Performance of the solar/peroxymonosulfate process in (waste)water treatment: abatement of micropollutants, roles of reactive oxygen species, and formation of disinfection by-products†
Received
14th September 2022
, Accepted 10th November 2022
First published on 14th November 2022
Abstract
This study investigated the effectiveness of peroxymonosulfate (PMS) as an oxidative agent in micro-contaminant removal with activation via simulated solar irradiation (Xe-lamp, emission spectrum ranging from 300 to 800 nm). During solar/PMS treatment, the abatement of nitrobenzene (NB), flunixin meglumine (FMME), aspirin (ASA), and benzoic acid (BA) followed pseudo-first order kinetics, with degradation at pH 8.5 faster (1.28–1.78 times) than that at pH 5.5. The removal of NB (1.22 mg L−1), FMME (4.92 mg L−1), ASA (1.80 mg L−1), and BA (1.23 mg L−1) reached 51.2%, 68.0%, 66.6%, and 90.7% in 15 min at pH 8.5, respectively, with corresponding pseudo-first order rate constants (kobs) of 5.1 × 10−2, 7.7 × 10−2, 7.4 × 10−2, and 1.6 × 10−1 min−1, respectively. Production of hydroxyl radicals, sulfate radicals (SO4˙−), and other reactive oxygen species (ROS) was determined by using NB and BA as probe compounds in the solar/PMS system. At pH of 5.5–8.5, SO4˙− made a dominant contribution (45.7% and 52.0%, respectively) to the overall removal of ASA and FMME. Compared with treatment of chlorination alone, solar/PMS pretreatment followed by post-chlorination resulted in a significant increase in disinfection by-product (DBP) formation (by 176% for trihalomethanes and 273% for haloacetic acids) in the Yangtze River water samples, as well as inhibition of algae growth (by 35.6%) due to higher toxic effects of residual PMS or potential oxidation intermediates in the system. The lowest EE/Ototal consumed in the solar/PMS system was estimated to be 72–110 kW h m−3 order−1 with the optimal PMS dosages of 0.36–0.68 mM. This work identified ROS generated during solar/PMS treatment and evaluated their contributions to the efficient depletion of organic micro-pollutants. The potential risk of increased DBP production and bio-toxicity was also investigated when applying the system to actual water treatment.
Water impact
The solar/peroxymonosulfate (PMS) process demonstrates efficient removal and cost-effective application in degrading emerging organic contaminants. This study identified reactive oxygen species involved in the solar/PMS system and revealed their contributions to pollutant elimination. Furthermore, production of disinfection by-products during chlorination with solar/PMS pre-oxidation and bio-toxicity of solar/PMS-treated water were evaluated to assess the potential applications of this technology in water and wastewater treatment.
|
1. Introduction
Non-steroidal anti-inflammatory drugs (NSAIDs) are widely present in natural water and wastewater systems, with concentrations ranging from ng L−1 to μg L−1,1e.g., pharmaceuticals and personal care products (PPCPs) were detected with mean concentrations of 6.57 μg L−1 and 2.14 μg L−1 in influents and effluents, respectively, from six wastewater treatment plants (WWTPs) along the Yangtze River, North China.2 Elimination of such micro-pollutants with conventional water treatment processes is often limited (e.g., removals of 18 micro-pollutants were <30% during clarification and sand filtration processes),3 due to negative removal effects by biological treatment processes which are originally designed for removal of organic matter and suspended solids.4 Thus, searching for more effective techniques is of extensive interest to water treatment researchers. Advanced oxidation processes (AOPs), such as ultraviolet-based AOPs,5 heterogeneous photo-catalysis,6 Fenton and photo-Fenton processes,7 and persulfate-based AOPs,8 are considered as efficient treatments for decomposition of these chemicals. Among them, persulfate-AOP systems are the current research hotspot because of sulfate radicals (SO4˙−) having higher selectivity and longer half-life than hydroxyl radicals (˙OH) and being able to achieve similar or better performance in degradation of emerging pollutants.9
Peroxymonosulfate (PMS) is one of the strongest oxidative agents with a redox potential of 1.82 V among those applied in water remediation.10 However, it has relatively high selectivity in oxidizing environmental organic pollutants,11i.e., low reactivity toward certain contaminants. For example, the PMS alone process (80 μM) showed low conversion (<2%) of 5.33 mg L−1 atenolol at pH 7.0 within 10 min.12 Therefore, PMS treatment needs to be combined with thermal heating,13 transition metals,14 nanocarbon materials,15 and ultraviolet16 or solar irradiation,17 which provide extra energy aiming to break the O–O bond in the PMS molecule and form highly reactive species like SO4˙− and ˙OH to improve degradation efficiencies of persistent pollutants via radical oxidation pathways.18 Among various forms of energy, solar irradiation has been increasingly used in water remediation as a cheap, environmental-friendly, and renewable source of energy.19 It is composed of UVA-UVB (280–400 nm), visible light (400–760 nm), and infrared light (760–4000 nm).20 Although the UV region (3% for UVB and 97% for UVA) accounts for less than 5% of solar spectra,21 solar UVA-UVB radiation induces various direct and indirect photochemical processes resulting in micro-pollutant degradation in natural water bodies.22 Several solar-driven AOPs demonstrated notable performance in removing organic micro-pollutants. During free available chlorine (FAC) photolysis at simulated sunlight (300–400 nm), the production of reactive oxygen species (ROS) (e.g., O3, ˙OH) led to 88.6% removal of carbamazepine within 70 min in buffered solutions.23 The herbicide 2,4-dichlorophenoxyacetic acid was totally eliminated after a 3-h treatment with hydrogen peroxide (H2O2)/UV-B+A light (3 mW cm−2) at pH 3.6 with Xe-lamp irradiation simulating sunlight.24 Addition of 10 mg L−1 peracetic acid (PAA) resulted in 56% removal of carbamazepine in wastewater under simulated sunlight after 300 min.25 Taken together, solar irradiation is considered as a promising cost-effective approach for large-scale aqueous-phase applications. In comparison to other commonly used agents in forms of liquid (e.g., FAC, H2O2, and PAA) added to the system, solid-form oxidant PMS is much more stable and easier to be transported. Besides, SO4˙−-based AOPs are sometimes superior to ˙OH-based AOPs because degradation of organic pollutants by SO4˙− proceeds through selective pathways.8 Exposed to sunlight, PMS absorbs photons to produce ROS, e.g., ˙OH and
, which further react with micro-pollutants.26 Under natural sunlight, nearly 20% removal of sulfamethoxazole was achieved with addition of 0.01 mM PMS into distilled water in 30 min.27 Almost 85% elimination of bezafibrate (initial concentration 250 μg L−1) was achieved in 2 h by treatment with 400 μM PMS with exposure to simulated sunlight.28 This system could be applied to tertiary treatment in urban wastewater treatment plants to degrade trace organic contaminants prior to their discharge into the receiving waters.29 Besides, the solar/PMS system presents potent disinfecting action, which could be used in swimming pool disinfection to avoid excessive chlorination.30 Moreover, this solar-based process is expected to be implemented in isolated areas with high solar radiation incidence and for treatment of small water volumes.21 However, few studies simultaneously identified ROS generated during solar/PMS treatment and evaluated their contributions to organic pollutant removal.31,32 In terms of practical application in water and wastewater treatment, the economic cost and optimal oxidant consumption have not been well documented. In addition, little work has been conducted to investigate toxicity of disinfection byproducts (DBPs) formed using solar/PMS as a pretreatment prior to final disinfection.
In this study, a solar/PMS system was investigated for its performance in contaminant elimination using nitrobenzene (NB), flunixin meglumine (FMME), aspirin (ASA), and benzoic acid (BA) as model compounds. ASA and FMME are two kinds of emerging PPCPs, resulting in widespread existence (ng L−1–μg L−1) of water contamination and environmental risks;33 for example, ASA was detected at 25.3 ± 1.26
μg L−1 in surface water at the sampling site Msunduzi in South Africa.34 As industrial pollutants, 19 million pounds of NB is released into the environment,35 while the global BA production potential reaches 600
000 tonnes annually.36 The objectives of this work were: (i) to determine degradation kinetics of the model contaminants and contributions of various ROS to contaminant degradation during solar/PMS treatment; (ii) to investigate the toxic effects of solar/PMS as pre-treatment prior to final disinfection, in terms of formation of DBPs and consequent bio-toxicity to algae; and (iii) finally, to perform economic analysis to evaluate feasibility of applying the solar/PMS process in the actual water treatment practices.
2. Experimental section
2.1 Materials
A list of chemicals and materials used in this study is provided in Text S1.† The secondary-treated wastewater sample was taken in January 2021 at the effluent of secondary treatment (secondary clarifier) from Chengnan Wastewater Treatment Plant in Nanjing, Jiangsu Province, China. Water from the Yangtze River was collected at latitude 32.0°N and longitude 118.7°E in February 2021. After filtration by 0.22-μm mixed cellulose ester (MCE) membrane filters (50 mm diameter), water samples were stored at 4 °C before use. Water quality parameters were recorded with the overall information shown in Table S2.†
2.2 Experimental devices and procedures
The photolysis experiments were conducted with a high brightness parallel light source system (CHF-XM500, Beijing Perfectlight Technology Co., Ltd. China) equipped with 500 W ultra high-pressure short-arc xenon lamps to simulate sunlight (300–800 nm consisting of UV and visible light). The UV photon fluence rate of the simulated sunlight was determined previously to be 1.14 × 10−4 E m−2 s−1 and the effective light intensity of the apparatus was 6.06 W cm−2.37 Natural sunlight experiments, undertaken to evaluate the use of PMS photolysis under conditions representative of point-of-use solar applications, were conducted on the roof of Jinshi Chen building on the campus of Southeast University (latitude: 31.89°N, longitude: 118.82°E) without thermo-stating, at an ambient temperature of (37 ± 3 °C).
