In situ detection of neodymium isotopes using tunable diode laser absorption spectroscopy for nuclear forensic analysis
Received
12th October 2022
, Accepted 11th November 2022
First published on 14th November 2022
Abstract
As evidence for tracing the history of nuclear materials, in situ detection of neodymium isotopes using tunable diode laser absorption spectroscopy (TDLAS) was investigated. A compact optical configuration was constructed by integrating laser optics and sample vaporization apparatus. The absorption spectra of all naturally occurring neodymium isotopes were measured from the interaction between collimated neodymium vapor and a tunable diode laser. Under optimized conditions for weakening the Doppler effect, seven neodymium isotopes could be clearly identified in the absorption spectra notwithstanding their small isotopic shifts (about 0.3 pm). By comparing these distinctive absorption peaks, the isotopic abundance could be accurately estimated with about 0.24% absolute bias. Furthermore, the detection limit of neodymium in various oxide matrices was evaluated to verify the sensitivity of the system. Although the direct absorption mode exhibited insufficient absorbance, the signal amplification methods including chemical reduction and wavelength modulation successfully improved the detection limit down to the ppm level. Consequently, the present research reported for the first time the absorption spectra of seven neodymium isotopes and also demonstrated the feasibility of a TDLAS-based detection system for isotope analysis in nuclear forensics.
Introduction
Nuclear forensics is a comprehensive analysis of nuclear and radioactive materials for law enforcement investigations or the assessment of nuclear security vulnerabilities.1 Numerous analytical techniques have been utilized for nuclear forensics to prevent terrorism or trafficking associated with nuclear materials.2 In particular, isotopic analysis plays a significant role in determining the genesis and history of forensic targets.3 Neodymium isotopes, for example, could be conclusive evidence for the classification of uranium ore concentrates (UOCs).4 Natural neodymium is composed of seven isotopes, including five stable isotopes (142Nd, 143Nd, 145Nd, 146Nd, and 148Nd) and two long-lived radioisotopes (144Nd and 150Nd). Depending on the geological characteristics, the ratio of 143Nd to 144Nd exhibits specific values due to the decay of 147Sm (T1/2 = 1.06 × 1011y)3. The given isotope composition of neodymium, therefore, could be utilized to verify where the UOC stems from. In addition, the ratio between 145Nd and 146Nd could be used as an indicator for the burnup history of uranium fuel from pressurized water reactors.5 For these reasons, neodymium isotopes are considered one of the representative signature materials for nuclear forensics. Mass spectrometry techniques such as ICP-MS (inductively coupled plasma mass spectrometry) and TIMS (thermal ionization mass spectrometry) have been commonly utilized for isotope detection of neodymium.6,7 However, these methods entail complex and tedious sample preparation, including chemical separation and dilution to avoid isobaric interference. Since nuclear forensics requires immediate decision-making with comprehensive information on the target material, a fieldable analytical method for neodymium isotope detection should be developed.
Laser spectroscopy could be a promising technique for nuclear forensics due to its advantages including fast detection, portable apparatus, and simple sample preparation.8,9 However, it is not easy to distinguish the optical spectra of isotopes since the magnitude of the isotopic shift is too small to be practically resolved using a conventional spectrometer.10 To overcome this drawback, tunable diode laser absorption spectroscopy (TDLAS) could be employed. The high resolution adequate to resolve small isotopic shifts could be achieved by utilizing an external cavity diode laser (ECDL), which provides an extremely narrow linewidth (about 100 kHz). The absorption spectra induced by the interaction between the diode laser and the gas mixture could provide qualitative and quantitative information about the isotopes of interest. Additionally, the use of collimated atomic vapor (atomic beam) as an analyte further enhances the spectral resolution by weakening the Doppler effect.11
Up to now, several efforts have been made to develop isotope detection techniques based on TDLAS with collimated effusion sources.11–13 Lebedev et al.12 demonstrated the detection capability of a compact TDLAS system for erbium isotopes. Their subsequent studies showed that the TDLAS system is capable of not only distinguishing the uranium isotopes,13 but also identifying their unreported hyperfine structure.14 Those studies sufficiently verified the possibility of TDLAS for in-field isotope detection. However, TDLAS has not been applied to neodymium detection since the previous diode lasers were not appropriate to examine the atomic transition of neodymium in the visible range from 400 to 500 nm. Furthermore, the sensitivity has not been sufficiently considered despite its significance. Since the detection capability of an optical configuration could heavily depend on the sample and conditions,15 the limit of detection should be systematically evaluated to confirm the feasibility. In addition, the signal enhancement method might be required to satisfy the desired performance for nuclear forensic applications.
