DOI:
10.1039/D3MA00091E
(Paper)
Mater. Adv., 2023,
4, 5273-5281
Green synthesis of Mesona Blumes gum capped silver nanoparticles and their antioxidant, antibacterial and catalytic studies†
Received
27th February 2023
, Accepted 16th September 2023
First published on 21st September 2023
Abstract
Economically viable and eco-friendly Mesona Blumes (M.B.) gum was employed to synthesize AgNPs. The synthesis parameters such as concentration of silver nitrate (AgNO3), M.B. gum, microwave irradiation time and pH effect were optimized using a UV-visible spectrophotometer. It was observed that increasing the concentration of silver nitrate (AgNO3), M.B. gum, and microwave irradiation time enhanced the formation of MB@AgNPs. Fourier transform infrared spectroscopy (FTIR), X-ray powder diffraction (XRD), and transmission electron microscopy (TEM) techniques were used to characterize the synthesized MB@AgNPs. The XRD results confirmed a face-centred cubic structure, whereas TEM analysis indicated spherical particles of size 10 nm ± 2 nm. The majority of AgNPs were smooth and spherical, and very few were irregular. The catalytic efficacy of the MB@AgNPs was studied in the presence of NaBH4 for the reduction of crystal violet (CV) and rhodamine-B (Rh-B) dyes. Rh-B dye was reduced in 300 seconds, while CV dye was reduced in 280 seconds. The inhibition zones of Staphylococcus aureus, Bacillus subtilis, Escherichia coli, and Bacillus cereus for the MB@AgNPs were 14, 12, 15, and 11 mm, respectively. The DPPH radical scavenging ability of the AgNPs was assessed using a DPPH radical scavenging assay.
1. Introduction
The textiles, paint, paper, pulp and food industries are the major consumers of colourants and dyestuffs.1 The dye effluents released in water usually contain toxic organic chemicals.2 The dyes commonly found in effluents from process industries include rhodamine-B–B, methyl orange, methyl blue, crystal violet, methylene red, etc. Light cannot penetrate the surface when dyes accumulate in the water, affecting plant photosynthesis.3 This causes the depletion of oxygen content in the water, resulting in aquatic life and plants dying.
The majority of dyes and pollutants are complex, non-biodegradable, and very difficult to decompose. Water contamination can be treated through different methods such as ultrafiltration, electrochemical, coagulation, physisorption, chemical precipitation, and ion exchange processes.4–6 A variety of natural and synthetic adsorbents can also be used to remove pollutants from wastewater, such as coconut fibres and jute, etc.7 However, the conventional methods are not feasible due to their slow process, incomplete removal of pollutants, and high operating costs etc. Therefore, the robust and efficient removal of industrial effluents and organic pollutants from water bodies is essential to maintain a healthy environment.
NaBH4, along with nanoparticles or nanocomposites, is reported as an efficient and rapid method of eliminating organic pollutants from wastewater.8,9 Several metal nanoparticles such as Ag, Au, ruthenium, nickel, Cu10,11 and metal oxide nanoparticles (ZnO, TiO2, etc.) have been used for the degradation of organic pollutants.12 It has been reported13 that GO/MOF composites can eliminate organic contaminants from wastewater by Fuhua WeiIDet et al., 2021. Recent literature shows that the use of AgNPs has remarkable potential in the degradation of organic pollutants due to their remarkable properties, including high chemical activity and unique interfacial interactions.14 In addition, the smaller size enhances the surface area, enhancing the catalytic activity. A capping agent is present on the conjugate, allowing AgNPs to disperse uniformly on the sponge surface without clumping together. Catalysts made from nanoparticles are easily separated.
Therefore, researchers have focused on the synthesis of silver nanoparticles as silver nanoparticles are extensively used in industrial, biomedical, and pharmaceutical fields.15 The synthesis of silver nanoparticles using natural and synthetic stabilization systems has been reported by various preparation methods such as chemical, physical, biological, etc.16–19 The chemical process of synthesizing silver nanoparticles is expensive and environmentally hazardous, whereas the physical method is complex, time-consuming, and requires expensive equipment. However, the biogenic synthesis of metal nanoparticles has several advantages: simplicity, less-economic, environmental friendliness, and reliability. This synthetic route also encompasses the use of several biogenic macromolecules that act as capping agents and stabilizers.20 Silver nanoparticle synthesis has also been reported using natural gums, leaf extract, and microorganisms.21 It is novel that Mesona Blumes (M.B.) gum extract was used in this study to study the synthesis of silver nanoparticles without the use of expensive or toxic techniques. The gum comprises 9.74% protein, 30.89% ash, 2.98% crude fibre, and 42.19% sugar (w/w). It contains mostly arabinose, glucose, galactose, and uronic acid as monosaccharides.22 Functional groups of the gum effectively act as capping and reducing agents for the synthesized AgNPs.23 Since biogenic silver metal nanoparticles are highly efficient, low-cost, and simple to produce, they were considered to be highly promising for wastewater remediation. Silver nanoparticles derived from plant extracts have a greater advantage regarding their catalytic properties. The microwave irradiation method is a simple and ultra-fast technique for synthesizing metal nanoparticles. Therefore, microwave irradiation (M.W.) was used to prepare AgNPs using M.B. gum. A UV-Vis spectrophotometer was used to carry out MB@AgNP optimization experiments, while FTIR, XRD, and TEM were employed to characterize the synthesized MB@AgNPs.