Experimental devices and procedures used in this study are described in Text S2.†
2.3 Analytical methods
Concentrations of NB, FMME, ASA, and BA were determined using an ultra-performance liquid chromatography (UPLC) system (Waters, USA) equipped with an Acqutiy BEH-C18 column (1.7 μm, 2.1 × 100 mm) followed by a UV-vis detector (Table S3†). Total organic carbon (TOC) concentrations in water samples were determined using a TOC-L CPH type TOC analyzer (Shimadzu, Japan). Concentrations of trihalomethanes (THMs) and haloacetic acids (HAAs) in samples taken from the reaction solutions, directly chlorinated or pretreated by solar/PMS followed with chlorination, were extracted with methyl tert-butyl ether (MTBE) and determined by gas chromatography with an electron capture detector (GC/ECD) according to the USEPA-551 and USEPA-552 methods.38 Dissolved sulfate (SO42−) concentration was detected using the Ba2+-based sulfaVer4 method (HACH module No.8051) with reference to the USEPA-375.4 method.39 Peroxymonosulfate concentration was measured with the ABTS method using a UV-vis spectrophotometer (HACH, DR6000).40
2.4 PMS and simulated solar treatment procedures
Treatments were undertaken using 10 mM phosphate buffer (PB) solutions or actual water samples at pH 5.5, 7.0, or 8.5, according to the following approaches: (i) exposure to simulated sunlight or natural sunlight alone (solar alone or sunlight alone), (ii) exposure to PMS alone, (iii) exposure to simulated sunlight or natural sunlight in the presence of PMS (solar/PMS or sunlight/PMS), (iv) exposure to solar/PMS with natural organic matter (NOM), and (v) exposure to solar/PMS followed by chlorination. All the experiments were conducted in at least duplicate. Four pollutants, NB (1.22 mg L−1), FMME (4.92 mg L−1), ASA (1.80 mg L−1), and BA (1.23 mg L−1), were dosed as model compounds to investigate the degradation efficiencies by solar/PMS. NB and BA were also used as the probe contaminants for identification of ROS.
2.5 Data processing methods
2.5.1 Kinetic methods.
Pseudo-first order kinetics were applied in modeling degradation kinetics of the model contaminants, as shown in eqn (1) | 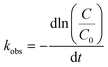 | (1) |
where t is the reaction time (min), kobs is the observed pseudo-first order rate constant (min−1), C is the concentration (M) of the target contaminant at time t, and C0 is its initial concentration (M) at t = 0.
2.5.2 Activation energy calculation.
The Arrhenius formula was used to calculate the activation energies of different pollutants with respect to temperature according to eqn (2). | 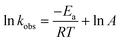 | (2) |
where A represents the frequency factor (min−1), Ea is the chemical reaction activation energy (kJ mol−1), R is the universal gas constant (J mol−1 K−1), and T is the absolute temperature (K) of the reaction.
2.5.3 Toxicity assays.
Acute toxicity assays were performed for solar/PMS treated samples by measuring the biomass production of algae and its chlorophyll and carotenoid contents. Details of the assay procedures, determination of pigment contents, and calculation of growth inhibition rates are provided in Text S3.†
2.5.4 Economic analysis.
Electrical energy per order (EE/O, kW h m−3 order−1) includes the equivalent electrical energy consumption of the oxidant (EE/OOxidant, kW h m−3 order−1) and the power consumption of the simulated sunlight device (EE/OSolar, kW h m−3 order−1), with EE/OOxidant being the product of oxidant consumption (Oxidant/O, mg L−1 order−1) and electricity consumption per amount of oxidant production (Oxidantenergy, kW h mg−1).41 The value of PMSenergy was calculated to be 110.17 × 10−6 kW h mg−1 according to the price of electricity ($3.5 × 10−2 kW h −1) and the oxidant ($3.89 kg−1) in Jiangsu Province, China. Calculations for the above terms are shown in eqn (3)–(5). | 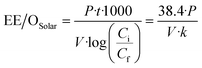 | (3) |
| 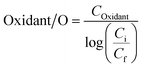 | (4) |
| EE/OOxidant = Oxidantenergy × Oxidant/O | (5) |
where P is the effective optical power of the simulated sunlight device (kW), t is the reaction time required for one order of magnitude of pollutant degradation (h), V is the total volume of the solution (L), k denotes the observed rate constant for pollutant degradation (min−1), Ci and Cf mean the initial and post-reaction concentrations of the target species (mM), respectively, and COxidant is the concentration of the oxidant in solutions (mg L−1).
2.5.5 DBPs cytotoxicity analysis.
The cytotoxicity of DBPs generated during chlorination with and without solar/PMS pretreatment was calculated according to molar concentrations of DBPs and lethal concentration 50% (LC50) values42 with details described in Text S4.†
2.5.6 Statistical analysis.
Statistically significant differences among treatments were obtained by analysis of variance (ANOVA) using SPSS 22.0 (SPSS Inc., Chicago, USA). The data groups were considered significantly different at p < 0.05.
3. Results and discussion
3.1 Solar-induced rapid degradation
Control experiments were undertaken to examine the effect of solar radiation or PMS alone on the abatement of the selected target compounds. As shown in Fig. S1,† elimination of contaminants (NB, FMME, ASA, and BA) was negligible (<4%) after 15 min of treatment with solar irradiation alone (6.06 W cm−2) at circumneutral pH. Prior work also showed that solar irradiation alone (2.0 × 10−3 W cm−2) yielded poor degradation (<14%) of polyvinyl alcohol after 6 h of irradiation.43 This result demonstrated that solar irradiation alone without generation of strong ROS could hardly lead to decomposition of organic pollutants. Treatment with PMS alone also led to generally low removal of all the four model contaminants (<5%) at circumneutral pH. Compared to treatment with either solar irradiation or PMS alone (Fig. S1†), the combination of PMS and simulated sunlight performed better in degrading pollutants with the other experimental conditions kept identical (pH = 7.0), improving the removal rates of NB, FMME, ASA, and BA to 41.7%, 61.4%, 63.1%, and 81.2%, respectively. This result implied generation of ROS in the solar/PMS system (λ > 300 nm).44 As shown in Fig. S2a,† elimination of FMME, ASA, and BA by natural sunlight irradiation alone was negligible (<1%) at pH 7.0. An obvious decrease of NB concentration (40.2%) in solution (at nearly 30 °C after 15 min of irradiation without temperature control) may be attributed to volatilization with a boiling temperature of 210.85 °C.45 During sunlight/PMS treatment, the removal of NB, FMME, ASA, and BA was 42.7%, 25.0%, 20.1%, and 13.9%, respectively (Fig. S2b†), lower than that under simulated solar irradiation. This could be explained by that the highest solar radiation intensity of natural sunlight in Nanjing was 1092.45 W m−2 in summer according to a previous survey,46 lower than the light intensity in the simulated sunlight experiment here (6.06 × 104 W m−2). Nevertheless, the result revealed the activation effect of PMS by natural sunlight, demonstrating the potential application of the solar/PMS system.
3.2 pH dependent degradation
The effect of pH on degradation of the target compounds was investigated for treatments of PMS alone and PMS/solar at varying pH conditions (solar irradiation alone was found to have minimal impact on pollutant degradation and thus not investigated further). Fig. S3† presents the oxidation effect of PMS alone on contaminant elimination and removal efficiencies increased with pH varying from 5.5 to 8.5. After 15 min, depletion of NB, FMME, ASA, and BA at pH 8.5 reached 9.8%, 9.3%, 9.2%, and 4.1%, respectively, higher than that at pH 5.5 (0.6–5.8%) and pH 7.0 (2.0–7.0%), which warrants further investigation at higher PMS exposures. Prior work also showed that degradation of ibuprofen after treatment with PMS for 120 min was <10% at pH values of 5.0 and 7.0, while it increased to 41% when pH increased to 9.0.32 The higher depletion of contaminants at higher pH might be in part related to activation of PMS with hydroxyl anions in a weakly basic environment, generating the superoxide radical (O2˙−) and singlet oxygen (1O2).47
Fig. 1 presents the removal of NB, FMME, ASA, and BA in the solar/PMS system at varying pH conditions. After 15 min of solar/PMS treatment, NB, FMME, ASA, and BA were degraded by 35.9%, 57.0%, 56%, and 73.3% at pH 5.5, respectively, while their elimination increased to 51.2%, 68.0%, 66.6% and 90.7% at pH 8.5 (contaminant removal: pH 8.5 > pH 7.0 > pH 5.5), respectively. Similarly, previous work reported that bisphenol A removal in the UV-C/PMS system also increased (from 88.3% to 97.9%) with the pH value increasing from 5.0 to 10.0, indicating that alkaline conditions might facilitate PMS activation.48 The degradation kinetics of the four contaminants during solar/PMS treatment at all pH conditions followed pseudo-first order kinetics, as shown in Fig. S4.† The observed pseudo-first order rate constants (kobs) for each contaminant at different pH values were determined through linear regression of the degradation curves and are summarized in Fig. S5.† For all the four contaminants, kobs increased with pH increasing from 5.5 to 8.5, again supporting that higher pH facilitated contaminant removal in the solar/PMS system. In addition, values of kobs for the four contaminants were kobs-NB = 3.2–5.1 × 10−2 min−1, kobs-FMME = 5.9–7.7 × 10−2 min−1, kobs-ASA = 5.8–7.4 × 10−2 min−1, and kobs-BA = 0.9–1.6 × 10−1 min−1, implying that the order of contaminant degradation efficiency was as follows: BA > FMME ≈ ASA > NB.