The present study focused on the development of a TDLAS system for the detection of neodymium isotopes in nuclear forensics. The compact optical configuration including a vaporization device was constructed and optimized to obtain the desired resolution and sensitivity. Absorption spectra were measured from the interaction between a finely tuned diode laser and collimated neodymium vapor. Under optimized conditions to mitigate the Doppler broadening effect, seven neodymium isotopes could be clearly distinguished in the observed spectra. The isotopic ratio of neodymium could be estimated by comparing the area of the absorption peak. In addition, signal enhancement methods including chemical reduction and wavelength modulation could successfully improve the detection sensitivity by a factor of about 100 in comparison to a direct absorption mode. The detection limit of neodymium was a few ppm in common ore matrices. In conclusion, the capability of the constructed TDLAS system was demonstrated in terms of resolution and sensitivity for the in-field detection of neodymium isotopes.
Experimental
Fig. 1 describes the schematic diagram of the experimental set-up. An external cavity diode laser (DL pro, 5 mW, Toptica Photonics) with a narrow linewidth (<100 kHz at 5 μs integration time) was employed to detect the atomic transition of neodymium centered at 492.45 nm. The laser wavelength was finely tuned by the piezoelectric current from a laser controller (DLC pro, Toptica Photonics), as shown in Fig. 2(a). This laser light was divided into two pathways by a beam splitter. One of them is forwarded to a wavelength meter (WS-7, HighFinesse GmbH) for monitoring the laser wavelength. The other was delivered to a photodiode (818-BB, Newport Corporation) after passing through the right side above the crucible. The diameter of the laser beam was adjusted to the size of the crucible exit using a planoconvex lens. The signal from the photodiode was monitored using an oscilloscope (TDS 380P, Tektronix). A lock-in amplifier (SRS 830, Stanford Research Systems) was utilized for laser wavelength modulation (please refer to the section on wavelength modulation).
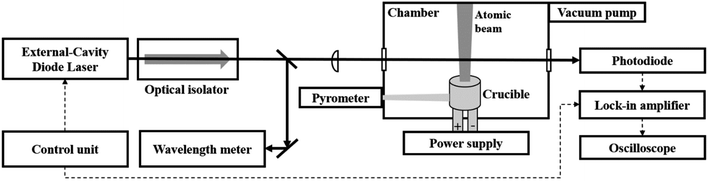 |
| Fig. 1 Schematic diagram of the experimental set-up. | |
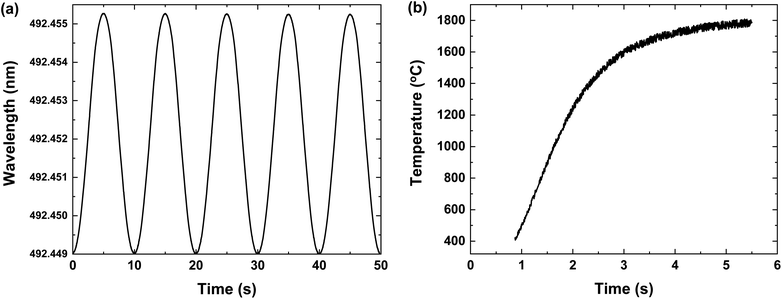 |
| Fig. 2 (a) Sinusoidally tuned wavelength of an external cavity diode laser and (b) crucible temperature as a function of time at an applied power of 150 W. | |
To portably measure the absorption spectrum from various analytes, the vaporization apparatus consisting of a crucible and chamber needs to be integrated into the system. At the same time, the system should be as compact and simple as possible. Therefore, a simple cylindrical crucible was fabricated by folding a piece of tantalum foil (0.025 mm thickness, Alfa Aesar), which has a superior heat resistance and ductility.12 The crucible was loaded in a handmade vacuum chamber (1 ∼ 2 mTorr) and fixed using a copper feed-through connected to a power supply (PSU 6, GW instruments). The present configuration requires only a small volume (1 m3) and weight (60 kg), which allows mobile use.
The neodymium metal (99.9% purity, Aldrich) and oxide (Nd2O3, 99.99% purity, Aldrich) powders with natural isotopic abundance were prepared as the target analyte. To prevent cross-contamination, the crucible was replaced with a new one for each different sample. After inserting about 50 mg of sample, the crucible was heated by applying electric power and the temperature was monitored using a remote pyrometer (Raynger®, Fluke Process Instruments), as shown in Fig. 2(b). When applying power, the temperature reached the set value within a few seconds. After this, the system could sustainably create the stable atomic beam for a few minutes, providing sufficient time for the vapor-laser interaction. Every measurement including the blank was repeated five times under the same conditions.
