DOI:
10.1039/D2FO02914F
(Paper)
Food Funct., 2023,
14, 112-121
Anti-inflammatory potential of digested Brassica sprout extracts in human macrophage-like HL-60 cells†
Received
29th September 2022
, Accepted 1st December 2022
First published on 2nd December 2022
Abstract
Cruciferous vegetables have been reported to be a great source of anti-inflammatory compounds. Specifically, sprouts from the Brassicaceae family stand out for their high content of glucosinolates (and their bioactive derivatives, isothiocyanates), phenolic acids, and anthocyanins. Despite the evident anti-inflammatory activity of certain Brassica phytochemicals such as sulforaphane or phenolic acids, the effect of digested Brassica vegetables on inflammation remains understudied. In this work, we aimed to evaluate the anti-inflammatory potential of the bioaccessible forms of cruciferous bioactives (from red cabbage sprouts (RCS) and red radish sprouts (RRS)) obtained upon in vitro gastrointestinal digestion in the HL-60 macrophage-like differentiated human cell line. The study was performed under basal conditions or stimulated with a low dose of LPS for 24 hours as a validated in vitro model of chronic inflammation. The cell viability was determined by MTT assay. The gene expression and production of pro-inflammatory cytokines TNF-α, IL-6 and IL-1β were determined by RT-qPCR and ELISA respectively. Our results revealed no cytotoxicity with any of the treatments in LPS-stimulated macrophage-like HL60 cells. Regarding cytokine production, digestates significantly decreased the production of the three pro-inflammatory cytokines at concentrations of 50 and 100 μg mL−1 except for IL-1β treated with RCS digestates. Furthermore, the RT-qPCR analysis showed a decrease in the relative expression of pro-inflammatory cytokines in LPS-stimulated cells treated with RRS digestates at 100 μg mL−1 but not with red cabbage digestates. In conclusion, RRS bioaccessible compounds in the extracts could be used as dietary coadjuvants given their potential anti-inflammatory effect on this in vitro model of chronic inflammation.
1. Introduction
Nowadays, it is widely accepted that the dietary pattern is a key factor in improving human health. A “healthy diet” is defined as one with a high percentage of vegetables and fiber, an adequate content of proteins, and rich in vitamins and micronutrients.1 In that sense, in the last few decades a high research effort has been exerted to demonstrate the beneficial health effects of bioactive compounds present in diverse types of foods, with special attention to those coming from fruits and vegetables. Among them, cruciferous vegetables stand out not only for their nutritional value but also for their diverse content of a wide range of bioactive compounds, such as flavonols, anthocyanins and glucosinolates (GSLs). Furthermore, the dietary intake of these molecules has been related to a reduction in the incidence of non-communicable diseases related to inflammatory processes.2
Due to their short time of production and high concentration of health-promoting biomolecules, cruciferous sprouts have emerged as a great option for incorporation in the daily diet.3 Sprouts obtained from Brassicaceae vegetables are one of the main sources of GSLs, which are the main secondary metabolites present in Brassicaceae species. These molecules present a β-thioglucoside-N-hydrosulfate bound to a sulfur β-glucopyranose with a variable side chain depending on the precursor amino acid.4 However, the bioactivity of GSLs depends on their hydrolysis products after catalyzation by the myrosinase, mainly isothiocyanates (ITCs) and indoles.5 Certain Brassica sprouts, such as broccoli, contain high levels of glucoraphanin, of which the corresponding ITC is sulforaphane (SFN). SFN has been widely studied for its anti-tumorigenic, anti-oxidative and anti-inflammatory properties.6 In addition, the ITC iberin, derived from the glucoiberin present in some sprouts (e.g. red cabbage), has been also reported to be involved in the induction of several anti-inflammatory pathways.7 Glucobrassicin (GBS) is also an abundant indolic GSL present in Brassicaceae, and it mainly produces indole-3-carbinol (I3C). However, I3C usually undergoes dimeric condensation, giving 3,3-diindolylmethane (DIM).8 Both molecules have been demonstrated to be agonists of the aryl hydrocarbon receptor (AhR), and therefore increase the rate of detoxification pathways.9
Other colored Brassicaceae sprouts, such as red radish, are especially rich in anthocyanins. These compounds belong to the family of flavonoids, showing a C6–C3–C6 carbon skeleton and cyanidin, and they are frequently present in highly glycosylated and acylated forms in Brassicaceae sprouts, particularly in red radish varieties.10 The interest in anthocyanins has recently increased not only because of their anti-oxidant properties, but also due to their role in reducing the risk of renal and hepatic damage caused by oxidative stress conditions.11,12
In recent decades, low-grade inflammatory processes have been highly related to non-communicable diseases, such as obesity, diabetes and cancer.13,14 Inflammation is a coordinated process performed by the immune system, whose main function is to maintain the organism's homeostasis. This process is triggered by both foreign agents and endogenous signals of tissue damage, thus playing a key role in tissue repair.15 Within this process, a broad variety of molecular mediators are involved, such as pro-inflammatory cytokines such as interleukin 6 (IL-6), interleukin 1 beta (IL-1β) and tumor necrosis factor alpha (TNF-α), which are produced to a large extent by macrophages.16 It is noteworthy that an inflammatory process can lead to chronic inflammation if pathogenic agents or tissue damage signals are persistent and the acute inflammation is not correctly resolved. Sustained in time, chronic inflammation stages often are related to non-communicable diseases like type 2 diabetes and cardiovascular and neurodegenerative diseases, among others.17 Thus, understanding the physiopathology of these types of long-term inflammatory processes is of a great importance for the identification of new targets and strategies against these chronic inflammatory-related diseases.
Currently, the main therapeutic options to treat the above-mentioned inflammatory diseases are non-steroidal anti-inflammatory drugs (NSAIDs), since they act by blocking cyclooxygenases implicated in the synthesis of inflammatory mediators, like prostaglandins.18 However, NSAIDs present relevant adverse effects, which include cardiovascular, renal, and gastrointestinal toxicity. As a consequence, the need for new strategies and therapeutic agents that could ameliorate the inflammatory condition without these deleterious effects has become a major research challenge.19,20 In this scenario, compounds derived from cruciferous vegetables have emerged as a possible alternative, either as a drug on their own or as a possible nutritional intervention option. For example, SFN has been described to induce the Nrf2 transcription factor, which is implicated in the transcription of diverse genes associated with the anti-inflammatory response.21 Furthermore, in vivo studies conducted on 40 overweight subjects who consumed 30 g of broccoli sprouts per day in their diet for 10 weeks showed a decrease in IL-6 levels.22 In this line, we previously reported that in macrophage-like HL-60 cells several cruciferous-derived compounds exhibited potential anti-inflammatory activity without showing any cytotoxic effect.23 Nevertheless, the concentrations used in most in vitro assays often differ from the actual concentrations of these compounds that can be found in the circulation after intestinal digestion.24 Thus, the aim of this work was to assess the anti-inflammatory and cytotoxic effect of the resulting product of an in vitro digestion process (digestates) of red radish sprout (RRS) and red cabbage sprout (RCS) extracts in a human macrophage-like cell model derived from HL-60.
