Burger, C. Krentz, S. Mercer and G. Gagnon, Manganese removal and occurrence of manganese oxidizing bacteria in full-scale biofilters, J. Water Supply: Res. Technol.--AQUA, 2008, 57(5), 351–359 CrossRef CAS.
- S. Dangeti, B. Roshani, B. Rindall, J. McBeth and W. Chang, Biofiltration field study for cold Fe(II)- and Mn(II)-rich groundwater: Accelerated Mn(II) removal kinetics and cold-adapted Mn(II)-oxidizing microbial populations, Water Qual. Res. J., 2017, 52(4), 229–242 CrossRef CAS.
- Q. Cheng, L. Nengzi, L. Bao, Y. Huang, S. Liu, X. Cheng, B. Li and J. Zhang, Distribution and genetic diversity of microbial populations in the pilot-scale biofilter for simultaneous removal of ammonia, iron, and manganese from real groundwater, Chemosphere, 2017, 182, 450–457 CrossRef CAS PubMed.
- A. Piazza, L. Casalini, V. Pacini, G. Sanguinetti, J. Ottado and N. Gottig, Environmental bacteria involved in manganese(II) oxidation and removal from groundwater, Front. Microbiol., 2019, 10, 119 CrossRef.
- I. Breda, L. Ramsay, D. Soborg, R. Dimitrova and P. Roslev, Manganese removal processes at 10 groundwater fed full-scale drinking water treatment plants, Water Qual. Res. J., 2019, 54(4), 326–337 CrossRef CAS.
-
W. R. Knocke, R. Hungate and S. Occiano, Removal of soluble manganese from water by oxide-coated filter media, Project #306, AwwaRF, Denver, 1990 Search PubMed.
- J. Tobiason, A. Bazilio, J. Goodwill, X. Mai and C. Nguyen, Manganese removal from drinking water sources, Curr. Pollut. Rep., 2016, 2, 168–177 CrossRef CAS.
- H. Yu and J. Leadbetter, Bacterial chemolithoautotrophy via manganese oxidation, Nature, 2020, 583, 453–458 CrossRef CAS.
- J. Bruins, B. Petrusevski, Y. Slokar, K. Huysman, K. Joris, J. Kruithof and M. Kennedy, Biological and physico-chemical formation of Birnessite during the ripening of manganese removal filters, Water Res., 2015, 69, 154–161 CrossRef CAS.
- L. Ramsay, I. Breda and D. Soborg, Comprehensive analysis of the start-up period of a full-scale drinking water biofilter provides guidance for optimization, Drinking Water Eng. Sci., 2018, 11, 87–100 CrossRef CAS.
-
V. Snoeyink and D. Jenkins, in Water Chemistry, John Wiley and Sons, New York, 1980 Search PubMed.
- V. Hoyland, W. Knocke, J. Falkinham, A. Pruden and G. Singh, Effect of drinking water treatment process parameters on biological removal of manganese from surface water, Water Res., 2014, 66, 31–39 CrossRef CAS PubMed.
-
L. Hakes, A. Evans, C. Russell, B. Knocke, M. Ziv-El, S. Keithley and M. Kirisits, Troubleshooting reduced biofilter manganese removal following winter shutdowns, in Proceedings of the Annual AWWA Water Quality Technology Conference, Indianapolis, 2016 Search PubMed.
- J. Bruins, D. Vries, B. Petrusevski, Y. Slokar and M. Kennedy, Assessment of manganese removal from over 100 groundwater treatment plants, J. Water Supply: Res. Technol.--AQUA, 2014, 63(4), 268–280 CrossRef CAS.
-
M. Benjamin and D. Lawler, in Water quality engineering: Physical/chemical treatment processes, John Wiley and Sons, Hoboken, 2013 Search PubMed.
- H. Granger, A. Stoddart and G. Gagnon, Direct biofiltration for manganese removal from surface water, J. Environ. Eng., 2014, 140(4), 04014006 CrossRef.