Results and discussion
Optimization of the detection system
Neodymium isotope analysis by laser spectroscopy has rarely been conducted due to the following reasons: (1) complex spectra of the seven isotopes, (2) a small isotopic shift, and (3) low optical sensitivity. As a result, most spectral features of neodymium isotopes are still unavailable. In this context, a comprehensive spectral analysis of the neodymium isotopes needs to be conducted. First of all, the atomic absorption spectra were measured from the neodymium transition (4f46s2–4f46s6p) at 492.45 nm. Within about five picometers of the spectral range, the absorption signal was identified with several peaks from neodymium isotopes, as shown in Fig. 3. It could be noticeable that the absorbance and linewidth heavily depend on the experimental conditions. Although the resolving power of the present diode laser is sufficient considering the reported isotope shift of neodymium,24 the neodymium isotopes cannot be distinguished in the black spectrum in Fig. 3. This result indicates the necessity of further optimization of the configuration to reduce the line-broadening effects.
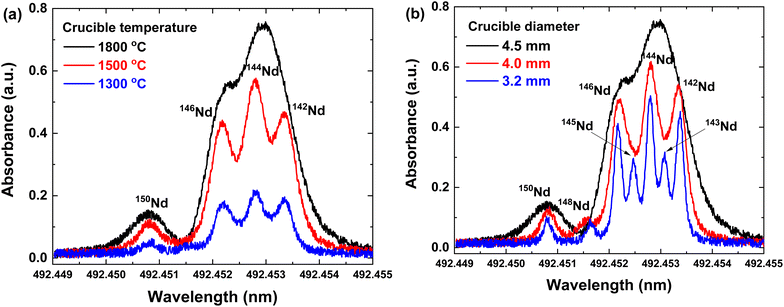 |
| Fig. 3 Absorption spectra of neodymium isotopes according to (a) the temperature of the crucible with a fixed diameter of 4.5 mm and (b) the diameter of the crucible at a fixed temperature of 1800 °C. | |
In the present work, most efforts of the optimization process were focused on finding the best crucible conditions, which determine the characteristics of atomic vapor19 and eventually govern the detection capability. Above all, the crucible temperature needs to be specifically adjusted to create a sufficiently dense and well-collimated atomic beam. In order to create the atomic beam of neodymium under the given ambient pressure (1 ∼ 2 mTorr), the crucible should be heated to higher than 1300 °C to reach the desired vapor pressure of the analyte (10 mTorr).16 Meanwhile, the Doppler line broadening leading to the overlap between absorption peaks could be enhanced under high-temperature conditions.17 With these two constraints in mind, the absorption spectra were measured with temperature being adjusted in the range from 1300 to 1800 °C, as shown in Fig. 3(a). As intuitively expected, the absorbance increased with temperature since the higher thermal energy produces denser atomic vapor. But at the same time, the line width of the absorption peak also broadened due to the Doppler broadening effect, and the peaks of neodymium isotopes were rarely resolved at temperatures higher than 1800 °C.
To overcome this drawback, the effect of the crucible geometry on the spectra was investigated, adjusting the diameter from 3.2 mm to 4.5 mm. As can be seen from Fig. 3(b), the line width of the isotope absorption peaks was remarkably reduced by narrowing the crucible. Strikingly, the crucible configuration with a diameter of 3.2 mm allowed the seven neodymium isotopes including minor isotopes (i.e.143Nd, 145Nd, and 148Nd) to be clearly distinguished in the absorption spectrum. This result could be explained by the fact that the atomic beam from the narrower crucible could be better collimated, thereby, weakening the Doppler broadening effect.17 The partial overlap between absorption peaks could be caused by the hyperfine structure of odd isotopes (143Nd and 145Nd). The isotopic shifts of neodymium isotopes with respect to the most abundant stable isotope, 142Nd, were calculated on the basis of each peak position (Table 1). The observed isotopic shift agreed well with the reported values in previous research studies using Fabry–Pérot spectrometry.24–26
Table 1 Observed isotopic shift in the neodymium absorption spectra and the results of the isotope quantification by comparing the area of Voigt profiles
|
Isotopic shift from the 142Nd peak (pm) |
Nominal abundance (%) |
Estimated abundance (%) |
Absolute bias (%) |
150Nd |
2.8 |
5.64 |
5.40 |
0.24 |
148Nd |
2.0 |
5.76 |
5.95 |
0.19 |
146Nd |
1.5 |
17.19 |
16.94 |
0.25 |
145Nd |
1.1 |
8.29 |
8.63 |
0.34 |
144Nd |
0.8 |
23.80 |
23.97 |
0.17 |
143Nd |
0.5 |
12.17 |
11.81 |
0.36 |
142Nd |
0.0 |
27.15 |
27.30 |
0.15 |
|
|
|
Average |
0.24 (±0.17) |
Multi-channel crucibles
In the previous section, even though sufficient resolution was achieved by reducing the crucible diameter, the absorbance also decreased at the same time. In other words, a narrowing of the crucible diameter can decrease the detection sensitivity. Further investigations, therefore, have been made to improve the resolution as well as the detection sensitivity. Various crucible designs have been developed to improve the density and directionality of atomic beams in previous studies.18–23 However, most of them require complex fabrication processes and cooling systems which can present barriers to maintaining the compactness of the system. In this context, a multichannel array that can be fabricated by inserting the internal capillary into the external crucible21 could be an attractive option for an alternative crucible design. In this configuration, the atomic vapor could be strongly collimated by multiple capillaries while maintaining the cross-sectional area of the crucible orifice.22 In the present work, a multichannel crucible consisting of five microcapillaries (ϕ = 1.5 mm) embedded into an external crucible (ϕ = 4.5 mm) was fabricated using pieces of tantalum foil.