2. Materials and methods
2.1. Plant material
A total of 50 g of seeds from red cabbage (Brassica oleracea L. var. capitata f. rubra) and red radish (Raphanus sativus L. var. sativus L. cv. Sango), provided by Intersemillas S.A. (Loriguilla, Valencia, Spain), were induced to germinate after decontamination in 1% bleach in de-ionized water followed by 24 hours of aeration. Subsequently, they were sown on an inert substrate of cellulose and kept in darkness and high relative humidity for 48 hours. Three-day-old germinated seeds were transferred to a growth chamber to grow the sprouts under a 20 W Protect BioLED (SysLed Spain, S.L.) photoperiod of 18 hours light/6 hours darkness, a temperature of 24 °C/18 °C, and a relative humidity of 60%/80%. Once collected, the 8-day sprouts were freeze-dried and ground prior to analysis. The resulting freeze-dried powder was stored at −80 °C.
2.2. Extract preparation and in vitro gastrointestinal digestions
Aqueous extracts (1
:
15, w
:
v) from freeze-dried RRS and RCS powders were elaborated by maceration for 4 hours in darkness and under continuous agitation. The samples were centrifuged at 13500g for 15 minutes. Supernatants were collected and percolated through a qualitative filter. For the in vitro gastrointestinal digestion, the INFOGEST standardized protocol was used.25 1 mL of the extract was mixed with 15 mL of a porcine pepsin solution (2000 units per mL in 100 mM HCl, Sigma-Aldrich, MO, USA) at pH 2 and 37 °C for 3 h. After the gastric digestion phase, 7.5 mL of 0.2 M sodium hydroxide (NaOH) was added to increase the pH to 7. For the intestinal digestion phase, another 7.5 mL of a pancreatin solution in phosphate buffer (2000 unit per mL, pH = 8) was added. For the intestinal phase, samples were incubated for 12 hours at 37 °C. In order to prevent the contamination of the samples by microorganisms, 75 μL of 0.5 mM merthiolate solution was included in the digestions. After the whole process, samples were kept overnight at 4 °C, then filtered through a 0.22 μm Ø PVDF membrane and freeze-dried for further experiments (Fig. 1). The digestates were labeled as follows: RRS (red radish sprout extract digestates); and (ii) RCS (red cabbage sprout extract digestates).
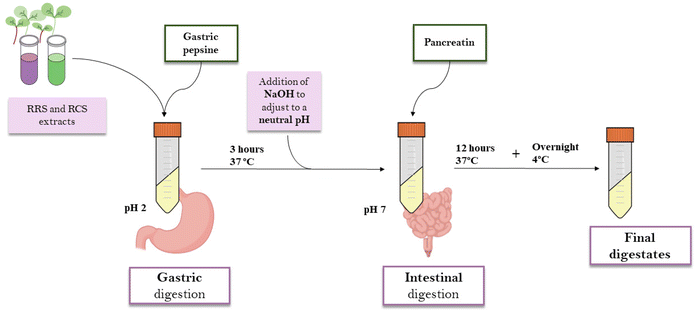 |
| Fig. 1 Schematic presentation of the standardized protocol followed for in vitro digestions. This protocol can be divided into a first phase of “gastric digestion”, developed at pH = 2 for 3 hours, and followed by a phase of “intestinal digestion” at pH = 7 for 12 hours. The whole protocol was performed at 37 °C. Once digested, the samples were incubated overnight at 4 °C and then kept at −80 °C until analysis. | |
2.3. Determination of ITCs in digestates
The presence of ITCs in both types of digestate from RRS and RCS extracts was analyzed and quantified using a UHPLC coupled with a 6460 triple quadrupole-MS/MS (Agilent Technologies, Waldbronn, Germany) and a Zorbax Eclipse Plus C18 column (2.1 × 50 mm, 1.8 μm).26 SFN, I3C, and DIM from Santa Cruz Biotech (California, USA) were used as standards. In order to quantify and detect the presence of flavonols and anthocyanins in the digestates, additional analysis with HPLC-DAD was performed and sinapinic acid (Sigma) and cyanidin-3-glucoside (Sigma) were used as external standards.26 Retention times and UV-Vis spectral characteristics, as well as the fragmentation patterns of the compounds of interest, were used in the determinations.
2.4. Cell culture
Digestates from RRS and RCS aqueous extracts were incorporated in the in vitro model of macrophage-like cells obtained by the differentiation of the human acute myeloid leukemia cell line HL-60 (ATCC® CCL-240™) as previously described.27 Briefly, the cells were kept undifferentiated and growing at an exponential ratio in complete culture media (CCM), RPMI-1640 (Biowest, Nuaillé, France) supplemented with 10% fetal bovine serum (GIBCO Invitrogen, Paisley, UK) and 1% penicillin/streptomycin (GIBCO) prior to their differentiation. Growing conditions were set at 37 °C and under a 5% CO2 atmosphere in the incubator. Cells were allowed to differentiate for conduction of the in vitro assays, always between passages number 5 and 20.
2.5. Cell viability assays
The cell viability of differentiated HL-60 macrophage-like cells treated with the digestates was measured using the MTT assay.27 Briefly, after cell differentiation with 10 ng mL−1 phorbol myristate acetate (PMA; Sigma Chemical Co., St Louis, MO, USA) in 96-well plates, the cells were pre-incubated with 10, 50, and 100 μg mL−1 of either RRS or RCS digestates, and after 30 minutes the cells were treated with LPS (0.1 μg mL−1) and incubated for 24 hours. MTT (Alfa Aesar, Thermo Fisher, Karlsruhe, Germany) was then added to the final concentration of 483 μM and incubated for 1 hour at 37 °C and under a 5% CO2 atmosphere. Then, an acidified isopropanol solubilization solution of 0.04 M hydrochloric acid and 0.1% NP-40 detergent was added to each well to lysate the cells and dissolve the purple formazan crystal formed inside the cells, obtaining a purple-colored solution. The absorbance was measured at 550 nm in a plate-reading spectrophotometer (Spectrostar Nano; BMG Labtech, Ortenberg, Germany). The cell viability was determined by comparison with control conditions, setting the latter as 100% viability, and thus 0% cytotoxicity. Control cells were maintained under basal conditions treated only with CCM. Three replicates were performed for each experiment.
