DOI:
10.1039/D3MA00107E
(Paper)
Mater. Adv., 2023,
4, 2365-2371
Sn2+ doping-induced large extra vibrational energy of an excited state for efficient blue emission in Cs2SnCl6:Bi†
Received
6th March 2023
, Accepted 26th April 2023
First published on 28th April 2023
Abstract
Luminescent metal halides doped with ns2-metal ions, such as 6s2-metal Bi3+, show excellent optoelectronic properties. However, the origin of the high photoluminescence quantum yield (PLQY) of Cs2SnCl6:Bi remains controversial and unclear. In this study, a series of Cs2SnCl6:Bi were synthesized by adopting different tin precursors of SnCl2/SnCl4. The samples from SnCl2 exhibited much higher PLQY than those from SnCl4, and the doping concentrations of Bi3+ had little effect on the high PLQY. When H3PO2 is added to the precursor of SnCl4 to reduce Sn4+ to Sn2+, the prepared Cs2SnCl6:Bi shows bright emission light and high PLQY. The high PLQY of Cs2SnCl6:Bi is attributed to the co-doping of Bi3+ and Sn2+. [SnCl4]2− and two VCl induce a large extra vibrational energy of the heavy localized excitons from [BiCl5]2− to enhance the efficiency of radiative recombination for high PLQY. This work deepens the understanding of the luminescence mechanism of Cs2SnCl6:Bi and supplies significant references for developing more perovskite materials with outstanding luminescence.
Introduction
Luminescent metal halides have attracted the attention of many researchers. Due to their superior optical properties, such as tunable emissions, high photoluminescence quantum yield (PLQY) and long diffraction length, Pb-based halides show promising perspective in LEDs, solar cells, photodetectors and X-ray imaging.1–11 However, the toxicity of the heavy metal Pb limits the development of practical applications.12,13 Accumulated Pb in human body can cause numerous brain-related symptoms, such as intellectual disability. Lead-free metal halides as substitutions have become a research tendency in recent years. Tin (Sn), which also has the characteristics of ns2 electrons and octahedral coordination, is a potential replacement for Pb. CsSnX3 exhibits tunable emission light through changing the ratio of halogens, which is similar to the properties of CsPbX3.14–16 However, CsSnX3 is unstable in air conditions, in which Sn2+ is easily oxidized to Sn4+, causing severe decomposition. Therefore, nontoxic and stable perovskite alternatives must be urgently developed.
Recently, many lead-free metal halides have been explored, such as Cs2NaInCl6, Cs2AgInCl6, Cs3Bi2I9, Cs3Cu2I5 and Cs2ZrCl6, owing to their excellent optoelectronic properties and stable structures.17–23 Among them, Cs2SnCl6 with its stable tetravalent cation is considered as a promising candidate for photoelectric applications.24,25 The Cs2SnCl6 crystal is derived from the three-dimensional CsSnCl3 perovskite, which periodically removes half of the Sn atoms at the center of each [SnCl6]2− octahedron, forming a vacant-ordered double perovskite structure (space group Fm
m). The [SnCl6]2− octahedral structure is isolated by a Cs+ cation. Pure Cs2SnCl6 exhibits poor photoluminescence property, but it is an excellent host material. Cs2SnCl6 is a zero-dimensional structure, and it can localize the excitons for the luminescent centers to achieve high effective emissions. Through doping with a luminescent center, such as Bi3+, Sb3+ and Te4+, Cs2SnCl6 can exhibit excellent luminescence with tunable emission wavelength.26–29 Sb3+-doped Cs2SnCl6 has been reported to emit orange light with a photoluminescence quantum yield (PLQY) of 37%, and Bi3+ doping resulted in blue light emission with a PLQY of 79%.30–32 The Bi3+/Te4+ co-doped perovskite derivative Cs2SnCl6 shows highly efficient and dual-band-tunable white-light emission owing to the strong electron-phonon coupling and efficient energy transfer.33–35
Interestingly, when Cs2SnCl6:Bi is synthesized by adopting SnCl2 as a precursor of the Sn source, Cs2SnCl6:Bi can get a high PLQY of ∼78%. However, if using SnCl4 as a precursor to synthesize Cs2SnCl6:Bi, the PLQY of the prepared Cs2SnCl6:Bi is below 30%. The underlying reason for this phenomenon remains unclear. Motivated by this, we synthesized a series of Bi3+-doped Cs2SnCl6 samples using SnCl2 or SnCl4 as the precursor under different conditions. Through comparing the photophysical properties of the Cs2SnCl6 samples with different Bi3+ doping content, we find that the samples from SnCl2 have much higher PLQY values than those from SnCl4, and the doping content of Bi3+ has little effect on the high PLQY. When some reductive H3PO2 is added in the precursor solution with SnCl4, the prepared Cs2SnCl6:Bi shows brighter emission light and higher PLQY than the sample without H3PO2. H3PO2 provides a reducing environment and reduces Sn4+ to Sn2+ during the reaction, and XPS spectra proved the existence of Sn2+ in Cs2SnCl6:Bi synthesized with H3PO2. [SnCl4]2− and two VCl induce the local lattice distortion, which can cause heavy localized excitons of [BiCl5]2−. The localized excitons make for a large extra vibrational energy to reduce the non-radiative transition and enhance the efficiency of radiative recombination. This work deepens the understanding of the luminescence mechanism of Cs2SnCl6:Bi, but also introduces novel ideas and approaches to develop more perovskite materials with outstanding luminescent properties.