The spatial distribution of the created atomic beam has to be evaluated to verify the integrity of the multichannel crucible. By moving focusing spots (0.1 mm in diameter) according to the direction perpendicular to the atomic beam, the spatially resolved absorbance was measured at 1 cm above the crucible exit, as presented in Fig. 4(a). The degree of beam collimation was greatly improved by adopting the multichannel array with divergence reduced from 7° to 2°. This enhancement in beam collimation could result in Doppler broadening suppression. The observed spectra using the multichannel crucible are shown in Fig. 4(b). The individual isotope could be clearly distinguished with the reduced full width at half maximum (FWHM) smaller than 0.2 pm. In addition, the magnitude of absorbance was slightly higher in comparison to the previous result from the single-channel crucible. Consequently, the present detection system could produce a well-collimated and sufficiently dense atomic beam to analyze the neodymium isotopes.
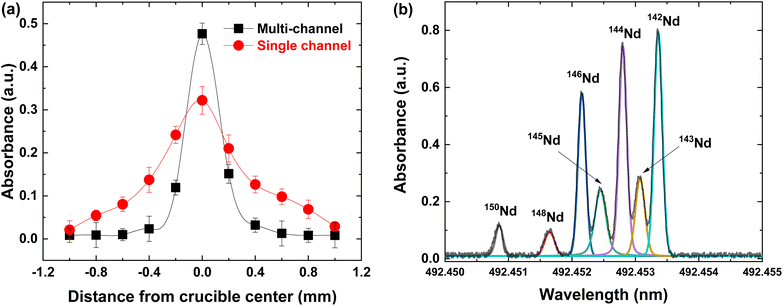 |
| Fig. 4 (a) Spatially resolved absorbance at 1 cm above the crucible using a single channel (red line) and multi-channel crucible (black line) and (b) absorption spectrum by using the multichannel crucible at a temperature of 1800 °C. (Each peak was fitted with the Voigt profile). | |
The quantity of the analyte could be proportional to the absorbance according to the Beer–Lambert law.27 In the same manner, the isotopic abundance of neodymium could be assessed by comparing the magnitude of the absorption signal. In order to unambiguously classify each isotope in the spectra, each absorption peak was fitted with the Voigt profile, shown as different coloured lines in Fig. 4(b). After the peak area of each profile was calculated as a standard for quantitative analysis, the isotopic abundance of neodymium was estimated by comparison, as listed in Table 1. The predicted values corresponded well to the nominal isotopic abundance with an average absolute bias of 0.24%. The result achieved so far demonstrates the capability of the present system for isotopic quantification through rapid and simple measurement.
Detection limit of neodymium in various matrices
The detection limit has to be evaluated systematically to check the feasibility of the constructed system, as natural neodymium in the ore commonly exists with complex compounds. The most common materials in the ore such as silica (SiO2), alumina (Al2O3), and hematite (Fe2O3) were prepared as matrices. Neodymium metal and oxide were mixed into these matrices in the range from 10 ppm up to 10%. After loading the samples into the multichannel crucible, the absorption spectra were measured at 1800 °C. The limit of detection (LOD) could be calculated using the 3-sigma formula, as given below.28 The symbol s indicates the slope of the linear calibration between absorbance and concentration. The noise (σ) was determined using the standard deviation of the background signal. The evaluated LOD is tabulated in Table 2.