- K. Greenstein, J. Lew, E. Dickenson and E. Wert, Investigation of biotransformation, sorption, and desorption of multiple chemical contaminants in pilot-scale drinking water biofilters, Chemosphere, 2018, 200, 248–256 CrossRef CAS.
-
B. Gage, D. O'Dowd and P. Williams, Biological iron and manganese removal, pilot and full scale applications, Ontario Water Works Association Conference, Toronto, 2001 Search PubMed.
- A. Comeau, W. Li, J. Tremblay, E. Carmack and C. Lovejoy, Arctic ocean microbial community structure before and after the 2007 record sea ice minimum, PLoS One, 2011, 6(11), e27492 CrossRef CAS PubMed.
- A. Comeau, G. Douglas and M. Langille, Microbiome Helper: A custom and streamlined workflow for microbiome research, mSystems, 2017, 2(1), e00127 CrossRef CAS PubMed.
- E. Bolyen, J. Rideout and M. Dillon,
et al., Reproducible, interactive, scalable and extensible microbiome data science using QIIME 2, Nat. Biotechnol., 2019, 37, 852–857 CrossRef CAS.
-
S. Andrews, FASTQC. A Quality Control Tool for High Throughput Sequence Data, Babraham Institute, 2010, https://www.bioinformatics.babraham.ac.uk/projects/fastqc/ Search PubMed.
- M. Martin, Cutadapt removes adapter sequences from high-throughput sequencing reads, EMBnet J., 2011, 17(1), 10 CrossRef.
- C. Quast, E. Pruesse, P. Yilmaz, J. Gerken, T. Schweer, P. Yarza, J. Peplies and F. Glockner, The SILVA ribosomal RNA gene database project: Improved data processing and web-based tools, Nucleic Acids Res., 2013, 41(D1), D590–D596 CrossRef CAS PubMed.
- B. Callahan, P. McMurdie, M. Rosen, A. Han, A. Johnson and S. Holmes, DADA2: High resolution sample inference from Illumina amplicon data, Nat. Methods, 2016, 13(7), 581–583 CrossRef CAS PubMed.
- M. Price, P. Dehal and A. Arkin, FastTree 2 - Approximately maximum-likelihood trees for large alignments, PLoS One, 2010, 5(3), e9490 CrossRef PubMed.
- K. Katoh and D. Standley, MAFFT multiple sequence alignment software version 7: Improvements in performance and usability, Mol. Biol. Evol., 2013, 30(4), 772–780 CrossRef CAS.
-
R Core Team, R: A language and environment for statistical computing, R Foundation for Statistical Computing, Vienna, Austria, 2020, https://www.R-project.org/ Search PubMed.
-
G. Kandlikar, ranacapa: Utility functions and ‘shiny’ app for simple environmental DNA visualizations and analyses, R package version 0.1.0, 2020, https://ggithub.comggauravsk/ranacapa Search PubMed.
- I. Wilson, Inhibition and facilitation of nucleic acid amplification, Appl. Environ. Microbiol., 1997, 63(10), 3741–3751 CrossRef CAS PubMed.
- P. McMurdie and S. Holmes, phyloseq: An R package for reproducible interactive analysis and graphics of microbiome census data, PLoS One, 2013, 8(4), e61217 CrossRef CAS.
-
J. Oksanen, F. Blanchet, M. Friendly, R. Kindt, P. Legendre, D. McGlinn, P. Minchin, R. O'Hara, G. Simpson, P. Solymos, M. Stevens, E. Szoecs and H. Wagner, vegan: Community ecology package, R package version 2.5-6, 2019, https://CRAN.R-project.org/package=vegan Search PubMed.
-
K. Andersen, R. Kirkegaard, S. Karst and M. Albertsen, ampvis2: an R package to analyse and visulaize 16S rRNA amplicon data, BioRxiv, 2018, preprint, p. 299537, DOI:10.1101/299537.
-
J. Arnold, ggthemes: Extra themes, scales and geoms for ‘ggplot2’, R packaged version 4.2.0, 2019, https://CRAN.R-project.org/package=ggthemes Search PubMed.