Results and discussion
As shown in the experimental processes in Fig. 1a, SnCl2 or SnCl4, CsCl and BiCl3 were added to the autoclave containing hydrochloric acid. The autoclave was heated in the oven at 180 °C for 10 hours to obtain Cs2SnCl6:Bi single crystals. As shown in Fig. 1b, the single crystals show regular hexagonal shape, and the size is about several to tens of micrometers, indicating good crystallinity. EDS mapping images show that Cs, Sn, Cl, and Bi are evenly distributed in Fig. 1d, meaning Bi was successfully doped into the lattice of Cs2SnCl6. Through changing the doping content of Bi and the Sn source from SnCl2 or SnCl4, a series of Cs2SnCl6 samples were prepared. The actual doping amount of Bi was tested by inductively coupled plasma optical emission spectrometry (ICP-OES), and the element contents were given in Table 1. The samples are named as Snx+:yBi (Snx+ represents that the sample is synthesized by SnCl2 or SnCl4 in the raw materials; y represents the actual doping concentration of Bi3+ replacing the site of Sn4+). As shown in Fig. 1c, all of the samples are confirmed to be pure Cs2SnCl6 with space group (PDF #70-2413). The position of the diffraction peak gradually shifted to a low angle as the concentration of doped Bi3+ increased, which indicates that Bi3+ was doped into the lattice of the Cs2SnCl6 crystal. Rietveld refinement of the samples of Sn2+:0.08% Bi and Sn4+:0.04% Bi were performed to acquire information on the crystal structure, as shown in Fig. S1 (ESI†). Sn2+:0.08% Bi and Sn4+:0.04% Bi are in the Fm
m space group. The lattice parameters of Sn2+:0.08% Bi are a = b = c = 10.388 Å, α = β = γ = 90°, as the lattice parameters of Sn2+:0.08% Bi are a = b = c = 10.386 Å, α = β = γ = 90°. Because the radius of the Bi3+ ion (1.03 Å for coordination number (CN) = 6) is larger than that of the Sn4+ ion (0.69 Å for CN = 6), Sn2+:0.08% Bi has larger lattice parameters that Sn4+:0.04% Bi. Therefore, these results further verified that the single phase was successfully obtained and the crystal structure was unchanged with the introduction of Bi3+ ions. Although the feeding amount of BiCl3 was from 1% to 8%, the actual doping contents of Bi3+ for all samples are at a very low level, less than 2%. In Table 1, all samples synthesized by SnCl2 exhibit a higher PLQY than those by SnCl4, as shown in Fig. 2a (the values of PLQY were calculated according to the PL spectra in Fig. S2, ESI†). PLQY of the samples synthesized by the SnCl2 precursor solution were above 50%, and did not change with the increase of the Bi3+ doping contents. Conversely, the PLQY of the samples synthesized by the SnCl4 precursor was varied with Bi3+ doping concentration, and could not reach 50%. Thus, the kind of tin halides precursor was the major factor for the high PLQY, and the Bi3+ doping content was a minor factor and had little