In addition to water contamination by dyes, people are becoming resistant to multiple drugs due to unintentional overuse of antibiotics.24 Multidrug resistance now affects the entire world and is challenging to overcome. The multidrug resistance of bacteria makes antibiotics ineffective in preventing diseases. Silver nanoparticles’ antimicrobial and anticancer properties can be useful in overcoming multidrug resistance issues.24 Therefore, in the present study, the antioxidant and antibacterial properties of the synthesized MB@AgNPs have also been tested in addition to dye degradation. It was found that bacterial cells were effectively disfunctionalized with MB@AgNPs. The synthesized MB@AgNPs were employed as a catalyst for the reduction of Rh-B and CV dyes in the presence of NaBH4. The antibacterial activity of the MB@AgNPs was evaluated against Staphylococcus aureus, Bacillus subtilis, Escherichia coli, and Bacillus cereus. In addition, the antioxidant activity of the MB@AgNPs was studied.
2. Experimental details
2.1. Materials
The gum was purchased from Telangana Girijana Cooperative Society, Telangana State, India. Analytical grade chemicals and reagents (AgNO3, NaBH4, Crystal violet and rhodamine-B dye) were used in this study, acquired from Sigma Aldrich and SD Fine-Chem Limited (Mumbai, India).
2.2. Preparation of M.B. gum extract
1 gram of M.B. gum powder was added to 100 mL of distilled water and sonicated at room temperature for 10 minutes. Whatman filter paper was used to filtrate the gum solution and utilized to synthesize AgNPs.
2.3. Biosynthesis of AgNPs with Salmalia Malabarica gum extract
15 mL of M.B. gum extract was mixed with 15 mL of 1.5 mM AgNO3 solution in a beaker. 450 Watts of microwave irradiation were applied to the reaction mixture for 160 seconds. After microwave irradiation, the mixture turned yellowish-orange colour,25 which implies the development of silver nanoparticles.
2.4. Purification of AgNPs from the mixture
The yellowish-orange suspension mixture was centrifuged at 10
000 rpm for 30 minutes. The supinated filtrate was decanted, and the residue was redispersed in Milli-Q water. A centrifugation and scattering procedure were repeated twice in order to remove the unreacted AgNO3 and Salmalia Malabarica gum extract solution from the product. The obtained purified AgNPs were dried at 60 °C in a hot air oven. The ESI† presents catalytic reduction, antioxidant, and antibacterial processes.
3. Results and discussion
3.1. UV-Visible absorption spectroscopic analysis
The synthesized MB@AgNPs were monitored with a UV-visible absorption spectrophotometer by evaluating the changes in solution colour.26 Microwave irradiation of the Ag+ ion solution results in a change in colour from colourless to yellowish orange. AgNPs are formed by the reduction of Ag+ to Ag0. The absorption peak of the MB@AgNPs was observed at 423 nm (Fig. 1a). Synthesized AgNPs were optimized using gum concentration, AgNO3 concentration, microwave irradiation time and the effect of pH. A UV-visible spectroscopy study was conducted by varying the concentration of M.B. gum solution by maintaining a constant AgNO3 concentration of 1.5 mM for 160 seconds of MWI.27 The absorbance intensity of the AgNPs at 423nm (Fig. 1a) increased with M.B. gum concentration (0.2 to 1%), implying the formation of MB@AgNPs. An increase in the concentration of M.B. gum resulted in a large quantity of phytochemicals that reduced Ag+1 to Ag0 and stabilized the AgNPs. In addition, the effect of AgNO3 concentration on MB@AgNP synthesis was investigated by varying the AgNO3 concentration (0.3–1.5 mM) by maintaining a constant concentration of M.B. solution (1%) for a MWI time of 160 s. The absorbance intensity increased (Fig. 1b) with the increase of the AgNO3 concentration, which indicated the good yield of MB@AgNPs formation in the solution. The effect of MWI time (40, 80, 120, 160 seconds at 450 W) on MB@AgNPs was investigated at 1% M.B. gum solution and 1.5 mM of AgNO3.28Fig. 2a shows that the absorbance intensity was increased with an increase in microwave irradiation. This indicates that the reducing ability (Ag+ to Ag0) of M.B. gum increased with microwave irradiation time.29 From UV-visible spectroscopic optimization studies, it is concluded that the maximum yield of AgNPs was obtained for the conditions of 160 s irradiation time with 1% M.B. gum solution and 1.5 mM of AgNO3.
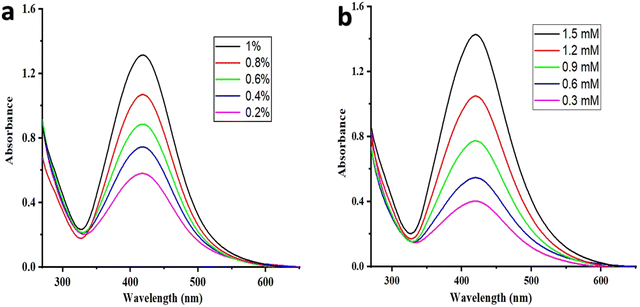 |
| Fig. 1 UV-visible absorption spectra of MB@AgNPs (a) at different concentrations of the MB gum solution and (b) at various concentrations of AgNO3 solution. | |
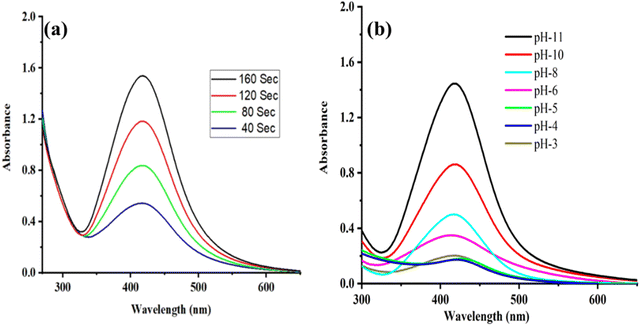 |
| Fig. 2 (a) UV-visible absorption spectrum of MB@AgNPs varying with microwave exposure time and (b) AgNPs synthesized in different pH conditions. | |
The effect of pH on AgNPs production was also investigated. Fig. 2b shows the UV-Vis spectra of AgNPs prepared at various pH values of gum solution (3, 4, 5, 6, 8, 10, and 11). When the pH value increased, the peak intensity increased gradually. This indicates the ionization of more functional groups in the gum; consequently, the rate of silver ion reduction was also increased.30 Even after a pH value of 11, the peak intensity remained constant. In the present study, a large amount of AgNPs was formed at pH = 11.