- H. Wickham, M. Averick, J. Bryan, W. Chang, L. McGowan, R. Francois and G. Grolemund,
et al., Welcome to the tidyverse, J. Open Source Softw., 2019, 4(43), 1686 CrossRef.
-
C. Wilke, cowplot: Streamlined plot theme and plot annotations for ‘ggplot2’, R package version 1.1.0, 2020, https://CRAN.R-project.org/package=cowplot Search PubMed.
- I. Breda, L. Ramsay and D. Soborg, The role of backwash in start-up of full-scale drinking water biofilters, J. Water Supply: Res. Technol.--AQUA, 2016, 65(3), 234–243 CrossRef.
- E. Gregory and J. T. Staley, Widespread distribution of ability to oxidize manganese among freshwater bacteria, Appl. Environ. Microbiol., 1982, 44, 509–511 CrossRef CAS PubMed.
- L. I. Sly, M. C. Hodgkindon and V. Arunpairojana, Effect of water velocity on the early development of manganese-depositing biofilm in a drinking-water distribution system, FEMS Microbiol. Lett., 1988, 53, 175–186 CrossRef CAS.
- A. Templeton, H. Staudigel and B. Tebo, Diverse Mn(II)-oxidizing bacteria isolated from submarine basalts at Loihi Seamount, Geomicrobiol. J., 2005, 22, 127–139 CrossRef CAS.
- V. Cahyani, J. Murase, E. Ishibashi, S. Asakawa and M. Kimura, Phylogenetic positions of Mn2+−oxidizing bacteria and fungi isolated from Mn nodules in rice field subsoils, Biol. Fertil. Soils, 2009, 45, 337–346 CrossRef.
- J. Cerrato, J. Falkinham, A. Dietrich, W. Knocke, C. McKinney and A. Pruden, Manganese-oxidizing and reducing microorganisms isolated from biofilms in chlorinated drinking water systems, Water Res., 2010, 44, 3935–3945 CrossRef CAS PubMed.
- M. Carmichael, S. Carmichael, C. Santelli, A. Strom and S. Brauer, Mn(II)-oxidizing bacteria are abundant and environmentally relevant members of ferromanganese deposits in caves of the upper Tennessee River Basin, Geomicrobiol. J., 2013, 30(9), 779–800 CrossRef CAS.
- W. Yang, Z. Zhang, Z. Zhang, H. Chen, J. Liu, M. Ali, F. Liu and L. Li, Population structure of manganese-oxidizing bacteria in stratified soils and properties of manganese oxide aggregates under manganese-complex medium enrichment, PLoS One, 2013, 8(9), e73778 CrossRef CAS PubMed.
- P. Yli-Hemminki, K. Jorgensen and J. Lehtoranta, Iron-Manganese concretions sustaining microbial life in the Baltic sea: The structure of the bacterial community and enrichments in metal-oxidizing conditions, Geomicrobiol. J., 2014, 31(4), 263–275 CrossRef CAS.
- S. Mayanna, C. Peacock, F. Schaffner, A. Grawunder, D. Merten, E. Kothe and G. Buchel, Biogenic precipitation of manganese oxides and enrichment of heavy metals at acidic soil pH, Chem. Geol., 2015, 402, 6–17 CrossRef CAS.
- T. Bohu, D. Akob, M. Abratis, C. Lazar and K. Kusel, Biological low-pH Mn(II) oxidation in a manganese deposit influenced by metal-rich groundwater, Appl. Environ. Microbiol., 2016, 82(10), 3009–3021 CrossRef CAS PubMed.
- D. N. Marcus, A. Pinto, K. Anantharaman, S. A. Ruberg, E. L. Kramer, L. Raskin and G. J. Dick, Diverse manganese (II) - oxidizing bacteria are prevalent in drinking water systems, Environ. Microbiol. Rep., 2017, 9(2), 120–128 CrossRef CAS.