effect on the high PLQY. In Fig. 2b and Fig. S3 (ESI†), all of the samples of Cs2SnCl6:Bi have a broad emission peak at 452 nm, and the optimal excitation wavelength of all samples is 349 nm. Moreover, when the mixture precursors of SnCl2 and SnCl4 were used, the PLQY of the prepared samples was enhanced from 12.53 to 49.38% with the increase of the SnCl2 proportion in the precursor, as shown in Fig. S4 (ESI†). In order to research the reason for the different PLQY, we chose two samples of Sn2+:0.08%Bi and Sn4+:0.04%Bi, which have almost the same amount of doping Bi, for photophysical performance testing and analysis. In Fig. 2b, comparing the PL spectra of Sn2+:0.08%Bi and Sn4+:0.04%Bi, the shape and center position of the emission peak are the same, whereas the intensity is different. The inset photograph shows that Sn2+:0.08%Bi emits brighter blue light under UV light (365 nm) than Sn4+:0.04%Bi. The PL decay curves of Sn2+:0.08%Bi and Sn4+:0.04%Bi are well fitted by a single exponential, and the lifetime values of Sn2+:0.08%Bi and Sn4+:0.04%Bi are close at 622.6 and 700.6 ns respectively (Fig. 2c). The PL spectra and lifetime values indicated that the blue emission comes from the same radiation transition. In Fig. 2d, comparing the absorption spectra of pure Cs2SnCl6, Sn2+:0.08%Bi and Sn4+:0.04%Bi, the absorption edge at 315 nm is from the host Cs2SnCl6, but Sn2+:0.08%Bi and Sn4+:0.04%Bi have a small absorption peak at 340 nm, which is attributed to the absorption of doping Bi ions. Thus, according to the above results, it can be concluded that the improvement of the radiative transition efficiency is the main reason for the improvement of PLQY in Sn2+:0.08%Bi.30 We speculate that (1) using SnCl2 as the raw material to synthesize Cs2SnCl6 may introduce some Sn2+ into the lattice because both Sn2+ and Sn4+ ions have octahedral coordination; (2) Sn2+ does not transfer energy to Bi, but only improves the radiative transition efficiency of Bi3+ due to the lattice distortion.
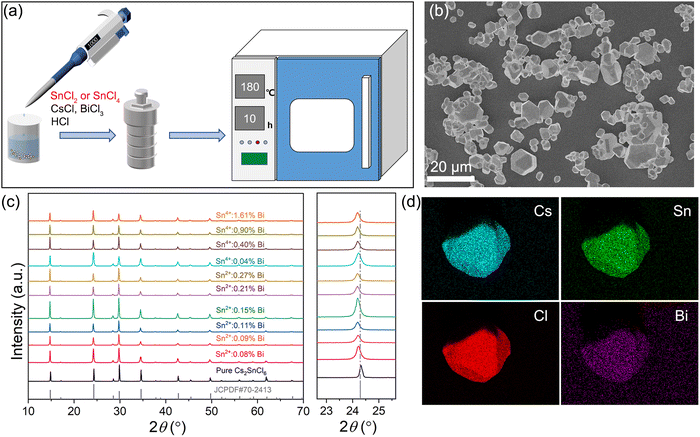 |
| Fig. 1 (a) Schematic diagram of the synthesis process. (b) SEM image of the Cs2SnCl6:Bi particles. (c) XRD patterns of Cs2SnCl6:Bi with varied doping content and different tin precursors. (d) EDS mapping for showing the distribution of individual elements Cs, Sn, Cl and Bi. | |
Table 1 Doping content of Bi and PLQY for the samples synthesized by the precursor of SnCl2 or SnCl4
Sn precursor |
SnCl4 |
SnCl2 |
Feeding Bi (%) |
0.99 |
2.91 |
4.76 |
7.41 |
0.99 |
2.91 |
4.76 |
7.41 |
9.09 |
13.04 |
Incorporated Bi (%) |
0.04 |
0.41 |
0.90 |
1.61 |
0.08 |
0.09 |
0.11 |
0.15 |
0.20 |
0.27 |
PLQY (%) |
31.8 |
28.39 |
6.46 |
7.9 |
57.29 |
53.79 |
52.39 |
51.44 |
51.78 |
52.98 |
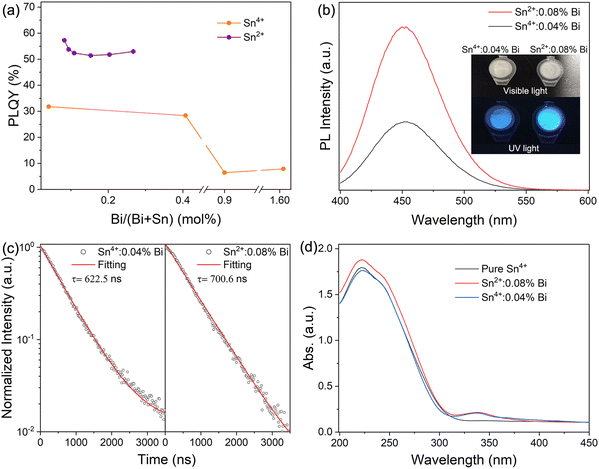 |