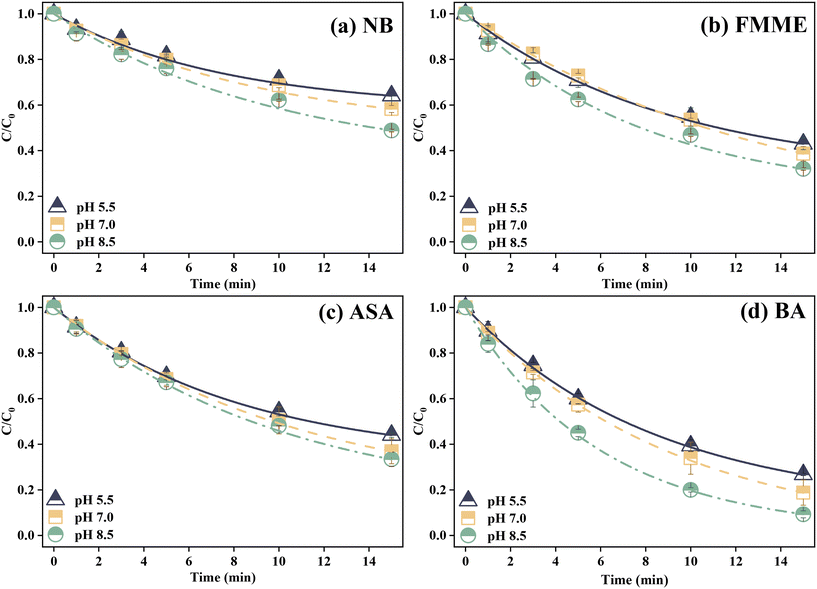 |
| Fig. 1 Effect of pH on degradation of (a) NB, (b) FMME, (c) ASA and (d) BA in the solar/PMS system. [NB]0 = 1.22 mg L−1, [FMME]0 = 4.92 mg L−1, [ASA]0 = 1.80 mg L−1, [BA]0 = 1.23 mg L−1, [PMS]0 = 2.0 mM, pH 5.5, 7.0 and 8.5 phosphate buffer, intensity of simulated sunlight = 6.06 W cm−2, T = 25 °C. | |
The association of the pollutant degradation rates with pH could be explained from several aspects. Firstly, PMS mainly exists in the form of HSO5− in the pH range of 6–8, while nearly half of HSO5− deprotonates into a more reactive species (SO52−) in alkaline medium (HSO5− ⇌ SO52− + H+; pKa = 9.4).49 The decay of SO52− also contributed to generation of SO4˙− in the system.50 Secondly, SO4˙− could react with OH− to form ˙OH at alkaline pH (>10%) with a rate constant of 6.5 × 107 M−1 s−1,50 which might result in enhanced pollutant degradation. Thirdly, the solution pH could affect the speciation of pollutants and accordingly their degradation efficiency. Generally, the reactivity of contaminants toward ROS increases with their degree of deprotonation,9 likely due to the ionic forms of organic contaminants having higher electron-donating abilities than their protonated species.51 The dissociation constants (pKa) of ASA, FMME, NB, and BA were 3.5, 5.8, 4.0, and 4.2, respectively.52 For instance, the concentration ratios of ionic/protonated species ([A−]/[HA]) for NB, FMME, ASA, and BA were calculated to be 31, 0.5, 100, and 20 at pH 5.5 according to the Henderson–Hasselbalch equation. While pH is higher than pKa (at pH 8.5), the ionic forms of the model pollutants become more dominant in the reaction solutions, leading to overall higher degradation efficiencies in the solar/PMS system. The result here is consistent with previous work that the deprotonated form of sulfamethoxazole exhibited higher reactivity towards SO4˙− by an electron-transfer mechanism.53 Besides the above, it should also be considered that phosphate used as a buffer reagent at pH 7.0 in the reaction solution could serve as a PMS activator to generate SO4˙−.54 The phosphate (pKa1 = 2.1, pKa2 = 7.2, and pKa3 = 12.3) was present predominantly as H2PO4− in the pH range from 2.1 to 7.2, while as HPO42− in the pH range from 7.2 to 8.5. At alkaline pH, PO43− and HPO42− as the main components could efficiently break the O–O bond of PMS.54 Thus, with elevation of solution pH and disassociation of H2PO4− into HPO42−, the contaminant decomposition was enhanced due to increasing activation of PMS with increasing fraction of HPO42−. Previous work reported that increasing phosphate concentration significantly (varying from 0.0 to 0.1 M) enhanced the removal of acid orange 7 during the PMS alone process.55 In this study, the total phosphate concentration (10 mM) was 1000-fold of the initial concentration of the target pollutants (10 μM), indicating yields of SO4˙− likely due to phosphate activation. In summary, it could be concluded that alkaline conditions (pH 8.5) were more favorable for the removal of the four pollutants with maximum kobs of 7.7 × 10−2–1.6 × 10−1 min−1, compared with neutral and acidic conditions in the solar/PMS system.
3.3 ROS identification and contribution
Two of the model contaminants, NB and BA, were also employed here as probe compounds to identify ROS and their contributions in contaminant removal in the solar/PMS system. Specifically, NB selectively reacts with ˙OH (k˙OH-NB = 4.7 × 109 M−1 s−1),56 while BA reacts rapidly with both SO4·−(kSO4·−-BA = 1.2 × 109
M−1
s−1)57 and ˙OH (k˙OH-BA = 5.3 × 109 M−1 s−1).58 The degradation of NB and BA was 91.5% and 85.1% after 15 min of solar/PMS treatment at pH 7.0, which both well fitted the pseudo-first-order kinetics, with corresponding kobs values determined to be 1.2 and 1.7 × 10−1 min−1, respectively (Fig. S6†). The results of NB degradation revealed that ˙OH was generated in the system and its steady-state concentration ([˙OH]ss) was calculated to be 2.98 × 10−14 M according to eqn (6). Assuming that BA was degraded only by ˙OH in the system, the kobs-BA was calculated to be 2.6 ×10−3 min−1, which is 2 orders of magnitude lower than the actual determined kobs-BA (1.7 × 10−1 min−1). This in turn demonstrated that SO4˙− was also involved in the system. The steady-state concentration of SO4˙− ([SO4˙−]ss) was calculated to be 8.48 × 10−13 M according to eqn (7) in the solar/PMS system at pH 7.0, which is 1-order of magnitude higher than [˙OH]ss, indicating the dominant contribution of SO4˙− to elimination of BA. In addition to generation of ˙OH and SO4˙−, PMS activation also leads to non-radical pathways (e.g.1O2 generation),59 although 1O2 and O2˙− were not investigated in this study. | kobs-NB = [˙OH]ss·k˙OH-NB | (6) |
| kobs-BA = [˙OH]ss·k˙OH-BA + [SO4˙−]ss·kSO4˙−-BA | (7) |
In the solar/PMS system containing the four model pollutants, the kobs-NB value was determined to be 0.040 min−1 at pH 7.0, with [˙OH]ss calculated to be 1.43 × 10−13 M according to eqn (6). Meanwhile, the kobs (min−1) value of BA decomposition (by both ˙OH and SO4˙−) was determined to be 0.112 min−1 at pH 7.0, and [SO4˙−]ss was calculated to be 9.24 × 10−13 M according to eqn (7). The second order rate constants of ˙OH reacting with ASA and FMME have been previously measured to be 2.53 × 109 M−1 s−1 (k˙OH-ASA) and 3.73 × 109 M−1 s−1 (k˙OH-FMME), and those of SO4˙− reacting with ASA and FMME are 5.25 × 108
M−1
s−1(kSO4·−-ASA) and 2.36 × 109
M−1
s−1(kSO4·−-FMME).37 Therefore, the observed rate constants (min−1) of the model contaminant degradation resulting from ˙OH
,
, and other active components
in the solar/PMS system can be calculated according to eqn (8)–(10), and ultimately, contributions of each ROS to the overall degradation of model contaminants were then calculated as
,
, and
. The values of the observed rate constants by each ROS and their contributions are summarized in Table S4,† and illustrated in Fig. 2.
|  | (8) |
|  | (9) |
|  | (10) |
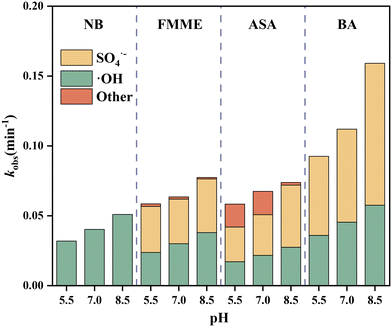 |
| Fig. 2 The kobs values of ROS for the degradation of NB, FMME, ASA, and BA in the solar/PMS system. Conditions: [NB]0 = 1.22 mg L−1, [FMME]0 = 4.92 mg L−1, [ASA]0 = 1.80 mg L−1, [BA]0 = 1.23 mg L−1, [PMS]0 = 2.0 mM, pH 5.5, 7.0 and 8.5 phosphate buffer, simulated sunlight intensity = 6.06 W cm−2, T = 25 °C. | |
As shown in Fig. S7,† SO4˙− played a predominant role in ASA and FMME degradation at all three pH values. The relative contributions of SO4˙− to ASA and FMME degradation were 42.4–60.2% and 49.5–56.3% with pH ranging from 5.5 to 8.5, respectively. The contribution of ˙OH to ASA and FMME removal was calculated to be 29.4–37.2% and 40.6–49.1% at pH 5.5–8.5, respectively (Table S4†). Other active ROS accounted for the minimum contribution to ASA and FMME degradation (2.6–28.2% and 1.4–3.1% at pH 5.5–8.5, respectively), although one should be aware that under neutral and acidic conditions, more than 20% of ASA degradation was caused by other ROS.
The results here are consistent with previous findings. It has been reported that ˙OH merely accounted for 4.6% while SO4˙− accounted for 52.7% on dodecyl dimethyl benzyl ammonium chloride degradation in a UV254/persulfate system at pH 7.0.60 It has also been reported that SO4˙− was the most important reactive species (making a contribution of 62.6%) in removing sulfamethoxazole in the Co2+/H3BO3/PMS system at pH 8.0.61 The higher contribution of SO4˙− is thought to be partly due to the redox potential of SO4˙− (2.6–3.1 V) being higher than that of ˙OH (1.9–2.7 V),62 in addition to [SO4˙−]ss higher than [˙OH]ss in the system. However, another previous study for dichloroacetonitrile degradation by the UV254/PMS process showed that ˙OH played a more vital role than SO4˙−, inconsistent with the results here, which could be explained by dichloroacetonitrile being able to act as an ˙OH scavenger, being more highly reactive toward ˙OH than SO4˙−.63
3.4 Effect of temperature and NOM
To evaluate the effect of temperature on removal of pollutants in the solar/PMS system, neutral solutions (containing 2.0 mM of PMS and four target pollutants) were set up in the thermostat circulator at different temperatures (25–70 °C). Fig. S8† shows that as the temperature changed from 25 °C to 70 °C at pH 7.0, the degradation efficiencies of NB and FMME after 15-min treatment significantly increased (p < 0.05) from 35.9% to 52.3% for NB, and from 57.0% to 71.7% for FMME, while elimination of ASA and BA was only slightly enhanced (with no statistical significance, p > 0.05) from 56.0% to 63.9% for ASA and from 73.3% to 75.4% for BA. Elevated temperature resulted in overall increase in contaminant removal, which appears to be partially due to increased ROS generation by thermal activation of PMS (eqn (11)).9 |  | (11) |
As shown in Fig. 3, the values of ln
kobs strongly correlated with 1/T (R2 > 0.94) in the temperature range of 291–335 K. According to the Arrhenius expression for rate constant (eqn (2)), the average chemical reaction activation energy of NB, FMME, ASA, and BA was calculated to be 17.8, 6.83, 2.95, and 0.61 kJ mol−1, respectively, lower than that required for acid orange 7 decolorization in the base/PMS system (34.53 kJ mol−1) at pH 9.8 (ref. 47) and acetaminophen degradation in the PMS/chlorine process (37.38 kJ mol−1).64 The result implies that the solar/PMS system facilitates contaminant degradation due to the low activation energy required for the chemical reaction.