- S. Dangeti, J. McBeth, B. Roshani, J. Vyskocil, B. Rindall and W. Chang, Microbial communities and biogenic Mn-oxides in an on-site biofiltration system for cold Fe-(II)- and Mn(II)-rich groundwater treatment, Sci. Total Environ., 2020, 710, 136386 CrossRef CAS.
- P. Corstjens, J. de Vrind, P. Westbroek and E. de Vrind-de Jong, Enzymatic iron oxidation by Leptothrix discophora: identification of an iron-oxidizing protein, Appl. Environ. Microbiol., 1992, 58(2), 450–454 CrossRef CAS.
- L. Hallbeck, F. Stahl and K. Pedersen, Phylogeny and phenotypic characterization of the stalk-forming and iron-oxidizing bacterium Gallionella ferruginea, Microbiology, 1993, 139, 1531–1535 CrossRef CAS PubMed.
- J. Liu, Z. Wang, S. M. Belchik, M. J. Edwards, C. Liu, D. Kennedy, E. Merkley, M. Lipton, J. Butt, D. Richardson, J. Zachara, J. Fredrickson, K. Rosso and L. Shi, Identification and characterization of MtoA: a decaheme c-type cytochrome of the neutrophilic Fe(II)-oxidizing bacterium Sideroxydans lithotrophicus ES-1, Front. Microbiol., 2012, 3, 37 Search PubMed.
-
J. P. Bowman, in The Prokaryotes: The Family Methylococcaceae, ed. E. Rosenberg, E. DeLong, S. Lory, E. Stackebrandt and F. Thompson, Springer-Verlag, Berlin, 2014 Search PubMed.
-
H. Daims, in The Prokaryotes: The Family Nitrospiraceae, ed. E. Rosenberg, E. DeLong, S. Lory, E. Stackebrandt and F. Thompson, Springer-Verlag, Berlin, 2014 Search PubMed.
- S. Kato, S. Krepski, C. Chan, T. Itoh and M. Ohkuma, Ferriphaelus amnicola gen. nov., sp. nov., a neutrophilic, stalk-forming, iron-oxidizng bacterium isolated from an iron-rich groundwater seep, Int. J. Syst. Evol. Microbiol., 2014, 64, 921–925 CrossRef CAS PubMed.
-
J. Prosser, I. Head and L. Stein, in The Prokaryotes: The Family Nitrosomonadaceae, ed. E. Rosenberg, E. DeLong, S. Lory, E. Stackebrandt and F. Thompson, Springer-Verlag, Berlin, 2014 Search PubMed.
- K. Kitzinger, H. Koch, S. Lucker, C. Sedlacek, C. Herbold, J. Schwartz, A. Daebeler, A. Mueller, M. Lukumbuzya, S. Romano, N. Leisch, S. Karst, R. Kirkegaard, M. Albertsen, P. Nielsen, M. Wagner and H. Daims, Characterization of the first “Candidatus Nitrotoga” isolate reveals metabolic versatility and separate evolution of widespread nitrite-oxidizing bacteria, MBio, 2018, 9(4), e01186 CrossRef CAS PubMed.
- Y. Cai, D. Li, Y. Liang, H. Zeng and J. Zhang, Operational parameters required for the start-up process of a biofilter to remove Fe, Mn, and NH3-N from low-temperature groundwater, Desalin. Water Treat., 2014, 57(8), 3588–3596 CrossRef.
- Y. Zhang, R. Sun, A. Zhou, J. Zhang, Y. Luan, J. Jia, X. Yue and J. Zhang, Microbial community response reveals underlying mechanism of industrial-scale manganese sand biofilters used for the simultaneous removal of iron, manganese and ammonia from groundwater, AMB Express, 2018, 8, 2 CrossRef PubMed.
- J. Deutzmann, M. Hoppert and B. Schink, Characterization and phylogeny of a novel methanotroph, Methyloglobulus morosus gen. nov., spec. nov., Syst. Appl. Microbiol., 2014, 37(3), 165–169 CrossRef CAS PubMed.