3.2. FTIR analysis
M.B. becomes a complex with Ag(I) through its hydroxyl and carbonyl functions. A strong antioxidant nature and a high reducing capacity are responsible for reducing Ag+ atoms to Ag0 atoms through these hydroxyl and carbonyl functions. In order to grow AgNPs, Ag atoms serve as nucleation sites. In these phytochemicals, oxygen-containing functional groups are bound to metal atoms on the surfaces, thereby controlling and stabilizing the size of the AgNPs. Fig. 3 shows the FTIR analysis of M.B. gum before and after AgNP synthesis, confirming this hypothesis.31 Formation and stabilization of MB@AgNPs by the hydroxyl and carbonyl groups of M.B. gum. The intensities of the hydroxyl and carbonyl groups and carboxyl peaks were found to be decreased.
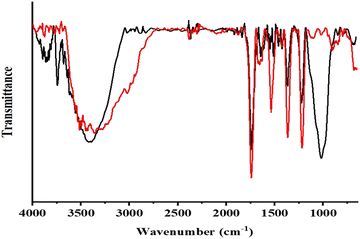 |
| Fig. 3 FTIR spectra of (a) MB gum (red colour) and (b) MB@AgNPs (black colour). | |
The FTIR spectra were recorded in the 400–4000 cm−1 range on a Shimadzu IR Affinity-1 using the KBr pellet method. FTIR measurements were used to assess the active functional groups in M.B. gum solution and their role in reducing and stabilizing AgNP formation. FTIR spectra of M.B. gum and MB@AgNPs were compared. A broad peak was observed at 3445 cm−1 in the FTIR spectrum of M.B. (Fig. 3 – red colour line), as well as a sharp stretching peak at 1750 cm−1, which indicates the presence of hydroxyl and carbonyl functional groups.32 Additionally, it exhibited strong characteristic peaks at 1500, 1400, and 1200 cm−1, which correspond to vibrations of C
C, O–H (bending), and C–O stretching. In the FTIR spectrum of MB@AgNPs (Fig. 3 – black line), the intensities of the peaks of hydroxyl and carbonyl groups and a new carboxyl peak at 1700 cm−1 were decreased. Similarly, the peak intensities of C–C (bending), O–H (bending) and C–O stretching vibrations were also decreased.33 Silver nanoparticles can be formed by binding with the functional groups of M.B. gum, as indicated by changes in the peak intensities in the spectrum of the MB@AgNPs. The reduction and stabilization of AgNPs was attributed to M.B. gum functional groups.
3.3. Powder XRD studies
A powder XRD study was performed on the MB@AgNPs to determine their crystallinity and structure. XRD (Fig. 4) showed four characteristic peaks at 2θ values of 39.65°, 45.24°, 65.14°, and 78.65° and these peaks were indexed to the (111), (200), (222) and (311) planes of the face-centered cubic structure of Ag (JCPDS-File No. 04-0783), respectively. The XRD pattern showed the (111) peak as the most intense,34 which indicates the predominant growth of AgNPs in the (111) orientation. The obtained highly intense peaks in the XRD pattern of the MB@AgNPs indicate the good crystalline nature of the synthesized MB@AgNPs.
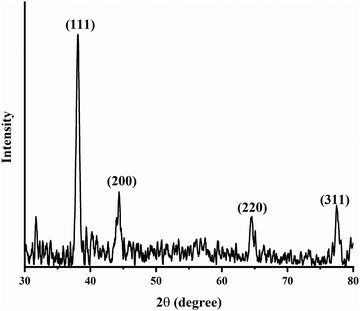 |
| Fig. 4 Powder X-ray diffraction pattern of MB@AgNPs. | |
The average crystallite size of the MB@AgNPs was calculated using Scherrer's equation25
D = kλ/β cos θ |
where λ = wavelength, θ = Bragg angle, β = full width at half maximum of the strongest peak, and k = shape-dependent Scherrer's constant.32 The calculated average crystallite size of the MB@AgNPs was found to be 8.49 nm.