| Fig. 2 (a) PLQY for the samples with different doping contents of Bi3+ using SnCl2 or SnCl4 as the precursor. (b) PL intensities of Sn2+:0.08%Bi and Sn4+:0.04%Bi, which have almost the same amount of doping Bi3+; the inset of (b) is the photograph of these two samples under visible light and UV light. (c) Time-resolved PL and respective fitting curves of Sn2+:0.08%Bi and Sn4+:0.04%Bi. (d) UV-Vis absorption spectra of Sn2+:0.08%Bi, Sn4+:0.04%Bi and Pure Sn4+. | |
To further confirm the effect of Sn2+ in the precursor, we added a little H3PO2 solution into the precursor solution with SnCl4 to reduce some Sn4+ to Sn2+. The prepared Cs2SnCl6:Bi samples with/without H3PO2 were named as Sn4+:Bi@H3PO2 and Sn4+:Bi. As shown in Fig. 3a, the addition of H3PO2 does not change the XRD patterns. Sn4+:Bi@H3PO2 and Sn4+:Bi are the pure Cs2SnCl6 phase without impure phases. In Fig. 3b, the normalized PLE spectra can well coincide, meaning that H3PO2 did not change the transition level. In Fig. 3c, the PL intensity of Sn4+:Bi@H3PO2 is higher than that of Sn4+:Bi. In the inset image, the Sn4+:Bi@H3PO2 powder shows brighter blue light under UV light. Meanwhile, the lifetime values are 730.4 and 744 ns for Sn4+:Bi@H3PO2 and Sn4+:Bi, respectively. The PLQY of Sn4+:Bi@H3PO2 is up to 35.4%, which is more than twice that of Sn4+:Bi (13.7%). These results of PL, PLE and lifetime are consistent with those of Sn4+:yBi and Sn2+:yBi. H3PO2 is supposed to reduce some Sn4+ to Sn2+ in the precursor solution, and Sn2+ is then doped into the Sn4+ lattice of Cs2SnCl6 together with Bi during the process of hydrothermal synthesis, resulting in a large increase of PLQY. Therefore, the Sn2+:yBi samples using SnCl2 as the precursor are Sn2+ and Bi co-doped Cs2SnCl6.
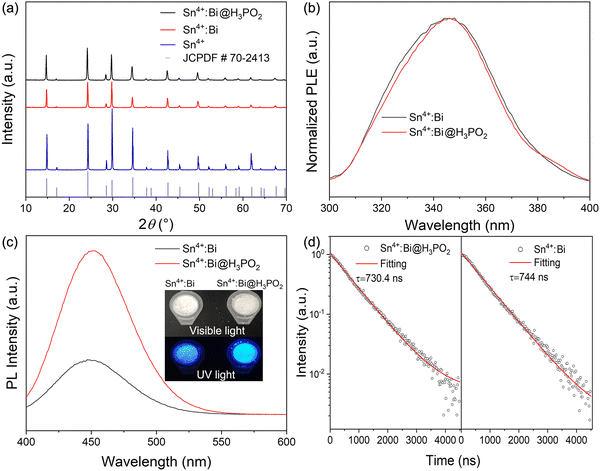 |
| Fig. 3 (a) XRD patterns of Sn4+:Bi@H3PO2, Sn4+:Bi and pure Sn4+. (b) PLE, (c) PL spectra of Sn4+:Bi@H3PO2 and Sn4+:Bi; the inset of (c) is the photograph of Sn4+:Bi@H3PO2 and Sn4+:Bi powders under visible light and UV light. (d) Time-resolved PL and respective fitting curves of Sn4+:Bi@H3PO2 and Sn4+:Bi. | |
To verify the presence of divalent tin ions in the prepared Cs2SnCl6:Bi, we performed XPS tests. Since Sn2+ on the surface of particles is easily oxidized to the tetravalent form in under air conditions, to ensure the reliability of the XPS data, we cut the single crystal in an inert atmosphere and tested the fresh cross-section. In the inert atmosphere, Sn2+ on the fresh cross-section is not oxidized and can be accurately detected. The full-scan XPS spectra in Fig. S5 (ESI†) show that all elements were detected at the cross-section. In Fig. 4a and b, the Sn 3d spectra of Sn4+:Bi@H3PO2 shows the obvious peaks of Sn2+ at binding energies of 493.65 and 485.25 eV, while that of Sn4+:Bi do not exhibit any peaks for Sn2+. This result proves that the samples prepared by using SnCl2 or SnCl4 and H3PO2 is Bi and Sn2+ co-doped Cs2SnCl6, and the improved PLQY may come from the distortion of the local structure caused by the divalent tin. The crystal diagram of the co-doped structure is shown in Fig. 5a. The Cs2SnCl6 crystal is a vacant-ordered double perovskite (space group Fm