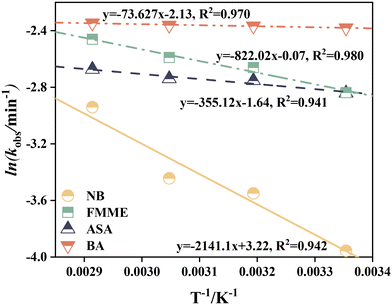 |
| Fig. 3 The kobs of degradation of NB, FMME, ASA, and BA as a function of the reciprocal Kelvin temperature in the solar/PMS system. Conditions: [NB]0 = 1.22 mg L−1, [FMME]0 = 4.92 mg L−1, [ASA]0 = 1.80 mg L−1, [BA]0 = 1.23 mg L−1, [PMS]0 = 2.0 mM, pH 7.0 phosphate buffer, reaction time = 15 min, intensity of simulated sunlight = 6.06 W cm−2, T = 25–70 °C. | |
The potential impact of natural organic matter (NOM) should be considered as an important factor when treating natural water using the solar/PMS system. For treatment with PMS alone (Fig. S9a†), the presence of NOM did not seem to have an apparent influence on contaminant removal (<6.0% decrease), suggesting that NOM had no effect on PMS activation to produce ROS without solar irradiation. For solar irradiation alone (Fig. S9b†), the impact of NOM on pollutant degradation was also negligible (<5.0% decrease), although an increase in the UV/vis spectrum (200 to 500 nm) of the reaction matrix was observed after addition of 6 mg L−1 NOM (Fig. S10†). In the solar/PMS system with NOM concentration increasing from 0.0 to 6.0 mg L−1 (Fig. S11†), the removal efficiencies of NB, FMME, ASA, and BA at 15 min decreased from 35.9%, 57.0%, 60.2%, and 87.2% to 21.7%, 46.0%, 35.6%, and 65.6%, respectively. Fig. S12† shows that the addition of various concentrations of NOM led to a significant drop in the determined values of kobs, compared with that without NOM. For instance, the kobs of ASA decreased by 53.2% (from 0.062 to 0.029 min−1) with NOM dosage from 0.0 mg L−1 to 6.0 mg L−1. Likewise, the kobs of FMME, NB, and BA also reduced by 29.5%, 50.2%, and 47.8% in the presence of NOM. Similar trends were also observed in carbamazepine elimination that kobs values in the presence of NOM were significantly smaller than those obtained in ultrapure water under solar irradiation alone.65 Overall, the above results suggested that NOM significantly inhibited elimination of organic pollutants by solar/PMS treatment, which can be attributed to (i) reduced availability of sunlight for ROS generation by PMS due to its strong absorbance in both the visible and long-wavelength UV spectra,66 and (ii) reduction in ROS steady-state concentrations as NOM act as a scavenger of radicals such as ˙OH.67 Notably, NOM containing ample chromophores could produce ROS such as ˙OH and 1O2 under sunlight radiation, further inducing indirect photo-degradation of organic pollutants.68 However, the inhibition effect of NOM seemed to outweigh the photosensitization effect in the system here.
Pre-filtered WWTP effluent and Yangtze River samples (TOC > 4.0 mg L−1) were examined to evaluate the actual effect in degrading organic pollutants with the solar/PMS system. The apparent inhibitions of contaminant removal were observed during solar/PMS treatment of the WWTP effluent and Yangtze River samples, compared to PB solutions at pH 7.0 (Fig. S13†), further supporting that addition of effluent organic matter or NOM primarily played a role in inhibiting pollutant removal in this study. After 15 min of reaction, elimination of NB, FMME, ASA, and BA decreased to 23.0%, 51.0%, 30.8%, and 55.3% in samples collected from Yangtze River, respectively, and 16.4%, 39.1%, 30.6%, and 48.2% in samples obtained from the WWTP, respectively, compared to that of pH 7.0 PB. The degradation rates of pollutants in Yangtze River samples were higher than those in WWTP effluents, implying that lower TOC (4.22 mg L−1 for Yangtze River and 8.07 mg L−1 for WWTP) resulted in less inhibition in contaminant decomposition due to less competitive consumption of ROS. Nevertheless, the results here overall support that solar/PMS treatment performed effectively in degrading pollutants in (wastewater-affected) natural water, although pollutant removal rates could be affected by water quality.
Additional experiments were undertaken to analyze the mineralization rate of organic matter in the two water samples during solar/PMS treatment. The concentration of total organic carbon (TOC) decreased from 9.28 to 7.14 mg L−1 in the Yangtze River sample and from 15.8 to 9.96 mg L−1 in the WWTP sample after 15 min of treatment with mineralization rates calculated to be 23% and 27%, respectively (Fig. S14†), indicating the potential capability of the system for degrading TOC in natural water.
3.5 DBP formation and theoretical cytotoxicity in the oxidation process and subsequent chlorination
Nine typical DBPs (four THMs and five HAAs) during chlorination were selected to evaluate the applicability of solar/PMS as a pre-treatment process prior to chlorine disinfection, with results presented in Tables S5 and S6.† The total concentrations of THMs by chlorination alone were determined to be 6.2, 7.2, and 11.4 μg L−1 at pH 5.5, 7.0, and 8.5, respectively (Fig. S15a†). In the presence of solar/PMS pre-oxidation, total concentrations of THMs increased by 23%, 13%, and 25% to 7.6, 8.2, and 14.2 μg L−1 at pH 5.5, 7.0, and 8.5, respectively, higher than that obtained after the chlorination process alone. Among the four regulated THMs, trichloromethane (CHCl3) accounted for the highest proportion of 53% (5.3 μg L−1) followed by bromodichloromethane (BDCM) for 40% (4.0 μg L−1) at pH 5.5–8.5. Formation of aldehydes and ketones by ˙OH and SO4˙− providing THM precursors was possibly the cause of increased THM production with solar/PMS treatment compared to chlorination alone without pre-oxidation.69 The increased production of total THMs with increasing pH (for both conditions with and without solar/PMS pre-treatment) is consistent with prior findings,70 which may be attributed to base-catalyzed hydrolysis; for example, alkaline conditions could improve trichloromethane production from hydrolysis decomposition of unstable DBPs such as chloral hydrate during chlorination.71 Nevertheless, total concentrations of THMs formed with solar/PMS pre-oxidation at all pH conditions were still lower than the maximum contaminant levels of 80 μg L−1 for THMs regulated by the United States Environmental Protection Agency (USEPA).
During the chlorination alone process without pretreatment, the concentrations of total HAAs raised from 8.0 to 11.9 μg L−1 with pH increasing from 5.5 to 7.0 and decreased to 5.8 μg L−1 at pH 8.5 (Fig. S15b†). The solar/PMS pre-oxidation resulted in substantially greater yields of HAAs of 81.3, 67.2, and 111 μg L−1 at pH 5.5, 7.0, and 8.5, respectively, which were 8.4, 4.3, and 16.7 times of their yields with chlorination alone treatment. In addition, the concentrations of dichloroacetic acid (DCAA) and trichloroacetic acid (TCAA) raised apparently as the major components of HAAs while bromoacetic acid (BAA) and dibromoacetic acid (DBAA) were below the detection limits. At all three pH values, DCAA occupied the highest proportions at pH 5.5 (58.1%) and pH 8.5 (52.7%) while TCAA accounted for the highest percentage at neutral pH (49.6%). In particular, chloroacetic acid (CAA), being more cytotoxic than DCAA and TCAA, was not detected during chlorination treatment alone but formed at concentrations of 4.8–5.8 μg L−1 with pre-oxidation of solar/PMS. This difference could be related to the alteration in structure and content of HAA precursors.72 Although DCAA and TCAA contributed to the excessive yields of HAAs (86.5 μg L−1) at pH 5.5–8.5 with the solar/PMS process, higher than the maximum contaminant levels of total HAAs (<60 μg L−1) regulated by USEPA standard, neither DCAA (44.9 μg L−1) nor TCAA (36.3 μg L−1) levels exceeded domestic drinking water sanitation standards of China (GB 5749-2006; DCAA < 50 μg L−1 and TCAA < 100 μg L−1). Given the contaminant dosage (mg L−1 levels), higher than corresponding concentrations detected in natural water (ng L−1–μg L−1),1,73 HAA yields were relatively under control here.
The increased production of HAAs was probably associated with low TOC mineralization of contaminants. Most pollutants transformed into various intermediate products such as aldehydes, ketones, and carboxylic acids after pre-oxidation treatment,74 which promoted generation of DBPs during chlorination acting as HAA precursors. For example, several intermediates (aromatic and chain byproducts) were identified during ASA degradation, subsequently forming alkyl carboxylic acids with the opening of the benzene ring after UV/O3 treatment.75 During post chlorination, HAAs formed through chlorine substitution and decarboxylation reactions of the above intermediates.76 Even though the target pollutants were efficiently degraded by solar/PMS (up to 35.9–73.3%, 41.7–81.2%, and 51.2–90.7% at pH 5.5, 7.0, and 8.5, respectively) in PB solutions, the low mineralization ratios (2.4%, 3.5%, and 20.0% at pH 5.5, 7.0, and 8.5, respectively) revealed formation of intermediates during the process (Fig. S16†). The highest concentration of nine DBPs in total was determined to be 125.2 μg L−1 at pH 8.5 by chlorination with solar/PMS pre-treatment, which increased by 360% compared to that by chlorination alone. The increase of DBP formation was much lower (increased by 46.2%) during chlorination with LED-UV365/persulfate pre-oxidation for treatment of acetaminophen and phenacetin in a previous study, which could be explained by higher mineralization of acetaminophen and phenacetin (27.5–47.1%) at pH 5.5–8.5 in 60 min.77 Therefore, the increased DBP formation was primarily relevant to inadequate mineralization of organic compounds by solar/PMS, forming intermediates that more readily transformed to DBPs in the subsequent chlorination.