3.4. TEM studies
To further confirm the size and shape of the MB@AgNPs, TEM analysis was carried out on MB@AgNPs. Fig. 5a shows TEM images of MB@AgNPs. TEM images showed spherical shape nanoparticles of the synthesized MB@AgNPs. The spherical AgNPs were not agglomerated, indicating the effective capping and stabilizing effect of the M.B. gum material. Fig. 5c shows a high-resolution TEM image of the synthesized AgNPs and clear lattice fringes, indicating that the synthesized nanoparticles were crystalline (Fig. 5b). SAED patterns of nanoparticles produced by the selected-area electron diffraction technique are shown in Fig. 5b, which confirms their highly crystalline nature. The rings are caused by diffraction from a face-centered cubic (FCC) silver plane (111), (200), (220) and (311). Fig. 5d shows the developed histogram using 150 nanoparticles. The particle size histogram of MB@AgNPs (Fig. 5b) showed an average diameter of 10 nm (± 2 nm), which is well matched with the crystallite size calculated from XRD. The TEM image showed well-dispersed MB@AgNPs with a smooth surface without any aggregation. The MB gum layer on the surface of the AgNPs prevented aggregation.35
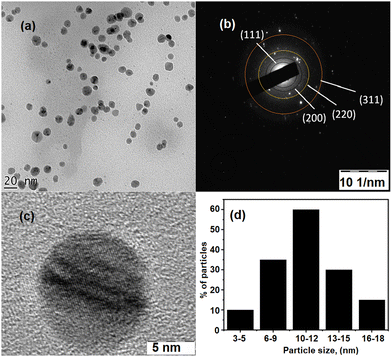 |
| Fig. 5 (a) TEM image of MB@AgNPs and (b) SAED pattern of MB@AgNPs; (c) 5 nm of MB@AgNPs; and (d) particle size distribution histogram of MB@AgNPs. | |
3.5. Catalytic reduction of crystal violet dye
In this study, MB@AgNPs are used to reduce CV dye with NaBH4 catalytically. The CV dye showed the following absorption peaks at 600, 305, and 250 nm in the UV-visible spectrophotometer.36 As shown in Fig. 6a, a change in the peak intensity was not detected even after 45 minutes with the addition of NaBH4 to the CV dye solution. This concluded that NaBH4 alone was not effective in reducing CV dye completely. A complete reduction of CV dye was achieved by adding MB@AgNPs to the above reaction. After the addition of MB@AgNPs, the intensity of the absorbance peaks (563, 305, and 250 nm) was found to be rapidly decreased (Fig. 6b). Upon completion of CV dye reduction, the blue colour of the CV dye solution turned colourless,37 which confirms the catalytic efficacy of the synthesized MB@AgNPs.38 We found that MB@AgNPs have reduced CV dye effectively (95%). The reduction reaction progress was traced using a linear relationship between ln (At/A0) and reaction time (Fig. 6c). It showed pseudo-first-order kinetics at room temperature, and the rate constant was 0.026 s−1. The influence of catalyst quantity was investigated in the 6–10 mg range. The rate constants for 6, 7, 8, 9, and 10 mg MB@AgNPs are 0.024, 0.026, 0.029, 0.033, and 0.037 s−1, respectively. As anticipated, the constant was increased with the increase of the MB@AgNPs quantity (Fig. 6d) in the reaction due to the increased number of reaction sites.
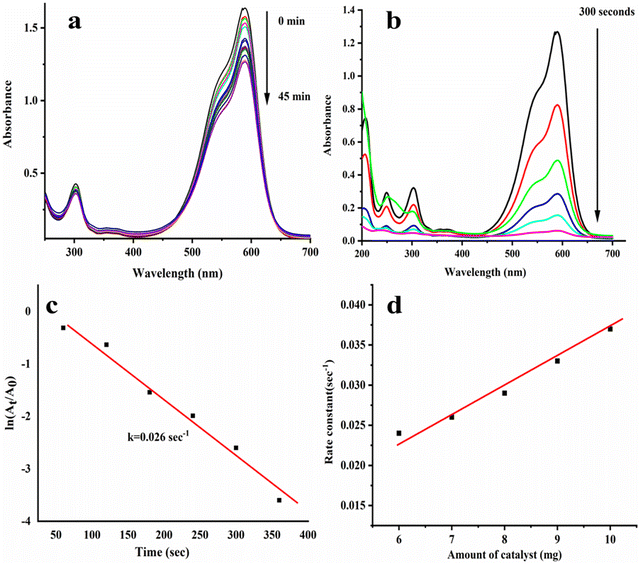 |
| Fig. 6 UV-Visible spectra of CV dye reduction: (a) in the presence of NaBH4 only, (b) in the presence of NaBH4 and MB@AgNPs, (c) the plot of log (At/A0) versus reaction time, and (d) plot of rate constant (k) versus amount of MB@AgNPs. | |
3.6. Catalytic reduction of Rh-B Dye
A wide range of applications for Rh-B is found in industry, such as printing and dyeing fabrics, paper, paint, leather, etc. Rh-B dye is soluble in water, and it is chemically basic. However, it can cause severe health problems, including irritated skin and eyes, as well as cancer. MB@AgNPs and NaBH4 were used to investigate the catalytic degradation of Rh-B dye. An absorption peak at 550 nm was observed in the Rh-B reduction when catalyzed by NaBH4. The peak at 550 nm remains constant even after 60 minutes in the case of Rh-B + NaBH4 (Fig. 7a), which suggests that NaBH4 alone could not be able to reduce Rh-B efficiently39,40 However, the Rh-B reduction was achieved quickly (in 280 seconds) in the presence of MB@AgNPs. When the reaction time was increased, the intensity of the absorption bands at 550 nm was decreased (Fig. 7b). A change in dye colour to colourless was observed.41,42 Rh-B was 95% reduced in the presence of MB@AgNPs.43 The reaction time (Fig. 7c) was calculated using ln
(At/A0), which indicates pseudo-first-order kinetics with a rate constant of 0.028 s−1 at room temperature.44 Our studies found that MB@AgNPs are more active due to their larger surface-to-volume ratio, high surface coverage, improved electron transfer properties, and efficacy in reducing the kinetic barriers of the reduction reactions.45 The role of the amount of catalyst was investigated in the range of 6–10 mg. The rate constants for 5, 6, 7, 8, 9, and 10 mg of MB@AgNPs are 0.0134, 0.023, 0.028, 0.031, 0.035, and 0.039 s−1, respectively. As anticipated, the constant was increased with the increase of the MB@AgNPs amount (Fig. 7d) due to an increase in the number of reaction sites.