m). [SnCl6]2− octahedral is isolated by the Cs+ cation. When SnCl2 is used as a precursor and co-doped with Bi, Sn2+ partially replaces Sn4+ to form a 4-coordinated octahedral structure [SnCl4]2− with two Cl vacancies; Bi replaces Sn4+ to form a 5-coordinated octahedral structure with a Cl vacancy. Bi belongs to the isolated luminescence center, which serves to localize the excitons and enhance radiative recombination.30 The spatial distortion of the [BiCl5]2− polyhedron will cause the change of luminescence properties, while the spatial distortion is affected by its surrounding environment. The incorporated Sn2+ in the state of [SnCl4]2− and two VCl can provide a large space to cause the local lattice distortion. When some [SnCl4]2− are adjacent with [BiCl5]2−, the heavy distortion of [BiCl5]2− occurs. This distortion induces more localized excitons by the Jahn-Teller effect,30 which can cause a large additional vibrational energy to prohibit the non-radiative transitions and enhance the efficiency of radiative recombination. To prove this inference, the extra vibrational energy is the direct evidence. Fig. S6 (ESI†) shows the change in the temperature-dependent PL intensity. Above 125 °C, Sn4+:Bi@H3PO2 still emitted light, whereas Sn4+:Bi no longer emitted light. The emission intensity of Sn4+:Bi@H3PO2 decreased more slowly than that of Sn4+:Bi with the increase of temperature. According to eqn (1),
| I = I0/(1 + e−ΔE′/kBT) | (1) |
where
I is the PL intensity, Δ
E′ is the extra vibrational energy required to produce a non-radiative transition, and
kB is the Boltzmann constant.
36 It can be determined that Sn
4+:Bi@H
3PO
2 should have a higher Δ
E′ than Sn
4+:Bi. In order to obtain the value of the extra vibrational energy, the PL spectra of the samples Sn
2+:0.08% Bi and Sn
4+:0.04% Bi were measured at different temperatures. Through fitting the PL intensity as a function of temperature as shown in Fig. S7 (ESI
†), the values of Δ
E′ for the samples Sn
2+:0.08% Bi and Sn
4+:0.04% Bi are 424 and 374 meV, respectively. Sn
2+:0.08% had a larger value of the extra vibrational energy than Sn
4+:0.04% Bi, which means that the non-radiative transitions of Sn
2+:0.08% Bi can be further prohibited to improve the efficiency of radiative transitions. As shown in
Fig. 5b and c, the incorporated Sn
2+ in the state of [SnCl
4]
2− and two V
Cl increased the energy value of Δ
E′ of [BiCl
5]
2− due to the more localized excitons, which reduces the probability of a non-radiative transition, and increases the probability of a radiative transition. Thus, Cs
2SnCl
6:Bi
3+ and Sn
2+ can finally achieve a significant improvement of PLQY.