As shown in Fig. 4, according to the concentrations and LC50 values of six monitored DBPs detected in the solar/PMS system, the cytotoxicity of individual DBPs with and without solar/PMS pre-oxidation was calculated. The total toxicity of DBPs increased from 2.16 × 10−5 to 2.02 × 10−4 at pH 5.5, from 3.08 × 10−5 to 1.84 × 10−4 at pH 7.0, and from 1.88 × 10−5 to 2.68 × 10−4 at pH 8.5 by adding solar/PMS pretreatment prior to chlorination. As shown in Fig. S17,† TCAA accounted for the largest percentage of total DBP cytotoxicity without pre-oxidation (69.7%, 69.5%, and 43.3% at pH 5.5, 7.0, and 8.5, respectively), which was followed by CHCl3 (10.5%, 13.0%, 22.7% at pH 5.5, 7.0, and 8.5, respectively). In comparison, after the solar/PMS pre-treatment and subsequent chlorination, the percentage of TCAA toxicity decreased to 36.1–44.7% at pH 5.5–8.5; meanwhile, the cytotoxicity of CAA increased significantly and dominated as the second largest proportion (32.8%) primarily due to its high toxicity (7.05 × 10−5), although its concentration was 1 order of magnitude lower than that of TCAA and DCAA. The experimental result demonstrated that the solar/PMS system led to an apparent increase in the total cytotoxicity of DBPs with a major increase in production of the high-toxicity species such as TCAA, CAA, and DCAA.
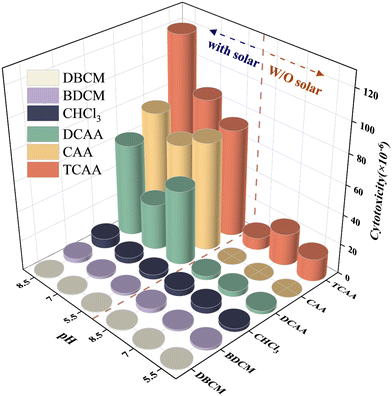 |
| Fig. 4 The calculated cytotoxicity of DBPs with and without (W/O) solar/PMS pre-oxidation in 15 min. Conditions: [NB]0 = 1.22 mg L−1, [FMME]0 = 4.92 mg L−1, [ASA]0 = 1.80 mg L−1, [BA]0 = 1.23 mg L−1, [PMS]0 = 2.0 mM, T = 25 °C, intensity of simulated sunlight = 6.06 W cm−2. | |
Chlorine disinfection is usually applied for drinking water sterilization and efficient for abatement of micro-pollutants, yet, formation of disinfection by-products (DBPs) due to reaction between NOM and chlorine could lead to toxicity during treatment.78,79 Fig. S18† shows variation of DBP production in buffered NOM solution with and without addition of target pollutants by solar/PMS pre-oxidation treatment followed by chlorination at pH 7.0. Through adding solar/PMS pretreatment prior to chlorination, the total THM yields decreased by 16.9% (14.4–11.9 μg L−1) and 11.8% (38.7–34.4 μg L−1) in the NOM only system and NOM + pollutant system, respectively; meanwhile, concentrations of CHCl3 and BDCM (the two most dominant THM species) decreased by 16.8% and 18.2%, respectively, in the absence of pollutants. The yield of total HAAs increased significantly from 23.6 to 73.9 μg L−1 in the NOM only system, while a slight increase (1.78%) was observed in the NOM + pollutant system. Moreover, TCAA and DCAA production (the two most dominant HAA species) increased by 212% and 334%, respectively. The matrices of natural water and wastewater are more complex than that of NOM solution. The difference in variations of THM versus HAA production might come from the fact that AOPs (i.e., UV/H2O2) show a tendency to decrease the aromaticity of NOM, and precursors for THMs and HAAs are aromatic and aliphatic, respectively.80 Similarly, the incomplete mineralization of organic compounds forming intermediates was the primary cause of high HAA levels under conditions with co-existence of NOM and contaminants,81 indicating potential risk for increasing HAA formation during water treatment by the solar/PMS system.
DBP yields were also analyzed for solar/PMS pretreatment followed by chlorination of water samples from Yangtze River and WWTP effluent (Fig. S19†). Typical DBP levels in solar/PMS treatment of Yangtze River water and WWTP effluent without the target pollutants were determined to be 27.9 μg L−1 and 45.5 μg L−1 of THMs (increased by 52% and 110%, respectively, compared to chlorination alone), and 73.4 μg L−1 and 36.0 μg L−1 of HAAs (increased by 235% and 111%, respectively). For all the samples treated by solar/PMS plus post-chlorination, the levels of THMs and HAAs in WWTP effluent with the target pollutants were 86.8 μg L−1 and 115 μg L−1, 143% and 245% higher than that in WWTP effluent alone, and 961% and 71% higher than that in PB systems at pH 7.0, respectively. Furthermore, the solar/PMS pre-oxidation during chlorination of actual waters may facilitate the DBP formation, but variations of specific DBPs (THMs versus HAAs) were not completely consistent with the above conclusion findings for the treatment of phosphate buffered NOM solution with addition of target pollutants. A possible explanation might be that the complex substances in real wastewater contributed to increased concentrations of DBP precursors after pre-oxidation of the solar/PMS system.
In summary, THM yields were effectively controlled in both the NOM only and NOM + pollutant system, whereas HAA yields increased after solar/PMS pretreatment plus post-chlorination compared to chlorination alone. In this study, solar/PMS pre-oxidation generally enhanced the total DBP formation during post-chlorination in reaction matrixes of PB solutions, buffered NOM solutions, and actual waters (surface water and wastewater effluent). Thus, more experiments should be conducted to further evaluate the potential effects of the system.
3.6 Bio-toxicity based on algal growth inhibition
Table S8† shows the biomass production of algae cultured in three systems (pollutants, PMS, solar/PMS-treated pollutants) as well as in the control system (without pollutants or PMS) increased over time (p < 0.05) (all the systems prepared in PB solutions at pH 7.0), which confirms the adaptability of algae in different wastewaters.82 As shown in Table S8,† the addition of pollutants promoted the growth of algae initially after 24 h of incubation, with its density increased by 6.3% in pollutant samples compared to the control group (p < 0.05), but ultimately exerted inhibitory effects on algal growth after 96 h of incubation by 8.5%. This may be ascribed to the low concentration of PPCPs initially serving as the carbon source for the algae to grow.6 With increasing incubation time, NSAIDs presented considerable toxic effects on the photosynthetic system of algae.83 The inhibition of algal growth was observed in systems of PMS and solar/PMS-treated pollutants though the entire culturing period. At the end-point of 96 h culturing, the algae densities in the systems of PMS alone (4.05 × 106 L−1) and solar/PMS-treated pollutants (4.06 × 106 L−1) were significantly lower (p < 0.05) than that in the system of untreated pollutants (5.76 × 106 L−1). Such inhibition of algal growth was likely due to the acute toxicity of residual PMS or degradation products formed by the pollutants in reaction with PMS or radicals in the system.84 In this study, the mean concentration of PMS (2 mM) decreased from 304 to 265 mg L−1 after 15 min of solar/PMS treatment, and the residual PMS was not quenched prior to algal growth assays (Fig. S20†). PMS has been shown to exhibit toxic effects on microalgae, e.g., addition of 0.2 mM PMS resulted in complete inhibition of Chlorella vulgaris growth.85
Concentrations of chlorophyll and carotenoid were also measured during the algal growth assays, as they serve as alternative indicators to evaluate the effects of PPCPs on algal growth.86 As shown in Fig. 5, the contents of chlorophyll a and b decreased by 43.3–48.8% (p < 0.05) in both systems of PMS alone and solar/PMS-treated pollutants compared to that in untreated pollutants. This result has provided additional evidence that exposure to the residual oxidant and/or degradation products caused abatement of chlorophyll, which further led to alteration of photosynthetic capacity.87 A decrease of total carotenoid content was also observed in both PMS alone (21.5%) and solar/PMS treatment (45.9%) systems. In summary, the existence of residual PMS and degradation products after 15 min solar/PMS pre-oxidation revealed potential ecological hazards, which demonstrated the necessity for removal of residual oxidants and degradation products from the finished water before discharge.
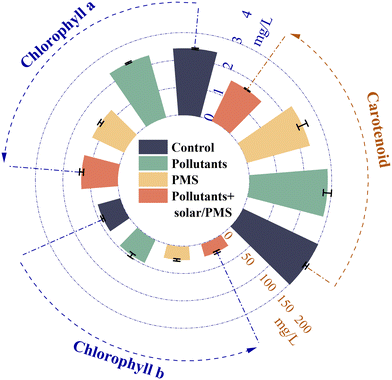 |
| Fig. 5 Determination of chlorophyll a and b (blue), and carotenoid (yellow) at 96 h. Conditions: [NB]0 = 1.22 mg L−1, [FMME]0 = 4.92 mg L−1, [ASA]0 = 1.80 mg L−1, [BA]0 = 1.23 mg L−1, [PMS]0 = 2.0 mM, intensity of simulated sunlight = 6.06 W cm−2, reaction time = 15 min, pH 7.0 phosphate buffer. Error bars represent standard error obtained from triplicate of pigment content. | |
3.7 Energy consumption
Energy consumption in the form of electrical energy per order (EE/O) during solar/PMS treatment of the model contaminants was estimated, and the resulting values of EE/OSolar, EE/OPMS, and EE/Ototal for each contaminant were depicted versus PMS concentrations (0.01–5 mM) in Fig. 6. For each contaminant, addition of PMS led to a slight decrease in EE/OSolar, while EE/OPMS increased linearly with increasing PMS dose, leading to overall V-shape EE/Ototal curves. The EE/Ototal value for degrading ASA reached the minimum (72.0 kW h m−3 order−1) at 0.36 mM PMS, which represented the optimal dosage of PMS added into solutions containing ASA. In the case of low-dose PMS (<0.36 mM), EE/OSolar played a dominant role in the total energy consumption EE/Ototal, while EE/OPMS turned out to be the predominant part of EE/Ototal when high-dose PMS was administered. Similarly, the power consumption of the solar/PMS system for degrading FMME, NB, and BA (>1 mg L−1) presented the same trend as degrading ASA under the same conditions, in which the minimum values of EE/Ototal consumed were calculated to be 79.2, 110, and 106 kW h m−3 order−1, respectively; meanwhile the optimum oxidant dosages corresponding to the minimum EE/Ototal values were 0.38, 0.51, and 0.68 mM, respectively. In this study, the solar/PMS system probably requires relatively high power consumption (72.0–106 kW h m−3 order−1) for removing pollutants (mg L−1), compared to the UV254/H2O2 system, which degraded 90% PPCPs (μg L−1) at pH 7.0 with EE/Ototal ranging from 0.22 to 8.09 kW h m−3 order−1 in wastewater.88 However, the UV254 energy accounts for most of the total costs, while the solar energy could be obtained from sunlight at a much lower cost due to the independence of solar irradiation from electricity.89 A previous study reported the minimum value of EE/Ototal (2.9 kW h m−3 order−1) and corresponding optimal PMS dosages (40 mM) when eliminating dimethyl benzodicarboxylate (100 mg L−1) in the UVC/PMS system,90 and in comparison, the solar/PMS system showed more economical benefits due to lower oxidant dosage (0.36–0.68 mM) required for organic contaminant degradation.