2.6.
In vitro anti-inflammatory assays
The anti-inflammatory potential of the RRS and RCS digestates was examined by determining their ability to modulate the in vitro secretion of cytokines under a low-intensity inflammatory state induced by lipopolysaccharide (LPS).28 To achieve this, HL-60 cells (0.2*106 cells per well) were stimulated with 10 ng mL−1 phorbol 12-myristate 13-acetate (PMA) in CCM for 24 hours, in order to differentiate them into human macrophage-like cells.27 Afterward, the cells were rested for 24 hours in CCM without PMA. Once the differentiation was complete, the digestates of RRS and RCS aqueous extracts were pre-incubated with different concentrations (10, 50, and 100 μg mL−1) at 37 °C for 30 minutes. LPS (from Escherichia coli 0111.B4, Sigma Chemical Co.) was then added to a final concentration of 0.1 μg mL−1 to induce a low-grade pro-inflammatory state similar to those found in chronic inflammatory diseases. Finally, the plates were incubated at 37 °C under a 5% CO2 atmosphere for 24 hours. Supernatants were collected and stored at −20 °C for further analysis. The cells were washed with PBS and then stored at −80 °C until RNA extraction.
2.7. Enzyme-linked immunosorbent assays
Stored supernatants from in vitro assays performed on human macrophage-like HL-60 cells were used to determine the levels of the pro-inflammatory cytokines TNF-α, IL-6, and IL-1β using enzyme-linked immunosorbent assay (ELISA) kits (Invitrogen, Thermo Fisher Scientific, Waltham, MA, USA). The absorbance in each well was determined using a microplate reader (Spectrostar Nano; BMG Labtech, Ortenberg, Germany) at 450 nm and corrected at 570 nm. Each cytokine concentration was calculated using the corresponding standard curve. The modulation of cytokines was attained by calculating the level of release inhibition when compared to control conditions. In order to compare the effect of the RRS and RCS digestates from the different experiments (n ≥ 2), concentrations of the cytokines obtained from enzyme-linked immunosorbent assays (ELISA) (in the ranges 30–350 pg mL−1 for TNF-α, 250–800 pg mL−1 for IL-6, and 30–120 pg mL−1 for IL-1β) were normalized for each individual assay by setting the negative control of the corresponding experiment as the reference (valued as 1 for control conditions). The effect of the digestates on cytokine levels has been presented as the mean ± SEM of normalized results.
2.8. Gene expression determination by RT-qPCR
The entire RNA was extracted from macrophage-like differentiated HL-60 cells using the GenElute Mammalian Total RNA Miniprep kit (Sigma) following the manufacturer's instructions. RNA was eluted in RNAse-free water and checked for concentration and purity using the Nanodrop spectrophotometer system (ND-100 3.3. Nanodrop Technologies, USA). Only samples with a ratio of Abs260/Abs280 between 1.8 and 2.1 were used for gene expression assays. The generation of cDNA was executed using a high-capacity RNA-to-cDNA kit (Thermo Fisher Scientific, USA), following the manufacturer's instructions. PCR amplification was carried out with a PowerUp SYBR Green PCR Master Mix (Applied Biosystems, USA) using a Fast 7500 real-time instrument (Applied Biosystems, USA). Glyceraldehyde 3-phosphate dehydrogenase (GAPDH) was used as the reference gene. Primer sequences are presented in the ESI Table S1.† The relative expression was calculated using the 2−ΔΔCt method. All experiments were performed in triplicate and as three independent experiments.
2.9. Statistical analysis
Statistical analysis was performed using t-Student, ANOVA, and Tukey's HSD tests. Values of p < 0.05 were considered to be statistically significant. The GraphPad Prism 9 software was used for performing the calculations.
3. Results
3.1. Quantitative composition of secondary metabolites in the digestates
The results of the analysis and quantification of secondary metabolites present in RRS and RCS upon digestion of the aqueous extracts are shown in Table 1. According to ITCs, the SFN concentrations were higher in the digestates of RRS when compared to the digestates of RCS (p = 0.002). In addition, sulforaphene (SFE) was only detected in the digestates of RRS. Regarding I3C, the digestates of RCS contained around 3-fold higher I3C amounts compared to the digestates of RRS (p = 0.015). No traces of DIM, the naturally formed dimer of I3C, were found in RCS, although it was quantified in RRS. The ITC iberin was detected in digestates of both RRS and RCS in similar contents (p = 0.075). Accounting for the total amount of ITCs, the digestates of RCS were significantly higher than the digestates of RRS (p < 0.05).
Table 1 Composition of GSLs hydrolysis products, ITCs and phenolic compounds (sinapic acid derivatives and anthocyanins) analysed in the digestates obtained from aqueous extracts of RRS and RCS (mM)
(mM) |
|
RRS extract digestate |
RCS extract digestate |
Results are represented as the mean ± SD (n = 3). Different letters indicate statistically significant differences between extract digestates in Tukey's HSD test (p < 0.05). RCS: red cabbage sprouts, RRS: red radish sprouts, SFN: sulforaphane, SFE: sulforaphene, I3C: indole-3-carbinol, and DIM: 3,3-diindolylmethane. |
Isothiocyanates |
SFN |
6.60 ± 0.40a |
1.92 ± 0.18b |
SFE |
1.68 ± 0.06 |
— |
I3C |
30.00 ± 2.50a |
74.00 ± 7.06b |
DIM |
20.00 ± 2.12 |
— |
Iberin |
12.94 ± 0.56a |
10.75 ± 1.07a |
Total
|
71.19 ± 3.70a
|
86.67 ± 8.25b
|
Phenolic compounds |
Sinapic acid derivatives |
11.17 ± 0.27a |
12.56 ± 0.38a |
Anthocyanins (cyanidins) |
4.10 ± 0.04 |
— |
The composition of phenolic acids and anthocyanins in both digestates was also analyzed (Table 1). Among phenolic acids, the characteristic sinapic acid derivatives of these varieties were detected and quantified by HPLC-DAD in similar contents for both types of digestate (p > 0.05). However, only the digestates of RRS aqueous extracts presented a quantifiable amount of cyanidins.