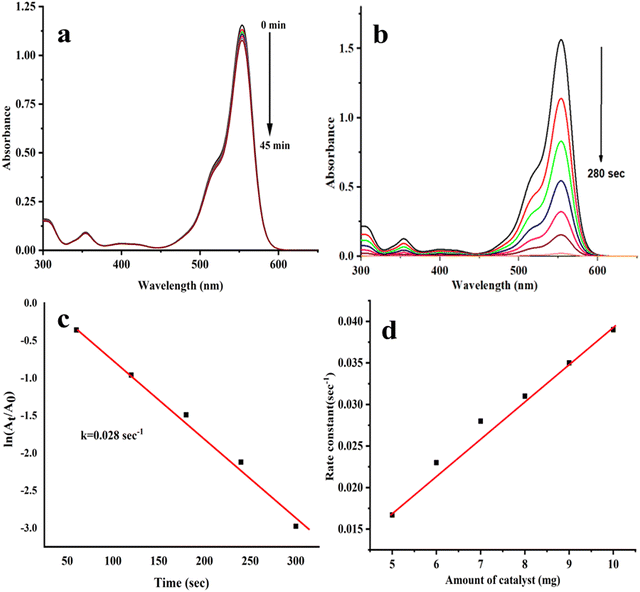 |
| Fig. 7 (a) Absorption spectra of Rh-B in the presence of NaBH4 in the absence of MB@AgNPs, (b) time-dependent UV-Vis spectra of Rh-B reduction with NaBH4 and MB@AgNP catalysts, (c) linear plot of (At/A0) vs. time for Rh-B reduction, and (d) plot of rate constant (k) versus amount of BM@AgNPs. | |
3.7. The catalytic reduction mechanism of the dyes
Fig. 8 illustrates CV and Rh-B dye's possible catalytic reduction mechanism using MB@AgNPs as the catalyst. The first stage of the reduction process results in NaBH4 adsorbing on the surface of the MB@AgNPs. These molecules include CV and Rh-B dye molecules from the analyte. By electrostatic interactions, AgNPs transfer electrons from BH4− (donor) to analytes (acceptor), and all of these reactions occur at the surface of the AgNPs. The electron transfer will reduce the analytes on the surface of the AgNPs.23 The by-products formed diffused into the bulk of the solution after being desorbed from the AgNP surface. In Table 1, we compared the catalytic activity of the MB@AgNPs with catalysts reported in the previous literature.
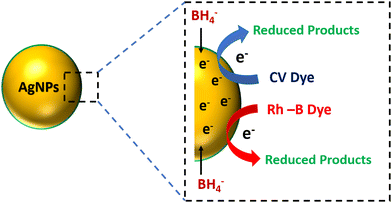 |
| Fig. 8 CV and Rh-B dye catalytic reduction mechanism by MB@AgNPs in the presence of NaBH4. | |
Table 1 Comparative study of MB@AgNPs and previously reported catalysts for CV and Rh-B reduction
Name of the dye |
Catalyst |
Time |
Ref. |
Rh-B |
Bael gum@AgNPs |
10 min |
46
|
MC-PDA-Ag |
20 min |
8
|
ANL@AuNPs |
120 min |
47
|
Colocasia esculentaAgNPs |
7 min |
48
|
MB@AgNPs
|
4 min 40 s
|
This work
|
CV |
Gumarabic@CuNPs |
20 min |
49
|
PtNPs |
15 min |
50
|
g-CN decorated with AgNPs |
8 min |
51
|
MB@AgNPs
|
5 min
|
This work
|
3.8. Recyclability of the MB@AgNP catalyst
The reusability of the MB@AgNP catalysts in various reduction processes of CV and Rh-B dyes was investigated. A vacuum oven was used to dry and clean the catalyst after being cleaned and dried in water and ethanol. MB@AgNPs can be recycled for reduction reactions of CV and Rh-B dyes, respectively, as shown in Fig. 9a and b. MB@AgNPs showed very little loss of catalytic activity after five cycles in the reduction of CV and Rh-B dyes, which indicates that the catalyst is highly stable.
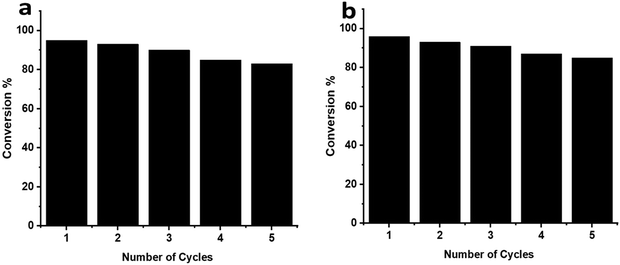 |
| Fig. 9 The catalytic performance of MB gum stabilized AgNPs within 5 cycles towards the reduction of (a) CV and (b) Rh-B dyes. | |
3.9. Antioxidant activity of MB@AgNPs
Based on ascorbic acid as the reference, MB@AgNPs are assessed for antioxidant activity using a DPPH assay. It is a simple and cost-effective assay. The synthesized MB@AgNPs were assessed for their antioxidant activity by measuring the percent inhibition of DPPH radicals in the presence of ascorbic acid.52 DPPH exhibits a high degree of hydrogen atom acceptance from antioxidant materials, so it is considered the most stable nitrogen-centred free radical. When AgNPs were added to the DPPH solution, the colour of DPPH changed, which was caused by hydrogen scavenging to form yellow-coloured DPPH. The inhibition activity of MB@AgNPs was compared to that of ascorbic acid (standard) in Fig. 10. The amount of silver nanoparticles significantly enhanced DPPH radical inhibition. Concentrations of 5, 10, 15, 20, 25 and 30 μL showed a scavenging rate of 22.5, 46.2, 55.45, 60.7, 63.6 and 62.9%, respectively. With the increased quenching rate of DPPH radicals, the obtained MB@AgNPs demonstrated that the MB@AgNPs have an enhanced antioxidation property.