Table 2 Limit of detection (LOD) of neodymium depending on matrices and methods using a multichannel crucible at 1800 °C
Unit: ppm |
Methoda |
SiO2 matrix (Cp: 26.5 J mol−1 K−1) |
Al2O3 matrix (Cp: 78.77 J mol−1 K−1) |
Fe2O3 matrix (Cp: 103.7 J mol−1 K−1) |
Direct absorption (DA), wavelength modulation (WM), and chemical reduction (CR).
|
Nd metal |
DA |
121 |
322 |
482 |
WM |
4 |
12 |
17 |
Nd oxide (Nd2O3) |
DA |
1584 |
2121 |
4105 |
CR |
373 |
521 |
742 |
CR + WM |
14 |
21 |
29 |
First, the salient point is the difference in LOD between metals and oxides. With direct absorption, the LOD for neodymium metal was a few hundred ppm, whereas the oxides have about a ten times higher detection limit. This difference could be caused by the fact that the oxide requires additional thermal energy for atomization. Namely, the atomic density of vapor from the oxide became much lower than that from the metal. This could be the drawback of the present system for in situ detection, considering that oxide is the common form of natural neodymium. Therefore, the method to solve this problem should be studied, which will be handled in the later section.
In terms of matrix dependency, the LOD increases in the order of silica, alumina, and hematite. This tendency seems to be governed by the specific heat capacity (Cp) of the matrix. The higher heat capacity means much more thermal energy is required to raise the temperature. Therefore, the high Cp matrix could extort the thermal energy from the target analyte, resulting in a reduction in the atomic density of the vapor. The detection limit is thus proportional to the Cp of the surrounding matrix. In the results of direct absorption, the lowest detection limit was 121 ppm for neodymium metal in the silica matrix. It should be noted that this level of sensitivity is not sufficient to be applied to the in-field detection of neodymium as the typical amount of neodymium is about 40 ppm in the natural ore.29 In this context, further investigations into signal enhancement should be conducted, which will be discussed in the following section.
Chemical reduction
As noted in the previous section, the detection limit was inferior for oxide samples due to the low density of the atomic beam. Chemical reduction could be the solution to this problem, as a reducing agent allows the target atom to be easily liberated from the oxides.30 The reaction expressed in eqn (2) could proceed in the forward direction when M2O3 is more thermodynamically favourable than Nd2O3 (i.e.
). | Nd2O3 + 2M (reducing agent) → 2Nd + M2O3 | (2) |
In the present work, erbium and lutetium were employed as candidates for reducing agents due to their negative oxidation potential and lower volatility (
,
, and
at 1800 °C).30,35,36 Before loading the sample into the crucible, 20 mg of Nd2O3 was evenly blended with erbium and lutetium metals. To examine the performance of the agents, the absorption signal was measured as a function of temperature (Fig. 5). The absorbance was increased severalfold by using reducing agents (red and blue lines) in comparison with direct absorption (black line). In particular, lutetium provided a significant enhancement of the signal, which was five times higher than that of direct absorption. This result could be related to the more negative oxidation potential of lutetium compared to erbium. Furthermore, the onset temperature for measuring the detectable signal decreased to 1300 °C using reducing agents, whereas the spectra without agents could not reveal a meaningful signal up to 1600 °C. Thus, the system can be operated at a lower temperature by using reducing agents, providing the advantages of power efficiency and mitigating the Doppler effect.
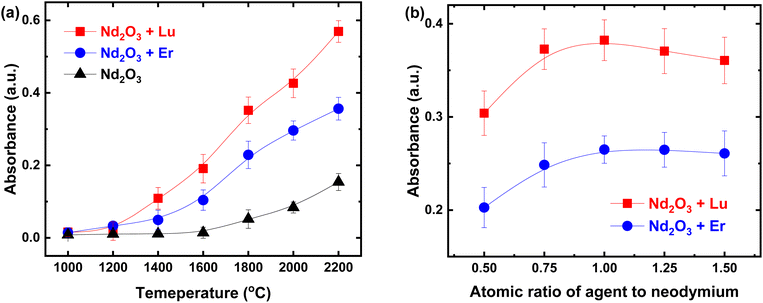 |
| Fig. 5 Absorbance of the 142Nd peak when using chemical reduction according to (a) the temperature and (b) the atomic ratio of the reducing agent to neodymium at 1800 °C. | |
To optimize the reduction conditions, the absorbance was investigated as a function of the amount of reducing agents, as shown in Fig. 5(b). The absorption signal increased continuously with the addition of the reducing agent until the number of its atoms became identical to that of neodymium. After this, an excess of agents gradually reduced the absorbance, since lutetium and erbium could act not merely as reducing agents but also as additional impurities. Taking these results into account, lutetium was added with the same number of atoms as neodymium to obtain the highest signal enhancement. Based on the enhanced absorption spectra using chemical reduction, the LOD was improved more than four times in comparison to direct absorption (refer to the CR row in Table 2).