3.2. The effect of digestates on human macrophage-like HL-60 cell viability
The percentage of cell viability of human macrophage-like HL-60 cells is presented in Fig. 2. The viability of the cells stimulated with LPS did not show any statistically significant difference when compared to those under basal conditions without LPS (control). Viability in terms of the metabolism ratio of the cells was also assessed in the presence of the two tested digestates with different concentrations of 10, 50, and 100 μg mL−1 under low-degree inflammation induced by LPS treatment. The RRS extract digestates induced a slight but significant elevation of the cellular metabolism with only a mild concentration of 50 μg mL−1 in the presence of LPS, showing an increase of 23% when compared to the control cells (p = 0.02), while no differences were found with cells treated with 10 and 100 μg mL−1 doses of digestates of RRS and LPS. Regarding RCS extract digestates, increases of 25% and 30% in cell viability were also observed in the cells treated with both 10 and 50 μg mL−1 respectively in the presence of LPS when compared to control conditions. No change in the cell viability was observed when they were treated with 100 μg mL−1 concentrations of RCS extract digestates and LPS.
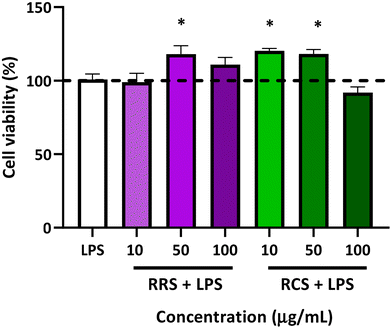 |
| Fig. 2 The effect on cell viability (%) of the macrophage-like HL-60 differentiated cells of red radish sprout (RRS) and red cabbage sprout (RCS) extract digestates (10, 50, and 100 μg mL−1) under a low degree of inflammatory conditions (0.1 μg mL−1 LPS). P-Values were calculated using the Student t-test. Values are expressed as percentages referred to a control basal condition without LPS (dash line). Results are presented as the mean ± SEM from 3 different MTT assays, with three replicates in each assay. *p < 0.05 between treatments and LPS-stimulated control. LPS, Lipopolysaccharide. | |
3.3. The in vitro effect of digestates on pro-inflammatory cytokines production by human macrophage-like HL-60 cells
The results obtained from analyzing the pro-inflammatory cytokine profile (TNF-α, IL-6, and IL-1β levels) released from human macrophage-like HL-60 cells treated with RRS and RCS extract digestates under low degrees of inflammatory conditions are presented in Fig. 3. For TNF-α levels (Fig. 3A), when LPS-stimulated cells were treated with 50 and 100 μg mL−1 doses of digestates obtained from RRS extracts, a reduction trend of 50% and 70% was observed respectively when compared with LPS-stimulated cells, while no differences were found for the TNF-α production when the lowest concentration of 10 μg mL−1 of the RRS extract digestates was added. Regarding the treatment with RCS extract digestates, a non-significant decrease of 50% in the levels of this pro-inflammatory cytokine was also observed with the highest concentration of 50 μg mL−1 when compared to the LPS-stimulated cells. Surprisingly, a further increase in TNF-α levels was observed after treatment with lower doses of 10 and 50 μg mL−1 of RCS extract digestates and LPS; these increments were significantly higher compared to those under basal conditions (p < 0.01 and p < 0.05 respectively for 10 and 50 μg mL−1 of RCS extract digestates).
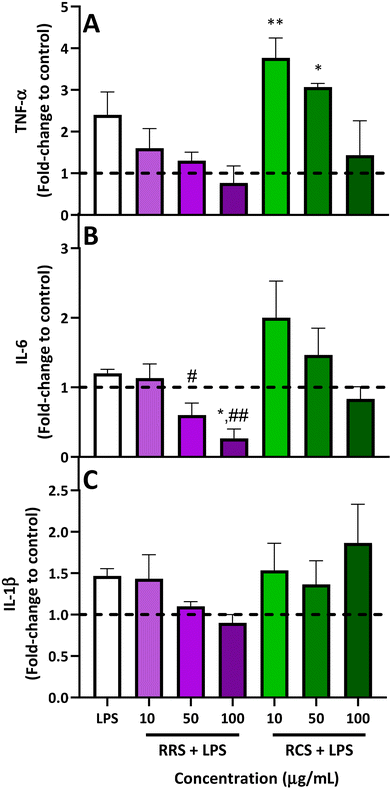 |
| Fig. 3 ELISA measured relative levels of TNF-α (A), IL-6 (B), and IL-1β (C) released from macrophage-like HL-60 differentiated cells under LPS-stimulated conditions (0.1 μg mL−1 LPS) following treatment with RRS and RCS extract digestates at different concentrations (10, 50 and 100 μg mL−1). P-Values were calculated using the ANOVA test with a post hoc Tukey's HSD test. Values are expressed as a fold-change to control basal conditions without LPS (dash line). Results are represented as the mean ± SEM from three different ELISA assays with three replicates performed in each assay. *p < 0.05, **p < 0.0 between treatments and basal conditions (without LPS). #p < 0.05, ##p < 0.0 between treatments and the LPS-stimulated control. TNF-α, tumor necrosis factor alpha; IL-6, interleukin 6; IL-1 β, interleukin 1 beta; LPS, lipopolysaccharide. | |
Regarding IL-6 (Fig. 3B), for RRS extract digestates, the highest reduction in this pro-inflammatory cytokine (80%) was achieved with 100 μg mL−1 compared to LPS-stimulated levels, reducing this cytokine level even further than those under basal conditions (p < 0.01 when compared with LPS-stimulated cells and p < 0.05 when compared to those under basal conditions), while the 50 μg mL−1 concentration produced a decrease of 46% compared with LPS-stimulated cells (p > 0.05). In the case of RCS extract digestates, a similar performance to that observed for TNF-α was also observed for IL-6. When LPS-stimulated macrophage-like cells were exposed to the highest concentration of 100 μg mL−1 of RCS extract digestates, a non-significant 30% decrease in IL-6 levels was observed, whilst an increment trend of 70% was found with the 10 μg mL−1 concentration. In the case of IL-1β (Fig. 3C), RRS extract digestates reported 26% and 35% decreasing tendencies respectively for concentrations of 50 and 100 μg mL−1, while no difference whatsoever was observed for the lowest concentration of 10 μg mL−1. For LPS-stimulated cells treated with RCS extract digestates, no significant differences were observed between the cells treated with only LPS and those treated with any of the three tested concentrations.