-
H. Webb, H. J. Ng and E. Ivanova, in The Prokaryotes: The Family Methylocystaceae, ed. E.
Rosenberg, E. DeLong, S. Lory, E. Stackebrandt and F. Thompson, Springer-Verlag, Berlin, 2014, pp. 341–347 Search PubMed.
-
D. Kelly, I. McDonald and A. Wood, in The Prokaryotes: The Family Methylobacteriaceae, ed. E. Rosenberg, E. DeLong, S. Lory, E. Stackebrandt and F. Thompson, Springer-Verlag, Berlin, 2014, pp. 341–347 Search PubMed.
-
N. Doronina, E. Kaparullina and Y. Trotsenko, in The Prokaryotes: The Family Methylophilaceae, ed. E. Rosenberg, E. DeLong, S. Lory, E. Stackebrandt and F. Thompson, Springer-Verlag, Berlin, 2014, pp. 341–347 Search PubMed.
- I. Breda, L. Ramsay and P. Roslev, Manganese oxidation and bacterial diversity on different filter media coatings during the start-up of drinking water biofilters, J. Water Supply: Res. Technol.--AQUA, 2017, 66(8), 641–650 CrossRef.
- Q. Cheng, Y. Huang, L. Nengzi and J. Zhang, Performance and microbial community profiles in pilot-scale biofilter for the simultaneous removal of ammonia, iron, and manganese at different manganese concentrations, Bioprocess Biosyst. Eng., 2019, 42, 741–752 CrossRef CAS PubMed.
- L. Poghosyan, H. Koch, J. Frank, M. van Kessel, G. Cremers, T. van Alen, M. Jetten, H. Op den Camp and S. Lucker, Metagenomic profiling of ammonia- and methane-oxidizing microorganisms in two sequential rapid sand filters, Water Res., 2020, 185, 116288 CrossRef CAS PubMed.
- W. Hu, J. Liang, F. Ju, Q. Wang, R. Liu, Y. Bai, H. Liu and J. Qu, Metagenomics unravels differential microbiome composition and metabolic potential in rapid sand filters purifying surface water versus groundwater, Environ. Sci. Technol., 2020, 54, 5197–5206 CrossRef CAS PubMed.
- C. Volk and M. LeChevallier, Assessing biodegradable organic matter, J. - Am. Water Works Assoc., 2000, 92(5), 64 CrossRef CAS.
-
Standard Methods for the Examination of Water and Wastewater, ed. E. Rice, R. Baird, A. Eaton and L. Clesceri, American Public Health Association/American Water Works Association/Water Environment Federation, Washington, 22nd edn, 2012 Search PubMed.
- G. de Vera, D. Gerrity, M. Stoker, W. Frehner and E. Wert, Impact of upstream chlorination on filter performance and microbial community structure of GAC and anthracite biofilters, Environ. Sci.: Water Res. Technol., 2018, 4, 1133 RSC.
- G. de Vera and E. Wert, Using discrete and online ATP measurements to evaluate regrowth potential following ozonation and (non)biological drinking water treatment, Water Res., 2019, 154, 377–386 CrossRef CAS PubMed.
- A. Stoddart, J. Schmidt and G. Gagnon, Biomass evolution in full-scale anthracite-sand drinking water filters following conversion to biofiltration, J. - Am. Water Works Assoc., 2016, 108, E615 CrossRef.
- L. Pharand, M. Van Dyke, W. Anderson and P. Huck, Assessment of biomass in drinking water biofilters by adenosine triphosphate, J. - Am. Water Works Assoc., 2014, 106(10), E433–E444 CrossRef.
- D. Limoli, C. Jones and D. Wozniak, Bacterial extracellular polysaccharides in biofilm formation and function, Microbiol. Spectrum, 2015, 3(3), MB-0011-2014 Search PubMed.
|
This journal is © The Royal Society of Chemistry 2023 |
Click here to see how this site uses Cookies. View our privacy policy here.