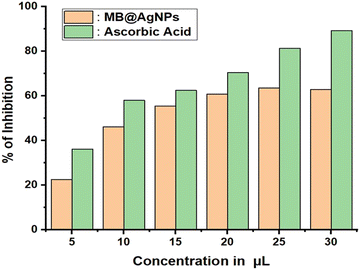 |
| Fig. 10 Antioxidant activity of MB@AgNPs. | |
3.10. Antibacterial activity of MB@AgNPs
We examined the antibacterial properties of MB@AgNPs against Gram-positive (Staphylococcus aureus & Bacillus subtilis) and Gram-negative (Escherichia coli & Bacillus cereus) strains.53 It was found that MB@AgNPs has significant antibacterial activity against pathogenic microorganisms at a concentration of 25 μL, 50 μL, 75 μL and 100 μL, respectively (Table 2). MB@AgNPs showed antibacterial activity against Escherichia coli & Bacillus cereus with a 15 and 12 mm zone of inhibition, respectively.54 Whereas it showed antibacterial activity against Staphylococcus aureus and Bacillus subtilis with inhibition zones of 14 and 13 mm, respectively.55 MB@AgNPs showed good antibacterial activity against Staphylococcus aureus and Escherichia coli, whereas Bacillus subtilis & Bacillus cereus showed moderate antibacterial activity. Bacillus subtilis and Bacillus cereus are spore-forming bacteria with thick peptidoglycan layers. These are highly resistant to temperature and drugs even though they are Gram-positive. These are the reasons for the moderate antibacterial activity of MB@AgNPs. Antibacterial activity was enhanced with an increase in the concentration of MB@AgNPs.
Table 2 Antibacterial activity of MB@AgNPs at different concentrations
S. No |
Micro Organism |
Zone of Inhibition (mm) |
25 μL |
50 μL |
75 μL |
100 μL |
Streptomycin
|
1 |
Staphylococcus aureus
|
6 mm |
8 mm |
10 mm |
14 mm |
15 mm |
2 |
Bacillus subtilis
|
— |
6 mm |
7 mm |
13 mm |
16 mm |
3 |
Escherichia coli
|
6 |
8 mm |
11 mm |
15 mm |
16 mm |
4 |
Bacillus cereus
|
5 mm |
6 mm |
8 mm |
12 mm |
15 mm |
4. Conclusions
An economically viable, ultrafast, and sustainable method was established to synthesize AgNPs by M.B. gum. Increase in the concentration of AgNO3, MB extract, microwave irradiation and pH enhanced the formation of MB@AgNPs. The XRD results confirmed a face-centered cubic structure. The TEM image of the MB@AgNPs showed spherical shape nanoparticles with an average size of 10 ± 2 nm. The MB@AgNPs scavenge 63% of free radicals, which makes them excellent antioxidants. CV and Rh-B dyes were catalytically reduced in 300 and 240 seconds, respectively, by MB@AgNPs in the presence of NaBH4. The catalytic activity of the MB@AgNPs was dose-dependent. The MB@AgNPs showed very little loss of catalytic activity after five cycles in the reduction of CV and Rh-B dyes, which indicates the high stability of the catalyst. The results showed that MB@AgNPs have remarkable catalytic activity towards the catalytic reduction of CV and Rh-B dyes. In addition, MB@AgNPs showed good antibacterial activity with inhibition zones of 14 mm and 15 mm, respectively, against Staphylococcus aureus & Escherichia coli. The MB@AgNPs showed good to moderate antibacterial activity with inhibition zones of 13 mm and 12 mm, respectively, against Bacillus subtilis & Bacillus cereus. The results showed that MB@AgNPs have significant antibacterial activity against the tested bacterial strains. The findings of this study conclude that the synthesized MB@AgNPs are efficient, reusable, eco-friendly catalysts and well-suited for environmental remediation. Based on the obtained results of this study, MB@AgNPs can be useful in biomedicine applications such as multidrug resistance and diabetic foot wound healing.
Conflicts of interest
The authors do not have any conflicts of interest.
Acknowledgements
Dr Kondaiah Seku is greatly thankful to The Ministry of Higher Education, Research, and Innovation (MOHERI), Sultanate of Oman, for the financial support from the MOHERI Research Grant (Proposal ID: MOHERI/BFP/RGP/EBR/20/253) and Dr Ahmed I. Osman wishes to acknowledge the support from the UKRI project “Advancing Creative Circular Economies for Plastics via Technological-Social Transitions” (ACCEPT Transitions, EP/S025545/1). The KSU authors express their sincere gratitude to Researchers Supporting Project number (RSP2023R368), King Saud University, Riyadh, Saudi Arabia. All data is provided in full in the results section of this paper.
References
- C. Fleischmann, M. Lievenbrück and H. Ritter, Polymers, 2015, 7, 717–746 CrossRef CAS.