3.4. Effect of digestates on the gene expression of pro-inflammatory cytokines
The gene expression of TNF, IL6, and IL1B was analyzed in the presence of both types of digestate under LPS-stimulated and non-stimulated human macrophage-like HL-60 cells (Fig. 4; ESI Fig. S1†). Assays were performed at the concentration of 100 μg mL−1 of both tested digestates since this dose displayed the highest inhibitory effect on in vitro pro-inflammatory cytokines release without any cytotoxic effects. For TNF, no significant differences were found between the LPS-stimulated control cells and the ones treated with the RRS and RCS extract digestates. However, under control conditions without LPS (a non-inflammatory scenario) the treatment with a 100 μg mL−1 concentration of the RCS extract digestate showed a 2-fold increase in the TNF relative gene expression when compared to non-treated cells (p < 0.05). Regarding the IL6 expression, HL-60 macrophage-like cells stimulated with LPS showed a 10-times decrease in the relative gene expression after treatment with 100 μg mL−1 digestates of the RRS extract (p < 0.05). Cells maintained under the same pro-inflammatory conditions treated with the same concentration of RCS extract digestates (100 μg mL−1) showed no statistically significant differences in the IL6 gene expression levels when compared to LPS-treated cells (p < 0.05). In the case of unstimulated cells cultured under non-inflammatory conditions, no statistically significant differences in the IL6 gene relative expression were found between non-treated cells and those treated with any of the digestates. When the IL1B gene expression was analyzed in LPS-stimulated cells, a decrease of approximately 50% was observed after treatment with 100 μg mL−1 digestates of RRS. No significant differences were found between RCS extract digestates and those with LPS treatment alone. Nonetheless, under unstimulated conditions, a 2-times increase in IL1B expression levels was observed after treatment with 100 μg mL−1 digestates of the RCS extract (p < 0.05).
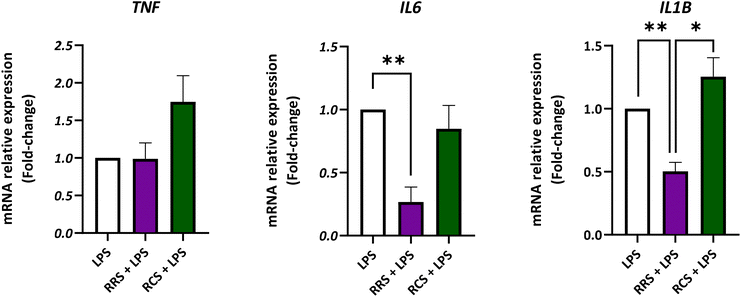 |
| Fig. 4 mRNA relative expression levels of genes involved in the immune response of human macrophage-like HL-60 differentiated cells exposed to red radish sprout (RRS) or red cabbage sprouts (RCS) extract digestate (100 μg mL−1) under low-degree inflammatory conditions (0.1 μg mL−1 LPS). P-Values were calculated using the ANOVA test with a post hoc Tukey's HSD test. Values are expressed as fold-change to the LPS-stimulated control. Results are represented as the mean ± SEM from three different assays with three replicates performed in each assay. *p < 0.05, **p < 0.01, ***p < 0.001. TNF, tumor necrosis factor; IL6, interleukin 6; IL1B, interleukin 1 beta; LPS, lipopolysaccharide. | |
4. Discussion
In recent years, the need to find alternative agents and/or coadjuvants to treat chronic inflammatory diseases has been highlighted. Emerging evidence has proposed the addition of phytochemicals obtained from plant sources with anti-inflammatory properties in the dietary pattern.29 Among those phytochemicals, natural compounds present in plants from the Brassicaceae family stand out because of their reported interaction with key molecular anti-inflammatory pathways.30 However, usually, testing doses used to conduct the corresponding in vitro and in vivo studies are higher than those concentrations that are used under physiological conditions or else do not represent suitable therapeutic approaches.24 Thus, this work aims to assess the anti-inflammatory potential of bioactive compounds of cruciferous sprouts (red radish and red cabbage) in their bioaccessible form (obtained by the digestion of aqueous extracts), using this simulation of physiological conditions for which the contents of individual compounds were in the concentration range from 0 to 50 μM. This in vitro digestion process provides the active fraction of compounds that might reach the gut epithelium and from there reach the lamina propria in human beings after the ingestion of food. To test the anti-inflammatory potential of such digestates we used a well-established in vitro inflammation cellular model31 consisting of human macrophage-like HL60 cells stimulated with LPS. This model tries to mimic systemic chronic inflammatory conditions present in certain non-communicable diseases such as cardiovascular disease, cancer, and diabetes.
The composition of the digestates of RRS and RCS extracts presented differences with a 20% lower content of total ITCs in RRS than in RCS. However, there was more variability in the compounds present in RRS than in RCS extract digestates, including SFE and DIM, which were exclusively present in RRS. The SFE is a structural analog of SFN, which only differs in one double bond that provides higher stability to this ITC.32 Also, previous studies have reported a high interconversion of GSLs into ITCs in sprouts, making them a great source for obtaining extracts and further digestates.33 In addition, although both digestates presented similar contents of sinapic acid derivatives, there was also a clear difference between the RCS and RRS, as the presence of cyanidins was only detected in RRS. The highly-acylated and glycosylated cyanidin derivatives of RRS may also interact with other bioactives in the extracts to exert their health-promoting activity in additive or synergistic combination, considering the demonstrated antioxidant and anti-inflammatory activity of the individual compounds, due to the coordination between the immune system and metabolism; developing novel dietary strategies to attenuate inflammation and oxidative stress seems promising and the combination of different metabolites present in food matrices (e.g. sprout extracts) could exert beneficial effects on metabolic markers and the reduction of inflammatory (cytokines) markers in cells and animals as found individually with anthocyanins23,34 and isothiocyanates.21,23,35
As the bioactivity of these digestates was determined in vitro, we found that they do not show any level of cytotoxicity with the assessed concentrations. These results were similar to those previously reported by us, in which among a series of diverse bioactive derivatives from Brassica plants, only SFN at the highest tested concentration (50 μM) showed a decrease in the cell viability of this in vitro model of HL-60 macrophage-like cells.31 In this regard, it is worth noting that the actual concentration of SFN present in the higher tested dose (100 μg mL−1) was only 3.72 μM for RRS and 1.08 μM for RCS, concentrations that were shown to be non-cytotoxic in this cellular model. Furthermore, low and medium digestate concentrations even produced a slight increase in the cell viability, in a similar way to that reported in HepG2 cells treated with Bimi® digestates.36 This phenomenon could be due to the presence of sugars and fibers that would favor an increase in the cell metabolism. Since in the extraction by aqueous maceration only hydrophilic molecules are extracted, no specific selection for ITCs has been made, thus, these kinds of molecules could also be present in the sprout extracts obtained before the in vitro digestion process.37
The in vitro anti-inflammatory potential of the digestates was tested by ELISA assay determination of the production of three pro-inflammatory cytokines TNF-α, IL-6, and IL-1β. It has been reported that TNF-α molecules exert a pleiotropic effect on diverse cell types, mainly by the activation of caspases, and the induction of intracellular signaling nuclear factor kappa B (NF-κB) and mitogen-activated protein kinases (MAPKs).38 IL-6 was originally defined as a differentiation factor for B cells. Its synthesis is regulated by LPS and TNF-α and shows both pro- and anti-inflammatory effects.39 IL-1β is also a pro-inflammatory interleukin that is secreted by macrophages in the classical activation pathway.40 Our results showed that the treatment of the cells with a low dose of RCS extract digestate (10 μg mL−1) unexpectedly tended to increase the TNF-α and IL-6 levels. For this digestate concentration, it might be argued that the ratio between the content of sugars and bioactive compounds could be high enough to activate the cell metabolism by the former (as observed by an increase in the cell metabolism), which would in turn induce the basal macrophage-like cells activity, augmenting thus the expression and release of cytokines in the LPS-dependent low-degree inflammatory scenario established. Furthermore, it has been reported that IL-6 is also a factor involved in glucose homeostasis, but whether its role is beneficial or detrimental is still a controversial topic.41 Nevertheless, a higher concentration of the 100 μg mL−1 RCS extract showed a trend of decreasing both the TNF-α and IL-6 production under LPS-stimulated conditions, and this inhibitory effect was mainly observed at the final protein expression. This activity at higher rates might be related to the inhibitory ability of iberin upon TLRs dimerization, impairing the NF-κB signaling patway.42 Furthermore, in LPS-induced RAW264.7 cells, I3C was shown to attenuate the production of inflammatory mediators, such as IL-6 and IL-1β.43 Since similar results were obtained with RCS extract digestates, its main bioactivity might occur through the modulation of these two molecules. However, herein IL-1β protein levels were unaffected by these treatments, which could be due to the differences between the two cellular models used, since HL-60 is a human cell line while RAW264.7 are mouse cells.