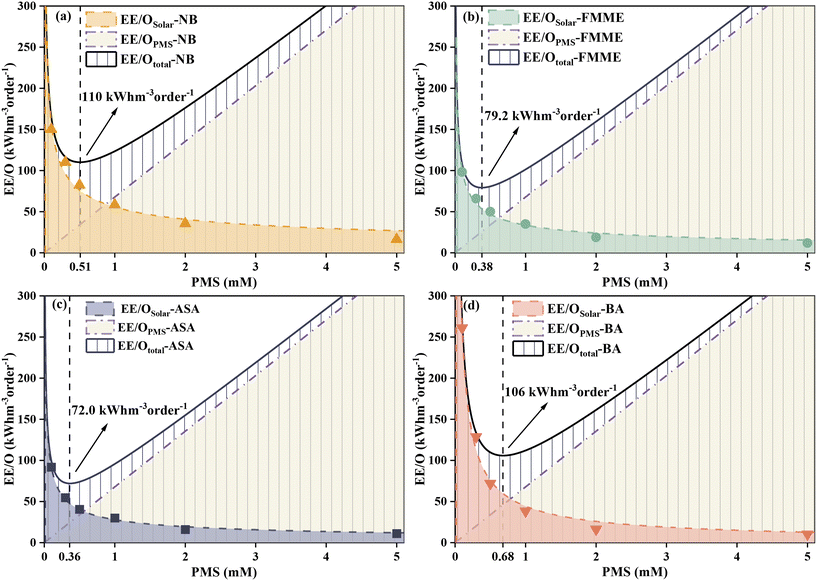 |
| Fig. 6 EE/OSolar, EE/OPMS and EE/Ototal for (a) NB, (b) FMME, (c) ASA, and (d) BA degradation. —▲— EE/OSolar-NB, —●— EE/OSolar-FMME, —■— EE/OSolar-ASA, —▼— EE/OSolar-BA. Conditions: [NB]0 = 1.22 mg L−1, [FMME]0 = 4.92 mg L−1, [ASA]0 = 1.80 mg L−1, [BA]0 = 1.23 mg L−1, pH 7.0 phosphate buffer, [PMS]0 = 0.01–10.0 mM, intensity of simulated sunlight = 6.06 W cm−2, T = 25 °C. | |
4. Conclusions
During solar/PMS treatment, kobs values for degradation of model pollutants NB, FMME, ASA, and BA increased with increasing pH and reached maximums at pH 8.5. Alkaline conditions significantly promoted the corresponding degradation efficiencies to 51.2%, 68.0%, 66.6%, and 90.7% after 15 min, respectively. SO4˙− made major contribution to the removal of ASA (42.4–60.0%) and FMME (49.5–56.3%), followed by ˙OH (29.4–37.2% for ASA and 40.6–49.1% for FMME) at pH 5.5–8.5.
In the investigation of DBP formation after solar/PMS pre-oxidation plus chlorination, the formation of THMs and HAAs in natural water increased obviously compared to that by chlorination alone. Meanwhile, the growth of algae after 96-h incubation was inhibited by 35.6% due to the presence of residual PMS and degradation products after solar/PMS pretreatment. Hence, in view of toxicity, further work should be conducted to explore the removal of residual oxidant and hazardous (by-)products after solar/PMS treatment and evaluate the practical applications of this technique.
Conflicts of interest
There are no conflicts to declare.
Acknowledgements
This work was financially supported by the National Natural Science Foundation of China (No.52070041), Natural Science Foundation of Jiangsu Province (No. BK20211175), National Key Research and Development Program of China (No.2019YFD1100303), the Priority Academic Program Development of Jiangsu Higher Education Institutions, and the Fundamental Research Funds for the Central Universities, “Zhishan” Scholars Programs of Southeast University.
References
- A. Rastogi, M. K. Tiwari and M. M. Ghangrekar, A review on environmental occurrence, toxicity and microbial degradation of Non-Steroidal Anti-Inflammatory Drugs (NSAIDs), J. Environ. Manage., 2021, 300, 113694 CrossRef CAS PubMed.
- Y. Zhou, J. Meng, M. Zhang, S. Chen, B. He, H. Zhao, Q. Li, S. Zhang and T. Wang, Which type of pollutants need to be controlled with priority in wastewater treatment plants: Traditional or emerging pollutants?, Environ. Int., 2019, 131, 104982 CrossRef CAS PubMed.
- M. Huerta-Fontela, M. Teresa Galceran and F. Ventura, Occurrence and removal of pharmaceuticals and hormones through drinking water treatment, Water Res., 2011, 45, 1432–1442 CrossRef CAS PubMed.
- Y. Yang, Y. S. Ok, K. H. Kim, E. E. Kwon and Y. F. Tsang, Occurrences and removal of pharmaceuticals and personal care products (PPCPs) in drinking water and water/sewage treatment plants: A review, Sci. Total Environ., 2017, 596-597, 303–320 CrossRef CAS PubMed.
- I. Sanchez-Montes, N. Wachter, B. F. Silva and J. M. Aquino, Comparison of UVC-based advanced oxidation processes in the mineralization of bisphenol A: Identi fication of oxidation by products and toxicity evaluation, Chem. Eng. J., 2020, 386, 123986 CrossRef CAS.
- J. Trawinski, P. Szpot, M. Zawadzki and R. Skibinski, Photochemical transformation of fentanyl under the simulated solar radiation - Enhancement of the process by heterogeneous photocatalysis and in silico analysis of toxicity, Sci. Total Environ., 2021, 791, 148171 CrossRef CAS PubMed.
- C. Amor, E. De Torres-Socias, J. A. Peres, M. I. Maldonado, I. Oller, S. Malato and M. S. Lucas, Mature landfill leachate treatment by coagulation/flocculation combined with Fenton and solar photo-Fenton processes, J. Hazard. Mater., 2015, 286, 261–268 CrossRef CAS PubMed.
- Q. Yang, Y. Ma, F. Chen, F. Yao, J. Sun, S. Wang, K. Yi, L. Hou, X. Li and D. Wang, Recent advances in photo-activated sulfate radical-advanced oxidation process (SR-AOP) for refractory organic pollutants removal in water, Chem. Eng. J., 2019, 378, 122149 CrossRef CAS.
- J. Wang and S. Wang, Activation of persulfate (PS) and peroxymonosulfate (PMS) and application for the degradation of emerging contaminants, Chem. Eng. J., 2018, 334, 1502–1517 CrossRef CAS.
- S. Waclawek, H. V. Lutze, K. Grubel, V. V. T. Padil, M. Cernik and D. D. Dionysiou, Chemistry of persulfates in water and wastewater treatment: A review, Chem. Eng. J., 2017, 330, 44–62 CrossRef CAS.
- R. Yin, W. Guo, H. Wang, J. Du, X. Zhou, Q. Wu, H. Zheng, J. Chang and N. Ren, Selective degradation of sulfonamide antibiotics by peroxymonosulfate alone: Direct oxidation and nonradical mechanisms, Chem. Eng. J., 2018, 334, 2539–2546 CrossRef CAS.
- X. Liu, T. Zhang, Y. Zhou, L. Fang and Y. Shao, Degradation of atenolol by UV/peroxymonosulfate: Kinetics, effect of operational parameters and mechanism, Chemosphere, 2013, 93, 2717–2724 CrossRef CAS PubMed.
- X. Xu, G. Pliego, C. Alonso, S. Liu, L. Nozal and J. J. Rodriguez, Reaction pathways of heat-activated persulfate oxidation of naphthenic acids in the presence and absence of dissolved oxygen in water, Chem. Eng. J., 2019, 370, 695–705 CrossRef CAS.
- C. Alexopoulou, A. Petala, Z. Frontistis, C. Drivas, S. Kennou, D. I. Kondarides and D. Mantzavinos, Copper phosphide and persulfate salt: A novel catalytic system for the degradation of aqueous phase micro-contaminants, Appl. Catal., B, 2019, 244, 178–187 CrossRef CAS.
- C. Guan, J. Jiang, S. Pang, J. Ma, X. Chen and T.-T. Lim, Nonradical transformation of sulfamethoxazole by carbon nanotube activated peroxydisulfate: Kinetics, mechanism and product toxicity, Chem. Eng. J., 2019, 378, 122147 CrossRef CAS.
- Y. Gao, N. Gao, W. Chu, Y. Zhang, J. Zhang and D. Yin, UV-activated persulfate oxidation of sulfamethoxypyridazine: Kinetics, degradation pathways and impact on DBP formation during subsequent chlorination, Chem. Eng. J., 2019, 370, 706–715 CrossRef CAS.
- J. Rodriguez-Chueca, S. Giannakis, M. Marjanovic, M. Kohantorabi, M. R. Gholami, D. Grandjean, L. F. de Alencastro and C. Pulgarin, Solar-assisted bacterial disinfection and removal of contaminants of emerging concern by Fe2+-activated HSO5− vs. S2O82− in drinking water, Appl. Catal., B, 2019, 248, 62–72 CrossRef CAS.
- S. Guerra-Rodríguez, R. Encarnación, D. N. Singh and J. Rodríguez-Chueca, Assessment of sulfate radical-based advanced oxidation processes for water and wastewater treatment: a review, Water, 2018, 10, 1828 CrossRef.
- Y. Pang, J. Zhang, R. Ma, Z. Qu, E. Lee and T. Luo, Solar-Thermal Water Evaporation: A Review, ACS Energy Lett., 2020, 5, 437–456 CrossRef CAS.
- W. Jessen, S. Wilbert, C. A. Gueymard, J. Polo, Z. Bian, A. Driesse, A. Habte, A. Marzo, P. R. Armstrong, F. Vignola and L. Ramírez, Proposal and evaluation of subordinate standard solar irradiance spectra for applications in solar energy systems, Sol. Energy, 2018, 168, 30–43 CrossRef.