- A. Khatri, M. H. Peerzada, M. Mohsin and M. White, J. Clean. Prod., 2015, 87, 50–57 CrossRef CAS.
- R. Kant, Nat. Sci., 2012, 04, 22–26 CAS.
- J. C. Cardoso, G. G. Bessegato and M. V. Boldrin Zanoni, Water Res., 2016, 98, 39–46 CrossRef CAS PubMed.
- M. M. Hassan and C. M. Carr, Chemosphere, 2018, 209, 201–219 CrossRef CAS PubMed.
- V. Buscio, M. Crespi and C. Gutiérrez-Bouzán, Desalin. Water Treat., 2016, 57, 8090–8096 CrossRef CAS.
- N. H. Phan, S. Rio, C. Faur, L. Le Coq, P. Le Cloirec and T. H. Nguyen, Carbon, 2006, 44, 2569–2577 CrossRef CAS.
- R. Kumar, A. Umar, R. Kumar, M. S. Chauhan, G. Kumar and S. Chauhan, Eng. Sci., 2021, 16, 288–300 CAS.
- A. J. Kora and L. Rastogi, Arab. J. Chem., 2018, 11, 1097–1106 CrossRef CAS.
- K. Seku, B. R. Gangapuram, B. Pejjai, M. Hussain, S. S. Hussaini, N. Golla and K. K. Kadimpati, Chem. Pap., 2019, 73, 1695–1704 CrossRef CAS.
- M. R. Axet and K. Philippot, Chem. Rev., 2020, 120, 1085–1145 CrossRef CAS PubMed.
- R. B. Rajput, S. N. Jamble and R. B. Kale, Eng. Sci., 2022, 17, 176–184 CAS.
- F. Wei, H. Zhang, Q. Ren, H. Chen, L. Yang, B. Ding, M. Yu and Z. Liang, PLoS One, 2021, 16, 1–12 Search PubMed.
- P. Balaraman, B. Balasubramanian, D. Kaliannan, M. Durai, H. Kamyab, S. Park, S. Chelliapan, C. T. Lee, V. Maluventhen and A. Maruthupandian, Waste Biomass Valorization, 2020, 11, 5255–5271 CrossRef CAS.
- S. R. Prasad, S. B. Teli, J. Ghosh, N. R. Prasad, V. S. Shaikh, G. M. Nazeruddin, A. G. Al-Sehemi, I. Patel and Y. I. Shaikh, Eng. Sci., 2021, 16, 90–128 CAS.
- K. Naseem, R. Begum, W. Wu, A. Irfan, J. Nisar, M. Azam and Z. H. Farooqi, Int. J. Environ. Sci. Technol., 2021, 18, 1809–1820 CrossRef CAS.
- I. Hussain, Z. H. Farooqi, F. Ali, R. Begum, A. Irfan, W. Wu, X. Wang, M. Shahid and J. Nisar, J. Mol. Liq., 2021, 335, 116106 CrossRef CAS.
- I. Hussain, F. Ali, M. Shahid, R. Begum, A. Irfan, W. Wu, S. Shaukat and Z. H. Farooqi, Appl. Organomet. Chem., 2021, 35, 1–13 CrossRef.
- R. Begum, G. Ahmad, J. Najeeb, W. Wu, A. Irfan, M. Azam, J. Nisar and Z. H. Farooqi, Chem. Phys. Lett., 2021, 763, 138263 CrossRef CAS.
- Y. Qu, X. Pei, W. Shen, X. Zhang, J. Wang, Z. Zhang, S. Li, S. You, F. Ma and J. Zhou, Phys. E, 2017, 88, 133–141 CrossRef CAS.
- K. Seku, B. R. Gangapuram, B. Pejjai, K. K. Kadimpati and N. Golla, J. Nanostructure Chem., 2018, 8, 179–188 CrossRef CAS.
- T. Feng, Z. B. Gu and Z. Y. Jin, Food Sci. Technol. Int., 2007, 13, 55–61 CrossRef CAS.
- K. Seku, S. S. Hussaini, M. Hussain, M. A. Siddiqui, N. Golla, D. Ravinder and B. Reddy G, Phys. E, 2022, 140, 115169–115177 CrossRef CAS.
- A. Roy, O. Bulut, S. Some, A. K. Mandal and M. D. Yilmaz, RSC Adv., 2019, 9, 2673–2702 RSC.
- K. Seku, S. S. Hussaini, B. Pejjai, M. M. S. Al Balushi, R. Dasari, N. Golla and G. B. Reddy, Chem. Pap., 2020, 1–14 Search PubMed.
- K. Kasinathan, K. Marimuthu, B. Murugesan, S. Samayanan, Y. Cai and C. Rathinam, J. Mol. Liq., 2021, 335, 116582 CrossRef CAS.
- A. Activities.
- K. Seku, S. Sulaiman Hussaini, N. Golla, G. Mangatayaru K, S. M. V. D, S. Rapolu, R. Bandi and B. Reddy G, Mater. Lett., 2020, 278, 128427–128430 CrossRef CAS.
- M. Anandan, G. Poorani, P. Boomi, K. Varunkumar, K. Anand, A. A. Chuturgoon, M. Saravanan and H. Gurumallesh Prabu, Process Biochem., 2019, 80, 80–88 CrossRef CAS.