On the other hand, RRS exhibited a significant dose–response inhibition of the levels of production of IL-6 and a tendency to exhibit the same trend with the other two cytokines assessed herein. Furthermore, at the concentration of 100 μg mL−1 both the IL-6 protein production and its gene expression levels decreased under unstimulated conditions. This difference in the better performance of RRS compared to RCS could be exerted by the higher concentration of SFN, and the exclusive presence of SFE, DIM, and anthocyanins in RRS. The ability of SFN to negatively regulate the TLR4-CD14 cell-mediated activation has been reported.44,45 In addition, its ability to block NF-κB downstream signaling could prevent the transcription of pro-inflammatory genes.46 Although the available literature about SFE interaction with anti-inflammatory pathways is not as large as that with SFN, this structural analog could also interact via the NF-κB pathway, which would result in a decrease in pro-inflammatory cytokine levels.47 DIM is the condensation product of the dimerization of I3C, and also a hydrolysis derivate from indolic GSL glucobrassicin. In our previous work, treatment with DIM in the same LPS-stimulated HL-60 macrophage-like cell culture model reported a high anti-inflammatory activity against IL-6 production.31 This could explain the effect of RRS in the IL6 relative expression in LPS-stimulated cells. Moreover, DIM is not only able to down-regulate the NF-κB pathway but it has also been reported to be an AhR agonist, increasing the IL-1β levels and triggering a detoxification cascade.9 Concerning sinapic acid and its derivatives, their effect on the modulation of the NLRP3 inflammasome has recently been discovered, reducing the IL-1β production in bone marrow-derived macrophages.48 Finally, anthocyanins have also been reported to suppress the NF-κB and MAPK signaling pathways.49 In addition, when the effect of anthocyanin cyanidin-3-glucoside upon the HL-60 macrophage-like cell model stimulated with LPS was previously assessed, an anti-inflammatory response (to a greater or lesser extent) on TNF-α, IL-6, and IL-1β was also found.31 For all that, the main differences between RCS and RRS extract digestates might rely on their richness in bioactive molecules, showing a highly synergic anti-inflammatory combination for the RRS treatment.
5. Conclusion
In this work, we were able to establish the anti-inflammatory potential of two different digestates obtained from the aqueous extracts of certain Brassicaceae sprouts. In this way, human macrophage-like HL60 cells maintained in an LPS-stimulated low-grade inflammatory scenario showed a reduction in pro-inflammatory cytokine production with a dose–response trend when treated with RRS extract digestates. Furthermore, this RRS treatment was able to decrease the IL6 relative gene expression even under non-inflammatory conditions. Therefore, this work highlights the relevance of the precise composition of plant-derived foods, as it is the key factor that can determine the anti-inflammatory potential of complex mixtures of vegetal origin and sheds light on the search for nutritional interventions or coadjuvants that might help to improve relevant non-communicable diseases related to low-grade and chronic systemic inflammation.
Author contributions
P. G.-I. performed experiments, analyzed the data, and wrote the manuscript. M. A. N.-S. performed experiments, analyzed the data and wrote the manuscript. A. O.-B. performed experiments. M. A. M.-S. performed experiments and wrote the manuscript. B. R.-M. conceived and designed the experimental plan, performed experiments, analyzed the data, provided the necessary facilities, and wrote the manuscript. A. J. R.-A. conceived, designed and coordinated the experimental plan, performed experiments, analyzed the data, and wrote the manuscript. D. A.-M. conceived and designed the experimental plan, provided plant materials, provided necessary facilities, and wrote the manuscript. All authors read and approved the manuscript.
Conflicts of interest
There are no conflicts to declare.
Acknowledgements
This research was funded by the Fundación Séneca – Murcia Regional Agency for Science and Technology (Comunidad Autónoma de la Región de Murcia, CARM), through the grant number 20855/PI/18, D. A. M., and, by the Institute of Health “Carlos III” (ISCIII), and co-funded by the Fondo Europeo de Desarrollo Regional-FEDER (grant number PI20/00505, B. R. M.). P. G.-I., was funded by a grant from the Fundación Séneca-CARM, Spain (21273/FPI/19). M. A. M.-S, was supported by a PFIS contract from the ISCIII (FI21/00003, ISCIII, Spain; co-funded by the Fondo Europeo de Desarrollo Regional-FEDER). B. R.-M., was supported by the “Miguel Servet Type I” program (CP19/00098, ISCIII, Spain; co-funded by the Fondo Europeo de Desarrollo Regional-FEDER).
References
- L. Galland, Diet and inflammation, Nutr. Clin. Pract., 2010, 25, 634–640 CrossRef PubMed.
- D. Ramirez, A. Abellán-Victorio, V. Beretta, A. Camargo and D. A. Moreno, Functional ingredients from Brassicaceae species: Overview and perspectives, Int. J. Mol. Sci., 2020, 21, 1998 CrossRef PubMed.