- I. Berruti, S. Nahim-Granados, M. J. Abeledo-Lameiro, I. Oller and M. I. Polo-López, Recent advances in solar photochemical processes for water and wastewater disinfection, Chem. Eng. J. Adv., 2022, 10, 100248 CrossRef CAS.
- R. G. Zepp, T. V. Callaghan and D. J. Erickson, Effects of increased solar ultraviolet radiation on biogeochemical cycles, Ambio, 1995, 24, 181–187 Search PubMed.
- B. Yang, R. S. Kookana, M. Williams, J. Du, H. Doan and A. Kumar, Removal of carbamazepine in aqueous solutions through solar photolysis of free available chlorine, Water Res., 2016, 100, 413–420 CrossRef CAS PubMed.
- A. Serra-Clusellas, L. De Angelis, C. H. Lin, P. Vo, M. Bayati, L. Sumner, Z. Lei, N. B. Amaral, L. M. Bertini, J. Mazza, L. R. Pizzio, J. D. Stripeikis, J. A. Rengifo-Herrera and M. M. Fidalgo de Cortalezzi, Abatement of 2,4-D by H2O2 solar photolysis and solar photo-Fenton-like process with minute Fe(III) concentrations, Water Res., 2018, 144, 572–580 CrossRef CAS PubMed.
- L. Rizzo, T. Agovino, S. Nahim-Granados, M. Castro-Alférez, P. Fernández-Ibáñez and M. I. Polo-López, Tertiary treatment of urban wastewater by solar and UV-C driven advanced oxidation with peracetic acid: Effect on contaminants of emerging concern and antibiotic resistance, Water Res., 2019, 149, 272–281 CrossRef CAS PubMed.
- L. W. Matzek and K. E. Carter, Activated persulfate for organic chemical degradation: A review, Chemosphere, 2016, 151, 178–188 CrossRef CAS PubMed.
- I. Berruti, I. Oller and M. I. Polo-Lopez, Direct oxidation of peroxymonosulfate under natural solar radiation: Accelerating the simultaneous removal of organic contaminants and pathogens from water, Chemosphere, 2021, 279, 130555 CrossRef CAS PubMed.
- R. R. Solis, F. J. Rivas, A. M. Chavez and D. D. Dionysiou, Simulated solar photo-assisted decomposition of peroxymonosulfate. Radiation filtering and operational variables influence on the oxidation of aqueous bezafibrate, Water Res., 2019, 162, 383–393 CrossRef CAS PubMed.
- M. Mahdi-Ahmed and S. Chiron, Ciprofloxacin oxidation by UV-C activated peroxymonosulfate in wastewater, J. Hazard. Mater., 2014, 265, 41–46 CrossRef CAS PubMed.
- G. P. Anipsitakis, T. P. Tufano and D. D. Dionysiou, Chemical and microbial decontamination of pool water using activated potassium peroxymonosulfate, Water Res., 2008, 42, 2899–2910 CrossRef CAS PubMed.
- S. Guerra-Rodríguez, A. R. L. Ribeiro, R. S. Ribeiro, E. Rodríguez, A. M. T. Silva and J. Rodríguez-Chueca, UV-A activation of peroxymonosulfate for the removal of micropollutants from secondary treated wastewater, Sci. Total Environ., 2021, 770, 145299 CrossRef PubMed.
- R. R. Solís, F. J. Rivas, A. M. Chávez and D. D. Dionysiou, Peroxymonosulfate/solar radiation process for the removal of aqueous microcontaminants. Kinetic modeling, influence of variables and matrix constituents, J. Hazard. Mater., 2020, 400, 123118 CrossRef PubMed.
- H. Wang, H. Xi, L. Xu, M. Jin, W. Zhao and H. Liu, Ecotoxicological effects, environmental fate and risks of pharmaceutical and personal care products in the water environment: A review, Sci. Total Environ., 2021, 788, 147819 CrossRef CAS PubMed.
- F. O. Agunbiade and B. Moodley, Occurrence and distribution pattern of acidic pharmaceuticals in surface water, wastewater, and sediment of the Msunduzi River, Kwazulu-Natal, South Africa, Environ. Toxicol. Chem., 2016, 35, 36–46 CrossRef CAS PubMed.
- T. Wei, M. Waqas, K. Xiao, B. Yang, Y. Luo, Q. Luo, J. Zhang, M. Wang, C. Zhu, T. He and Z. Lu, Effective degradation of refractory nitrobenzene in water by the natural 4-hydroxycoumarin under solar illumination, Chemosphere, 2019, 215, 199–205 CrossRef CAS PubMed.
- V. E. Sugihartono, N. N. N. Mahasti, Y.-J. Shih and Y.-H. Huang, Photo-persulfate oxidation and mineralization of benzoic acid: Kinetics and optimization under UVC irradiation, Chemosphere, 2022, 296, 133663 CrossRef CAS PubMed.
- C. Tan, H. Zhao, X. Wang, H. Yu, S. Chong, Y. Xu, E. Du, M. Chen, X. Peng and L. Su, Feasibility of micropollutants removal by solar-activated persulfate: Reactive oxygen species formation and influence on DBPs, Water Res., 2022, 210, 117981 CrossRef CAS PubMed.
- Z. Hua, D. Li, Z. Wu, D. Wang, Y. Cui, X. Huang, J. Fang and T. An, DBP formation and toxicity alteration during UV/chlorine treatment of wastewater and the effects of ammonia and bromide, Water Res., 2021, 188, 116549 CrossRef CAS PubMed.
- N. Yan, H. Zhong and M. L. Brusseau, The natural activation ability of subsurface media to promote in-situ chemical oxidation of 1,4-dioxane, Water Res., 2019, 149, 386–393 CrossRef CAS PubMed.
- Y. Lee, J. Yoon and U. von Gunten, Spectrophotometric determination of ferrate (Fe(VI)) in water by ABTS, Water Res., 2005, 39, 1946–1953 CrossRef CAS PubMed.
- Y. Xiao, L. Zhang, J. Yue, R. D. Webster and T.-T. Lim, Kinetic modeling and energy efficiency of UV/H2O2 treatment of iodinated trihalomethanes, Water Res., 2015, 75, 259–269 CrossRef CAS PubMed.
- Y. H. Chuang, A. Szczuka, F. Shabani, J. Munoz, R. Aflaki, S. D. Hammond and W. A. Mitch, Pilot-scale comparison of microfiltration/reverse osmosis and ozone/biological activated carbon with UV/hydrogen peroxide or UV/free chlorine AOP treatment for controlling disinfection byproducts during wastewater reuse, Water Res., 2019, 152, 215–225 CrossRef CAS PubMed.
- S. O. Ganiyu, M. Arslan and M. Gamal El-Din, Combined solar activated sulfate radical-based advanced oxidation processes (SR-AOPs) and biofiltration for the remediation of dissolved organics in oil sands produced water, Chem. Eng. J., 2022, 433, 134579 CrossRef CAS.
- R. R. Solís, F. J. Rivas, A. M. Chávez and D. D. Dionysiou, Simulated solar photo-assisted decomposition of peroxymonosulfate. Radiation filtering and operational variables influence on the oxidation of aqueous bezafibrate, Water Res., 2019, 162, 383–393 CrossRef PubMed.
- K. Kusano and I. Wadso, Enthalpy of vaporization of some organic substances at 25.0 °C and test of calorimeter, Bull. Chem. Soc. Jpn., 1971, 44, 1705–1707 CrossRef CAS.
- C. Xu and S. Li, Analysis of the CPMV index for evaluating indoor thermal comfort in southern China in summer, a case study in Nanjing, Front. Archit. Res., 2022, 11, 103–113 CrossRef.
- C. Qi, X. Liu, J. Ma, C. Lin, X. Li and H. Zhang, Activation of peroxymonosulfate by base: Implications for the degradation of organic pollutants, Chemosphere, 2016, 151, 280–288 CrossRef CAS PubMed.
- J. Sharma, I. M. Mishra, D. D. Dionysiou and V. Kumar, Oxidative removal of Bisphenol A by UV-C/peroxymonosulfate (PMS): Kinetics, influence of co-existing chemicals and degradation pathway, Chem. Eng. J., 2015, 276, 193–204 CrossRef CAS.
- S. K. Rani, D. Easwaramoorthy, I. M. Bilal and M. Palanichamy, Studies on Mn(II)-catalyzed oxidation of α-amino acids by peroxomonosulphate in alkaline medium-deamination and decarboxylation: A kinetic approach, Appl. Catal., A, 2009, 369, 1–7 CrossRef CAS.
- Y. Guan, J. Ma, X. Li, J. Fang and L. Chen, Influence of pH on the formation of sulfate and hydroxyl radicals in the UV/peroxymonosulfate system, Environ. Sci. Technol., 2011, 45, 9308–9314 CrossRef CAS PubMed.
- M. Muzolf, H. Szymusiak, A. Gliszczyńska-Świgło, I. M. C. M. Rietjens and B. E. Tyrakowska, pH-Dependent Radical Scavenging Capacity of Green Tea Catechins, J. Agric. Food Chem., 2008, 56, 816–823 CrossRef CAS PubMed.
- A. Sennaoui, S. Alahiane, F. Sakr, M. Tamimi, E. H. Ait Addi, M. Hamdani and A. Assabbane, Comparative degradation of benzoic acid and its hydroxylated derivatives by electro-Fenton technology using BDD/carbon-felt cells, J. Environ. Chem. Eng., 2019, 7, 103033 CrossRef CAS.
- C. Qi, X. Liu, C. Lin, X. Zhang, J. Ma, H. Tan and W. Ye, Degradation of sulfamethoxazole by microwave-activated persulfate: Kinetics, mechanism and acute toxicity, Chem. Eng. J., 2014, 249, 6–14 CrossRef CAS.
- P. Duan, X. Liu, B. Liu, M. Akram, Y. Li, J. Pan, Q. Yue, B. Gao and X. Xu, Effect of phosphate on peroxymonosulfate activation: Accelerating generation of sulfate radical and underlying mechanism, Appl. Catal., B, 2021, 298, 120532 CrossRef CAS.
- X. Lou, L. Wu, Y. Guo, C. Chen, Z. Wang, D. Xiao, C. Fang, J. Liu, J. Zhao and S. Lu, Peroxymonosulfate activation by phosphate anion for organics degradation in water, Chemosphere, 2014, 117, 582–585 CrossRef CAS PubMed.