Wavelength modulation
Although the sensitivity was improved by chemical reduction, the detection limit still could not meet the desired performance for neodymium detection in natural ores. Therefore, another improvement method needs to be applied. Wavelength modulation (WM) is one of the well-established methods to increase the sensitivity of absorption spectroscopy.31–33 The interaction between the modulated wavelength and absorption feature could produce harmonic components that can be isolated using a lock-in amplifier in a derivative form.34 The WM method could significantly improve the detection sensitivity by filtering the noise outside of the harmonics. In the present system (see Fig. 1), the laser wavelength was modulated in a fast-sinusoidal form using the laser controller synchronized with a lock-in amplifier. The second harmonic signal (2f) was utilized because of its largest SNR among the harmonic components. The modulation frequency and amplitude were optimized at 1 kHz and 450 mV to obtain the highest signal-to-noise (SNR).
Fig. 6(a) shows the 2f harmonic signal from the lock-in amplifier. The magnitude of the signal could be calculated from the difference between the maximum peak and the minimum point of the line wing. Under the optimized conditions, the SNR of the 2f harmonic signal is about 30 times higher than that of direct absorption. The linear calibration between the SNR and neodymium concentration is shown in Fig. 6(b). The second harmonic provides a detectable signal even for a trace amount of neodymium, while the direct absorption falls off rapidly to the noise level. By simultaneously adapting chemical reduction and wavelength modulation, the detection sensitivity could be increased by a factor of about 100. Furthermore, the detection limit was improved down to a few ppm (refer to Table 2). The final LOD became sufficient to be applied to nuclear forensics, considering that the average abundance of neodymium on the Earth's crust is about 40 ppm.29 Consequently, the present TDLAS-based system could provide sufficient sensitivity as well as resolution for in situ detection of neodymium isotopes.
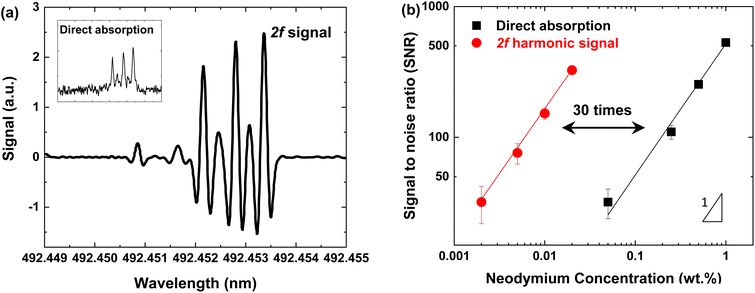 |
| Fig. 6 (a) Second harmonic signal from 5 wt% of neodymium in silica and (b) linear calibration between the SNR and neodymium concentration in the silica matrix with a slope of 1 on a logarithmic scale. | |
Conclusions
In the present study, a TDLAS system for the detection of neodymium isotopes was successfully constructed. The optical configuration with the atomic beam generation apparatus was optimized to obtain the desired resolution and sensitivity. A multichannel crucible was employed to weaken the Doppler broadening effect by improving the collimation of the atomic beam. In the observed spectra, the line width of the absorption peak could be reduced by 0.2 pm of FWHM, allowing seven isotopes of neodymium to be clearly distinguished. Using these distinctive spectra, the isotopic abundance of neodymium could be evaluated by comparing the peak area of each isotope. In addition, signal enhancement methods were adopted to improve the deficient sensitivity of direct absorption. The addition of lutetium as a reducing agent provides a five times higher signal by facilitating atomization of the neodymium oxide. Wavelength modulation could improve the SNR about 30 times by isolating the second harmonic signal. With these methods, the detection limit could be lowered to the ppm level. The present configuration requires only a small volume (1 m3) and weight (60 kg) to rapidly provide quantitative and qualitative information on neodymium isotopes. In addition, the preparation required is to detach only about 30–50 mg of the small piece to be loaded into the crucible. Consequently, the constructed system could compensate for the limitation of conventional mass spectrometry in fieldable applications and help to improve the celerity of decision-making in nuclear forensics.
Author contributions
Sung-Uk Choi: conceptualization, methodology, formal analysis, investigation, writing – original draft. Sol-Chan Han: investigation, validation, writing – review & editing. Jong-Il Yun: conceptualization, writing – review & editing, supervision.