- A. Abellán, R. Domínguez-Perles, D. A. Moreno and C. García-Viguera, Sorting out the value of cruciferous sprouts as sources of bioactive compounds for nutrition and health, Nutrients, 2019, 11, 429 CrossRef.
- D. B. Clarke, Glucosinolates, structures and analysis in food, Anal. Methods, 2010, 2, 310–325 RSC.
- M. A. Prieto, C. J. López and J. Simal-Gandara, Glucosinolates: Molecular structure, breakdown, genetic, bioavailability, properties and healthy and adverse effects, Adv. Food Nutr. Res., 2019, 90, 305–350 Search PubMed.
- R. Bayat Mokhtari, N. Baluch, T. S. Homayouni, E. Morgatskaya, S. Kumar, P. Kazemi and H. Yeger, The role of sulforaphane in cancer chemoprevention and health benefits: a mini-review, J. Cell Commun. Signaling, 2018, 12, 91–101 CrossRef.
- I. M. A. Ernst, K. Palani, T. Esatbeyoglu, K. Schwarz and G. Rimbach, Synthesis and Nrf2-inducing activity of the isothiocyanates iberverin, iberin and cheirolin, Pharmacol. Res., 2013, 70, 155–162 CrossRef CAS.
- K. R. Grose and L. F. Bjeldanes, Oligomerization of indole-3-carbinol in aqueous acid, Chem. Res. Toxicol., 1992, 5, 188–193 Search PubMed.
- T. T. Y. Wang, Q. Pham and Y. S. Kim, Elucidating the role of CD84 and AHR in modulation of LPS-induced cytokines production by cruciferous vegetable-derived compounds indole-3-carbinol and 3,3′-diindolylmethane, Int. J. Mol. Sci., 2018, 19, 1–16 Search PubMed.
- N. Zhang and P. Jing, Anthocyanins in Brassicaceae: Composition, stability, bioavailability and potential health benefits, Crit. Rev. Food Sci. Nutr., 2022, 62, 2205–2220 CrossRef CAS PubMed.
- N. Tena, J. Martín and A. G. Asuero, State of the art of anthocyanins: Antioxidant activity, sources, bioavailability and therapeutic effect in human health, Antioxidants, 2020, 9, 451 CrossRef CAS PubMed.
- J. M. Sankhari, M. C. Thounaojam, R. N. Jadeja, R. V. Devkar and A. V. Ramachandran, Anthocyanin-rich red cabbage (Brassica oleracea L.) extract attenuates cardiac and hepatic oxidative stress in rats fed an atherogenic diet, J. Sci. Food Agric., 2012, 98, 1688–1693 CrossRef PubMed.
- F. R. Greten and S. I. Grivennikov, Inflammation and cancer: Triggers, mechanisms, and consequences, Immunity, 2019, 51, 27–41 CrossRef.
- S. Galmés, M. Cifre, A. Palou, P. Oliver and F. Serra, A genetic score of predisposition to low-grade inflammation associated with obesity may contribute to discern population at risk for metabolic syndrome, Nutrients, 2019, 11, 298 CrossRef.
- R. Medzhitov, Origin and physiological roles of inflammation, Nature, 2008, 454, 428–435 CrossRef.
- D. R. Germolec, R. P. Frawley and E. Evans, Markers of inflammation, Methods Mol. Biol., 2010, 598, 53–73 CrossRef.
- R. Scrivo, M. Vasile, I. Bartosiewicz and G. Valesini, Inflammation as ‘common soil’ of the multifactorial diseases, Autoimmun. Rev., 2011, 10, 369–374 CrossRef PubMed.
- G. A. Green, Understanding NSAIDs: From aspirin to COX-2, Clin. Cornerstone, 2001, 3, 50–59 CrossRef CAS.
- K. M. Knights, A. A. Mangoni and J. O. Miners, Defining the COX inhibitor selectivity of NSAIDs: Implications for understanding toxicity, Expert Rev. Clin. Pharmacol., 2010, 3, 769–776 CrossRef CAS.
- M. Fine, Quantifying the impact of NSAID-associated adverse events., Am. J. Manage. Care, 2013, 19, 267–272 Search PubMed.
- A. J. Greaney, N. K. Maier, S. H. Leppla and M. Moayeri, Sulforaphane inhibits multiple inflammasomes through an Nrf2-independent mechanism, J. Leukocyte Biol., 2016, 99, 189–199 CrossRef CAS.
- M. T. López-Chillón, C. Carazo-Díaz, D. Prieto-Merino, P. Zafrilla, D. A. Moreno and D. Villaño, Effects of long-term consumption of broccoli sprouts on inflammatory markers in overweight subjects, Clin. Nutr., 2018, 38, 745–752 CrossRef PubMed.
- A. J. Ruiz-Alcaraz, M. A. Martínez-Sánchez, P. García-Peñarrubia, M. Martinez-Esparza, B. Ramos-Molina and D. A. Moreno, Analysis of the anti-inflammatory potential of Brassica bioactive compounds in a human macrophage-like cell model derived from HL-60 cells, Biomed. Pharmacother., 2022, 149, 112804 CrossRef.
- S. Quirante-Moya, P. García-Ibañez, F. Quirante-Moya, D. Villaño and D. A. Moreno, The role of Brassica bioactives on human health: Are we studying it the right way?, Molecules, 2020, 25, 1591 CrossRef.
- M. Minekus, M. Alminger, P. Alvito, S. Ballance, T. Bohn, C. Bourlieu, F. Carrière, R. Boutrou, M. Corredig, D. Dupont, C. Dufour, L. Egger, M. Golding, S. Karakaya, B. Kirkhus, S. Le Feunteun, U. Lesmes, A. MacIerzanka, A. MacKie, S. Marze, D. J. McClements, O. Ménard, I. Recio, C. N. Santos, R. P. Singh, G. E. Vegarud, M. S. J. Wickham, W. Weitschies and A. Brodkorb, A standardised static in vitro digestion method suitable for food-an international consensus, Food Funct., 2014, 5, 1113–1124 RSC.
- N. Baenas, C. Suárez-Martínez, C. García-Viguera and D. A. Moreno, Bioavailability and new biomarkers of cruciferous sprouts consumption, Food Res. Int., 2017, 100, 497–503 CrossRef.
- A. Guirado, J. I. López Sánchez, A. J. Ruiz-Alcaraz, D. Bautista and J. Gálvez, Synthesis and biological evaluation of 4-alkoxy-6,9-dichloro[1,2,4] triazolo[4,3-a]quinoxalines as inhibitors of TNF-α and IL-6, Eur. J. Med. Chem., 2012, 54, 87–94 CrossRef.