- K. D. Asmus, B. Cercek, M. Ebert, A. Henglein and A. Wigger, Pulse radiolysis of nitrobenzene solutions, Trans. Faraday Soc., 1967, 63, 2435–2441 RSC.
- P. Neta, R. E. Huie and A. B. Ross, Rate constants for reactions of inorganic radicals in aqueous solution, J. Phys. Chem. Ref. Data, 1988, 17, 1027–1284 CrossRef CAS.
- G. V. Buxton, C. L. Greenstock, W. P. Helman and A. B. Ross, Critical review of rate constants for reactions of hydrated electrons, hydrogen atoms and hydroxyl radicals (.OH/.O−) in aqueous solution, J. Phys. Chem. Ref. Data, 1988, 17, 513–886 CrossRef CAS.
- S. Giannakis, K.-Y. A. Lin and F. Ghanbari, A review of the recent advances on the treatment of industrial wastewaters by Sulfate Radical-based Advanced Oxidation Processes (SR-AOPs), Chem. Eng. J., 2021, 406, 127083 CrossRef CAS.
- M. Lee, W. Wang, Q. Wu, N. Huang, Z. Xu and H. Hu, Degradation of dodecyl dimethyl benzyl ammonium chloride (DDBAC) as a non-oxidizing biocide in reverse osmosis system using UV/persulfate: Kinetics, degradation pathways, and toxicity evaluation, Chem. Eng. J., 2018, 352, 283–292 CrossRef CAS.
- B. Huang, Z. Xiong, P. Zhou, H. Zhang, Z. Pan, G. Yao and B. Lai, Ultrafast degradation of contaminants in a trace cobalt(II) activated peroxymonosulfate process triggered through borate: Indispensable role of intermediate complex, J. Hazard. Mater., 2022, 424, 127641 CrossRef CAS PubMed.
- W. Oh, Z. Dong and T.-T. Lim, Generation of sulfate radical through heterogeneous catalysis for organic contaminants removal: Current development, challenges and prospects, Appl. Catal., B, 2016, 194, 169–201 CrossRef CAS.
- X. Zhang, J. Yao, Z. Zhao and J. Liu, Degradation of haloacetonitriles with UV/peroxymonosulfate process: Degradation pathway and the role of hydroxyl radicals, Chem. Eng. J., 2019, 364, 1–10 CrossRef CAS.
- J. Ding, H. Nie, S. Wang, Y. Chen, Y. Wan, J. Wang, H. Xiao, S. Yue, J. Ma and P. Xie, Transformation of acetaminophen in solution containing both peroxymonosulfate and chlorine: Performance, mechanism, and disinfection by-product formation, Water Res., 2021, 189, 116605 CrossRef CAS PubMed.
- D. Awfa, M. Ateia, M. Fujii and C. Yoshimura, Photocatalytic degradation of organic micropollutants: Inhibition mechanisms by different fractions of natural organic matter, Water Res., 2020, 174, 115643 CrossRef CAS PubMed.
- Y. Ye, H. Bruning, W. Liu, H. Rijnaarts and D. Yntema, Effect of dissolved natural organic matter on the photocatalytic micropollutant removal performance of TiO2 nanotube array, J. Photochem. Photobiol., A, 2019, 371, 216–222 CrossRef CAS.
- J. Brame, M. Long, Q. Li and P. Alvarez, Trading oxidation power for efficiency: Differential inhibition of photo-generated hydroxyl radicals versus singlet oxygen, Water Res., 2014, 60, 259–266 CrossRef CAS PubMed.
- D. Vione, M. Minella, V. Maurino and C. Minero, Indirect Photochemistry in Sunlit Surface Waters: Photoinduced Production of Reactive Transient Species, Chem. – Eur. J., 2014, 20, 10590–10606 CrossRef CAS PubMed.
- P. Xie, J. Ma, W. Liu, J. Zou and S. Yue, Impact of UV/persulfate pretreatment on the formation of disinfection byproducts during subsequent chlorination of natural organic matter, Chem. Eng. J., 2015, 269, 203–211 CrossRef CAS.
- W. Chu, D. Yao, Y. Deng, M. Sui and N. Gao, Production of trihalomethanes, haloacetaldehydes and haloacetonitriles during chlorination of microcystin-LR and impacts of pre-oxidation on their formation, J. Hazard. Mater., 2017, 327, 153–160 CrossRef CAS PubMed.
- J. Fang, J. Ma, X. Yang and C. Shang, Formation of carbonaceous and nitrogenous disinfection by-products from the chlorination of Microcystis aeruginosa, Water Res., 2010, 44, 1934–1940 CrossRef CAS PubMed.
- Z. Hua, X. Kong, S. Hou, S. Zou, X. Xu, H. Huang and J. Fang, DBP alteration from NOM and model compounds after UV/persulfate treatment with post chlorination, Water Res., 2019, 158, 237–245 CrossRef CAS PubMed.
- D. Simazaki, R. Kubota, T. Suzuki, M. Akiba, T. Nishimura and S. Kunikane, Occurrence of selected pharmaceuticals at drinking water purification plants in Japan and implications for human health, Water Res., 2015, 76, 187–200 CrossRef CAS PubMed.
- C. Liu, X. Tang, J. Kim and G. V. Korshin, Formation of aldehydes and carboxylic acids in ozonated surface water and wastewater: A clear relationship with fluorescence changes, Chemosphere, 2015, 125, 182–190 CrossRef CAS PubMed.
- W. Zhe, Z. Wenjuan, W. Haihan, W. Zhiwei and C. Jing, Oxidation of acetylsalicylic acid in water by UV/O3 process: Removal, byproduct analysis, and investigation of degradation mechanism and pathway, J. Environ. Chem. Eng., 2021, 9, 106259 CrossRef CAS.
- W. Chu, N. Gao, S. W. Krasner, M. R. Templeton and D. Yin, Formation of halogenated C-, N-DBPs from chlor(am)ination and UV irradiation of tyrosine in drinking water, Environ. Pollut., 2012, 161, 8–14 CrossRef CAS PubMed.
- C. Tan, X. Jian, L. Su, X. Lu, J. Huang, J. Deng and W. Chu, Kinetic removal of acetaminophen and phenacetin during LED-UV365 photolysis of persulfate system: Reactive oxygen species generation, Chemosphere, 2021, 269, 129337 CrossRef CAS PubMed.
- S. Ding, W. Chu, T. Bond, Q. Wang, N. Gao, B. Xu and E. Du, Formation and estimated toxicity of trihalomethanes, haloacetonitriles, and haloacetamides from the chlor(am)ination of acetaminophen, J. Hazard. Mater., 2018, 341, 112–119 CrossRef PubMed.
- E. Du, J. Li, S. Zhou, L. Zheng and X. Fan, Transformation of naproxen during the chlorination process: Products identification and quantum chemistry validation, Chemosphere, 2018, 211, 1007–1017 CrossRef CAS PubMed.
- R. Lamsal, M. E. Walsh and G. A. Gagnon, Comparison of advanced oxidation processes for the removal of natural organic matter, Water Res., 2011, 45, 3263–3269 CrossRef CAS PubMed.
- T. Bond, E. H. Goslan, B. Jefferson, F. Roddick, L. Fan and S. A. Parsons, Chemical and biological oxidation of NOM surrogates and effect on HAA formation, Water Res., 2009, 43, 2615–2622 CrossRef CAS PubMed.
- E. Villar-Navarro, R. M. Baena-Nogueras, M. Paniw, J. A. Perales and P. A. Lara-Martín, Removal of pharmaceuticals in urban wastewater: High rate algae pond (HRAP) based technologies as an alternative to activated sludge based processes, Water Res., 2018, 139, 19–29 CrossRef CAS PubMed.
- H. Wang, M. Jin, W. Mao, C. Chen, L. Fu, Z. Li, S. Du and H. Liu, Photosynthetic toxicity of non-steroidal anti-inflammatory drugs (NSAIDs) on green algae Scenedesmus obliquus, Sci. Total Environ., 2020, 707, 136176 CrossRef CAS PubMed.
- X. Wang, X. Wang, J. Zhao, J. Song, C. Su and Z. Wang, Surface modified TiO2 floating photocatalyst with PDDA for efficient adsorption and photocatalytic inactivation of Microcystis aeruginosa, Water Res., 2018, 131, 320–333 CrossRef CAS PubMed.
- Y. Rao, F. Han, Q. Chen, D. Wang, D. Xue, H. Wang and S. Pu, Efficient degradation of diclofenac by LaFeO3-Catalyzed peroxymonosulfate oxidation---kinetics and toxicity assessment, Chemosphere, 2019, 218, 299–307 CrossRef CAS PubMed.
- T. Ding, M. Yang, J. Zhang, B. Yang, K. Lin, J. Li and J. Gan, Toxicity, degradation and metabolic fate of ibuprofen on freshwater diatom Navicula sp, J. Hazard. Mater., 2017, 330, 127–134 CrossRef CAS PubMed.
- I. Moro, V. Matozzo, A. Piovan, E. Moschin and F. D. Vecchia, Morpho-physiological effects of ibuprofen on Scenedesmus rubescens, Environ. Toxicol. Pharmacol., 2014, 38, 379–387 CrossRef CAS PubMed.
- K. Guo, Z. Wu, S. Yan, B. Yao, W. Song, Z. Hua, X. Zhang, X. Kong, X. Li and J. Fang, Comparison of the UV/chlorine and UV/H2O2 processes in the degradation of PPCPs in simulated drinking water and wastewater: Kinetics, radical mechanism and energy requirements, Water Res., 2018, 147, 184–194 CrossRef CAS PubMed.
- J. Rodríguez-Chueca, S. Giannakis, M. Marjanovic, M. Kohantorabi, M. R. Gholami, D. Grandjean, L. F. de Alencastro and C. Pulgarín, Solar-assisted bacterial disinfection and removal of contaminants of emerging concern by Fe2+-activated HSO5− vs. S2O82− in drinking water, Appl. Catal., B, 2019, 248, 62–72 CrossRef.
- J. Macías-Sánchez, L. Hinojosa-Reyes, J. L. Guzmán-Mar, J. M. Peralta-Hernández and A. Hernández-Ramírez, Performance of the photo-Fenton process in the degradation of a model azo dye mixture, Photochem. Photobiol. Sci., 2011, 10, 332–337 CrossRef PubMed.
|
This journal is © The Royal Society of Chemistry 2023 |
Click here to see how this site uses Cookies. View our privacy policy here.