Conflicts of interest
There are no conflicts to declare.
Acknowledgements
This study was supported by the National Research Foundation of Korea (NRF) grant funded by the Korean government (MSIP: Ministry of Science, ICT and Future Planning) (grant code: 2021M2C7A1A02076339).
References
- M. J. Kristo, A. M. Gaffney, N. Marks, K. Knight, W. S. Cassata and I. D. Hutcheon, Nuclear forensic science: analysis of nuclear material out of regulatory control, Annu. Rev. Earth Planet. Sci., 2016, 44, 555–579 CrossRef CAS , LLNL-JRNL-683854.
- M. J. Kristo and S. J. Tumey, The state of nuclear forensics, Nucl. Instrum. Methods Phys. Res., Sect. B, 2013, 294, 656–661 CrossRef CAS.
- J. Krajkó, Z. Varga, E. Yalcintas, M. Wallenius and K. Mayer, Application of neodymium isotope ratio measurements for the origin assessment of uranium ore concentrates, Talanta, 2014, 129, 499–504 CrossRef PubMed.
- S. O. O. John, I. T. Usman, T. C. Akpa and U. Ibrahim, Rare earth elements in uranium ore for nuclear forensic application, IOP Conf. Ser.: Earth Environ. Sci., 2021, 655(1), 012075 CrossRef.
- J. S. Kim, Y. S. Jeon, S. Dal Park, Y. K. Ha and K. Song, Analysis of high burnup pressurized water reactor fuel using uranium, plutonium, neodymium, and cesium isotope correlations with burnup, Nucl. Eng. Technol., 2015, 47(7), 924–933 CrossRef.
- K. F. Huang, J. Blusztajn, D. W. Oppo, W. B. Curry and B. Peucker-Ehrenbrink, High-precision and accurate determinations of neodymium isotopic compositions at nanogram levels in natural materials by MC-ICP-MS, J. Anal. At. Spectrom., 2012, 27(9), 1560–1567 RSC.
- J. Harvey and E. F. Baxter, An improved method for TIMS high precision neodymium isotope analysis of very small aliquots (1–10 ng), Chem. Geol., 2009, 258(3–4), 251–257 CrossRef CAS.
- S.-U. Choi, J.-Y. Lee and J.-I. Yun, Strontium isotope analysis using laser-induced breakdown spectroscopy and molecular laser-induced fluorescence at various atmospheric conditions, Spectrochim. Acta, Part B, 2022, 192, 106416 CrossRef CAS.
- S.-U. Choi, S.-C. Han, J.-Y. Lee and J.-I. Yun, Isotope analysis of iron on structural materials of nuclear power plants using double-pulse laser ablation molecular isotopic spectrometry, J. Anal. At. Spectrom., 2021, 36(6), 1287–1296 RSC.
- R. E. Russo, A. A. Bol'shakov, X. Mao, C. P. McKay, D. L. Perry and O. Sorkhabi, Laser ablation molecular isotopic spectrometry, Spectrochim. Acta, Part B, 2011, 66(2), 99–104 CrossRef.
- M. Lackner, Tunable diode laser absorption spectroscopy (TDLAS) in the process industries—a review, Rev. Chem. Eng., 2007, 23(2), 65–147 Search PubMed.
- V. Lebedev, J. H. Bartlett, A. Malyzhenkov and A. Castro, Micro-channel array crucible for isotope-resolved laser spectroscopy of high-temperature atomic beams, Rev. Sci. Instrum., 2017, 88(12), 126101 CrossRef PubMed.
- V. Lebedev, J. H. Bartlett and A. Castro, Isotope-resolved atomic beam laser spectroscopy of natural uranium, J. Anal. At. Spectrom., 2018, 33(11), 1862–1866 RSC.
- W. Wei, I. Savukov and A. Castro, Application of saturation absorption spectroscopy to study the hyperfine structure of 235U and accurate 235U/238U isotope ratio determinations at 861.031 nm, J. Anal. At. Spectrom., 2021, 36(9), 1912–1920 RSC.
- S. Lin, J. Chang, J. Sun and P. Xu, Improvement of the Detection Sensitivity for Tunable Diode Laser Absorption Spectroscopy: A Review, Front. Phys., 2022, 10, 853966 CrossRef.
- K. J. Ross and B. Sonntag, High-temperature metal atom beam sources, Rev. Sci. Instrum., 1995, 66(9), 4409–4433 CrossRef CAS.
- P. J. Chantry, Doppler broadening in beam experiments, J. Chem. Phys., 1971, 55(6), 2746–2759 CrossRef CAS.