- U. Maitra, H. Deng, T. Glaros, B. Baker, D. G. S. Capelluto, Z. Li and L. Li, Molecular mechanisms responsible for the selective and low-grade induction of proinflammatory mediators in murine macrophages by lipopolysaccharide, J. Immunol., 2012, 189, 1014–1023 CrossRef CAS PubMed.
- A. Durazzo, L. D’Addezio, E. Camilli, R. Piccinelli, A. Turrini, L. Marletta, S. Marconi, M. Lucarini, S. Lisciani, P. Gabrielli, L. Gambelli, A. Aguzzi and S. Sette, From plant compounds to botanicals and back: A current snapshot, Molecules, 2018, 23, 1844 CrossRef.
- P. García-Ibañez, L. Yepes-Molina, A. J. Ruiz-Alcaraz, M. Martínez-Esparza, D. A. Moreno, M. Carvajal and P. García-Peñarrubia, Brassica bioactives could ameliorate the chronic inflammatory condition of endometriosis, Int. J. Mol. Sci., 2020, 21, 1–20 Search PubMed.
- A. J. Ruiz-Alcaraz, M. A. Martínez-Sánchez, P. García-Peñarrubia, M. Martinez-Esparza, B. Ramos-Molina and D. A. Moreno, Analysis of the anti-inflammatory potential of Brassica bioactive compounds in a human macrophage-like cell model derived from HL-60 cells, Biomed. Pharmacother., 2022, 149, 112804 CrossRef CAS.
- J. Zhang, X. Li, P. Ge, B. Zhang, L. Wen, C. Gu and X. Zhou, Sulforaphene: Formation, stability, separation, purification, determination and biological activities, Sep. Purif. Rev., 2022, 51(3), 330–339 CrossRef CAS.
- G. R. de Nicola, M. Bagatta, E. Pagnotta, D. Angelino, L. Gennari, P. Ninfali, P. Rollin and R. Iori, Comparison of bioactive phytochemical content and release of isothiocyanates in selected Brassica sprouts, Food Chem., 2013, 141, 297–303 CrossRef CAS.
- Y. M. Lee, Y. Yoon, H. Yoon, H. M. Park, S. Song and K. J. Yeum, Dietary anthocyanins against obesity and inflammation, Nutrients, 2017, 9, 1–15 Search PubMed.
- M. T. López-Chillón, C. Carazo-Díaz, D. Prieto-Merino, P. Zafrilla, D. A. Moreno and D. Villaño, Effects of long-term consumption of broccoli sprouts on inflammatory markers in overweight subjects, Clin. Nutr., 2018, 38, 745–752 CrossRef.
- P. Garcia-Ibañez, D. A. Moreno and M. Carvajal, Nanoencapsulation of Bimi® extracts increases its bioaccessibility after in vitro digestion and evaluation of its activity in hepatocyte metabolism, Food Chem., 2022, 385, 132680 CrossRef.
- S. C. Chuo, H. M. Nasir, S. H. Mohd-Setapar, S. F. Mohamed, A. Ahmad, W. A. Wani, M. Muddassir and A. Alarifi, A glimpse intoh the extraction methods of active compounds from plants, Crit. Rev. Anal. Chem., 2020, 52, 667–696 CrossRef.
- H. Mitoma, T. Horiuchi, H. Tsukamoto and N. Ueda, Molecular mechanisms of action of anti-TNF-α agents – Comparison among therapeutic TNF-α antagonists, Cytokine, 2018, 101, 56–63 CrossRef.
- M. Akbari and V. Hassan-Zadeh, IL-6 signalling pathways and the development of type 2 diabetes, Inflammopharmacology, 2018, 26, 685–698 CrossRef PubMed.
- A. Capobianco and P. Rovere-Querini, Endometriosis, a disease of the macrophage, Front. Immunol., 2013, 4, 9 Search PubMed.
- L. L. Lehrskov and R. H. Christensen, The role of interleukin-6 in glucose homeostasis and lipid metabolism, Semin. Immunopathol., 2019, 41, 491–499 CrossRef PubMed.
- T. Shibata, F. Nakashima, K. Honda, Y. J. Lu, T. Kondo, Y. Ushida, K. Aizawa, H. Suganuma, S. Oe, H. Tanaka, T. Takahashi and K. Uchida, Toll-like receptors as a target of food-derived anti-inflammatory compounds, J. Biol. Chem., 2014, 289, 32757–32772 CrossRef CAS PubMed.
- J. Jiang, T. B. Kang, D. W. Shim, N. H. Oh, T. J. Kim and K. H. Lee, Indole-3-carbinol inhibits LPS-induced inflammatory response by blocking TRIF-dependent signaling pathway in macrophages, Food Chem. Toxicol., 2013, 57, 256–261 CrossRef PubMed.
- H. S. Youn, Y. S. Kim, Z. Y. Park, S. Y. Kim, N. Y. Choi, S. M. Joung, J. A. Seo, K.-M. Lim, M.-K. Kwak, D. H. Hwang and J. Y. Lee, Sulforaphane suppresses oligomerization of TLR4 in a thiol-dependent manner, J. Immunol., 2010, 184, 305–319 CrossRef.
- J. E. Koo, Z. Y. Park, N. D. Kim and J. Y. Lee, Sulforaphane inhibits the engagement of LPS with TLR4/MD2 complex by preferential binding to Cys133 in MD2, Biochem. Biophys. Res. Commun., 2013, 434, 600–605 CrossRef PubMed.
- E. Heiss, C. Herhaus, K. Klimo, H. Bartsch and C. Gerhäuser, Nuclear factor kappa B is a molecular target for sulforaphane-mediated anti-inflammatory mechanisms, J. Biol. Chem., 2001, 276, 32008–32015 CrossRef PubMed.
- S. Han, Z. Wang, J. Liu and Q. Yuan, Identifying the p65-dependent effect of sulforaphene on esophageal squamous cell carcinoma progression via bioinformatics analysis, Int. J. Mol. Sci., 2020, 22, 60 CrossRef PubMed.
- E. H. Lee, J. H. Shin, S. S. Kim and S. R. Seo, Sinapic acid controls inflammation by suppressing NLPR3 inflammasome activation, Cells, 2021, 10, 2327 CrossRef PubMed.
- T. Qiu, Y. Sun, X. Wang, L. Zheng, H. Zhang, L. Jiang, X. Zhu and H. Xiong, Drum drying-and extrusion-black rice anthocyanins exert anti-inflammatory effects via suppression of the NF-κB /MAPKs signaling pathways in LPS-induced RAW 264.7 cells, Food Biosci., 2021, 41, 100841 CrossRef.
|
This journal is © The Royal Society of Chemistry 2023 |
Click here to see how this site uses Cookies. View our privacy policy here.