- F. B. Araruna, T. M. de Oliveira, P. V. Quelemes, A. R. de Araújo Nobre, A. Plácido, A. G. Vasconcelos, R. C. M. de Paula, A. C. Mafud, M. P. de Almeida, C. Delerue-Matos, Y. P. Mascarenhas, P. Eaton, J. R. de Souza de Almeida Leite and D. A. da Silva, Carbohydr. Polym., 2020, 241, 115260–115269 CrossRef CAS PubMed.
- B. R. Ganapuram, M. Alle, R. Dadigala, A. Dasari, V. Maragoni and V. Guttena, Int. Nano Lett., 2015, 5, 215–222 CrossRef CAS.
- A. J. Kora and L. Rastogi, Ind. Crops Prod., 2016, 81, 1–10 CrossRef CAS.
- A. D. Mare, C. N. Ciurea, A. Man, M. Mareş, F. Toma, L. Berţa and C. Tanase, Plants, 2021, 10, 2153–2167 CrossRef CAS PubMed.
- G. Singh, M. Kumar, M. Singh and R. Vaish, J. Am. Ceram. Soc., 2021, 104, 1237–1246 CrossRef CAS.
- K. Seku, G. Bhagavanth Reddy, S. S. Hussaini, B. Pejjai, M. Hussain, D. M. Reddy, M. A. K. Khazaleh and G. Mangatayaru, Int. J. Biol. Macromol., 2022, 209, 912–922 CrossRef CAS PubMed.
- S. Ameen, M. S. Akhtar, M. Nazim and H. S. Shin, Mater. Lett., 2013, 96, 228–232 CrossRef CAS.
- A. K. Gupta, A. Pal and C. Sahoo, Dyes Pigm., 2006, 69, 224–232 CrossRef CAS.
- V. Seerangaraj, S. Sathiyavimal, S. N. Shankar, J. G. T. Nandagopal, P. Balashanmugam, F. A. Al-Misned, M. Shanmugavel, P. Senthilkumar and A. Pugazhendhi, J. Environ. Chem. Eng., 2021, 9, 105088 CrossRef CAS.
- R. W. R. Santana, A. E. B. Lima, L. K. C. de Souza, E. C. S. Santos, C. C. Santos, A. S. de Menezes, S. K. Sharma, L. S. Cavalcante, M. E. H. Maia da Costa, T. O. Sales, C. Jacinto, G. E. Luz and M. A. P. Almeida, J. Phys. Chem. Solids, 2023, 173, 111093 CrossRef CAS.
- R. Jain, M. Mathur, S. Sikarwar and A. Mittal, J. Environ. Manage., 2007, 85, 956–964 CrossRef CAS PubMed.
- S. Kumar, S. K. Sharma, R. D. Kaushik and L. P. Purohit, Mater. Today Chem., 2021, 20, 100464 CrossRef CAS.
- N. Alikhani, M. Hekmati, B. Karmakar and H. Veisi, Inorg. Chem. Commun., 2022, 139, 109351 CrossRef CAS.
- H. Veisi, P. Abassi, P. Mohammadi, T. Tamoradi and B. Karmakar, Sci. Rep., 2021, 11, 1–17 CrossRef PubMed.
- M. Ismail, M. I. Khan, S. B. Khan, K. Akhtar, M. A. Khan and A. M. Asiri, J. Mol. Liq., 2018, 268, 87–101 CrossRef CAS.
- S. Hemmati, M. M. Heravi, B. Karmakar and H. Veisi, J. Mol. Liq., 2020, 319, 114302 CrossRef CAS.
- R. Banu, D. Ramakrishna, G. B. Reddy, G. Veerabhadram and K. G. Mangatayaru, Mater. Today Proc., 2020, 43, 2265–2273 CrossRef.
- D. Baruah, M. Goswami, R. N. S. Yadav, A. Yadav and A. M. Das, J. Photochem. Photobiol., B, 2018, 186, 51–58 CrossRef CAS PubMed.
- M. Ismail, M. I. Khan, M. A. Khan, K. Akhtar, A. M. Asiri and S. B. Khan, Appl. Organomet. Chem., 2019, 33, e4971 CrossRef.
- P. Chawla, N. Kumar, A. Bains, S. B. Dhull, M. Kumar, R. Kaushik and S. Punia, Int. J. Biol. Macromol., 2020, 146, 232–242 CrossRef CAS PubMed.
- R. Dobrucka, Saudi J. Biol. Sci., 2019, 26, 31–37 CrossRef CAS PubMed.
- E. Murugan, S. Santhosh Kumar, K. M. Reshna and S. Govindaraju, J. Mater. Sci., 2019, 54, 5294–5310 CrossRef CAS.
- F. Gulbagca, S. Ozdemir, M. Gulcan and F. Sen, Heliyon, 2019, 5, e02980 CrossRef PubMed.
- D. Borah, N. Das, N. Das, A. Bhattacharjee, P. Sarmah, K. Ghosh, M. Chandel, J. Rout, P. Pandey, N. N. Ghosh and C. R. Bhattacharjee, Appl. Organomet. Chem., 2020, 34, e5597 CrossRef CAS.
- J. Sukweenadhi, K. I. Setiawan, C. Avanti, K. Kartini, E. J. Rupa and D. C. Yang, S. Afr. J. Chem. Eng., 2021, 38, 1–8 Search PubMed.
- K. Zomorodian, H. Veisi, S. M. Mousavi, M. S. Ataabadi, S. Yazdanpanah, J. Bagheri, A. P. Mehr, S. Hemmati and H. Veisi, Int. J. Nanomed., 2018, 13, 3965–3973 CrossRef CAS PubMed.
|
This journal is © The Royal Society of Chemistry 2023 |
Click here to see how this site uses Cookies. View our privacy policy here.