- R. C. Miller and P. Kusch, Velocity distributions in potassium and thallium atomic beams, Phys. Rev., 1955, 99(4), 1314 CrossRef CAS.
- N. Ahmed, A. Nadeem, M. Nawaz, S. A. Bhatti, M. Iqbal and M. A. Baig, Resistively heated high temperature atomic beam source, Rev. Sci. Instrum., 2005, 76(6), 063105 CrossRef.
- A. Pailloux, T. Alpettaz and E. Lizon, Candlestick oven with a silica wick provides an intense collimated cesium atomic beam, Rev. Sci. Instrum., 2007, 78(2), 023102 CrossRef CAS PubMed.
- J. G. King and J. R. Zacharias, Some new applications and techniques of molecular beams, Adv. Electron. Electron Phys., 1956, 8, 1–88 CAS.
- R. Senaratne, S. V. Rajagopal, Z. A. Geiger, K. M. Fujiwara, V. Lebedev and D. M. Weld, Effusive atomic oven nozzle design using an aligned microcapillary array, Rev. Sci. Instrum., 2015, 86(2), 023105 CrossRef PubMed.
- G. G. Padmabandu, G. R. Welch, I. N. Shubin, E. S. Fry, D. E. Nikonov, M. D. Lukin and M. O. Scully, Laser oscillation without population inversion in a sodium atomic beam, Phys. Rev. Lett., 1996, 76(12), 2053 CrossRef CAS PubMed.
- W. H. King, A. Steudel and M. Wilson, Optical isotope shifts in neodymium, Zeitschrift für Physik A, 1973, 265(3), 207–224 CrossRef CAS.
- S. A. Ahmad and G. D. Saksena, Isotope shift studies in neodymium spectra-I, Physica B+C, 1976, 85(1), 191–200 CrossRef.
- S. A. Ahmad and G. D. Saksena, Isotope shifts in the energy levels of the singly ionized neodymium atom, Spectrochim. Acta, Part B, 1981, 36(10), 943–950 CrossRef.
- D. F. Swinehart, The Beer-Lambert law, J. Chem. Educ., 1962, 39(7), 333 CrossRef CAS.
- V. Thomsen, D. Schatzlein and D. Mercuro, Limits of detection in spectroscopy, Spectroscopy, 2003, 18(12), 112–114 CAS.
-
W. M. Haynes, Abundance of elements in the earth's crust and in the sea, CRC Handbook of Chemistry and Physics, 2017, 97th edn, pp. 14–17 Search PubMed.
- J. H. Bartlett and A. Castro, Isotopic spectroscopy of uranium atomic beams produced by thermal reduction of uranium compounds, Spectrochim. Acta, Part B, 2019, 155, 61–66 CrossRef CAS.
- J. M. Supplee, E. A. Whittaker and W. Lenth, Theoretical description of frequency modulation and wavelength modulation spectroscopy, Appl. Opt., 1994, 33(27), 6294–6302 CrossRef CAS PubMed.
- S. Schilt, L. Thevenaz and P. Robert, Wavelength modulation spectroscopy: combined frequency and intensity laser modulation, Appl. Opt., 2003, 42(33), 6728–6738 CrossRef CAS PubMed.
- G. B. Rieker, J. B. Jeffries and R. K. Hanson, Calibration-free wavelength-modulation spectroscopy for measurements of gas temperature and concentration in harsh environments, Appl. Opt., 2009, 48(29), 5546–5560 CrossRef CAS PubMed.
- P. Kluczynski, J. Gustafsson, Å. M. Lindberg and O. Axner, Wavelength modulation absorption spectrometry—an extensive scrutiny of the generation of signals, Spectrochim. Acta, Part B, 2001, 56(8), 1277–1354 CrossRef.
- Y. Bian, S. Guo, L. Jiang, K. Tang and W. Ding, Extraction of rare earth elements from permanent magnet scraps by FeO–B2O3 flux treatment, J. Sustain. Metall., 2015, 1(2), 151–160 CrossRef.
- R. J. Konings, O. Beneš, A. Kovács, D. Manara, D. Sedmidubský, L. Gorokhov, V. S. Iorish, V. Yungman, E. Shenyavskaya and E. Osina, The thermodynamic properties of the f-elements and their compounds. Part 2. The lanthanide and actinide oxides, J. Phys. Chem. Ref. Data, 2014, 43(1), 013101 CrossRef.
Footnote |
† Present address: Glenn T. Seaborg Institute, Lawrence Livermore National Laboratory, Livermore, California 94550, United States. |
|
This journal is © The Royal Society of Chemistry 2023 |
Click here to see how this site uses Cookies. View our privacy policy here.