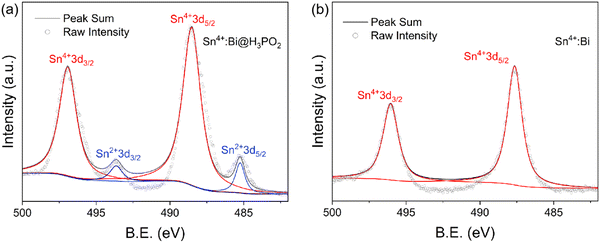 |
| Fig. 4 High-resolution XPS spectra of Sn 3d for (a) Sn4+:Bi@H3PO2 and (b) Sn4+:Bi. | |
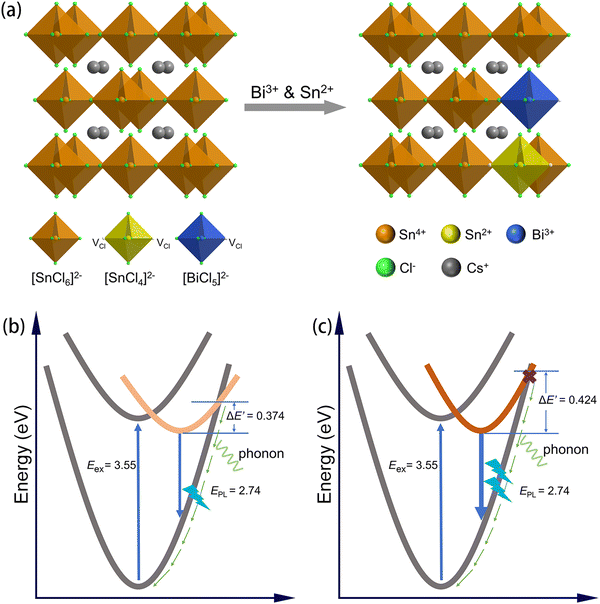 |
| Fig. 5 (a) Schematic diagram of the crystal structure of Bi3+ and Sn2+-doped Cs2SnCl6. Schematic of the energy level structure for (b) Sn4+:Bi and (c) Sn4+:Bi@H3PO2. | |
Conclusion
In summary, a series of Cs2SnCl6:Bi with different precursors have been synthesized, and the photophysical properties were investigated. Comparing Cs2SnCl6:Bi synthesized by SnCl4, Cs2SnCl6:Bi from SnCl2 shows much higher PLQY, and the doping content of Bi3+ has little effect on the high PLQY. The reductive H3PO2 in the precursor solution of SnCl4 makes the prepared Cs2SnCl6:Bi show brighter emission light and higher PLQY (35.4%) than the sample without H3PO2 (PLQY of 13.7%). This is because H3PO2 reduces Sn4+ to Sn2+ during the reaction, and the XPS spectra proved the existence of Sn2+ in the Cs2SnCl6:Bi sample synthesized with H3PO2. The sample is actually Bi3+ and Sn2+ co-doped Cs2SnCl6. [SnCl4]2− and two VCl induce the local lattice distortion, which can cause the heavy localized excitons of [BiCl5]2− to enhance the efficiency of radiative recombination. This work improves the deep understanding of the luminescence mechanism of Cs2SnCl6:Bi, and provides a new idea for developing efficient perovskite materials.
Experimental section
Materials and chemicals: cesium chloride (CsCl, Macklin, 99.9%), bismuth chloride (BiCl3, Macklin, 99.99%), tin chloride dihydrate (SnCl2·2H2O, Aladdin, 99.99%), tin tetrachloride (SnCl4, Energy Chemical, 99%), hypophosphorous acid (H3PO2, Aladdin, 50 wt% in water), and hydrochloric acid (HCl, Guangzhou Chemical Reagent Factory, 37 wt% in water) were the starting reagents. All of the chemicals were used without further purification, unless otherwise stated.
Preparation of precursors: 4 mL SnCl4 was dissolved in 17 mL HCl to prepare 2 M SnCl4 solution. A total of 12 mmol (3784.08 mg) BiCl3 was dissolved in 6 mL HCl to prepare 2 M BiCl3 solution.
Synthesis of Cs2SnCl6:Bi: an amount of 336.72 mg (2 mmol) CsCl, 225.65 mg (1 mmol) SnCl2·2H2O or 0.5 mL (1 mmol) SnCl4 precursor, and 0–50 μL (0–0.1 mmol) BiCl3 precursor were added in a polytetrafluoroethylene (PTFE) container with 15 mL of 37% HCl. The container was placed in a muffle furnace and kept at 180 °C for 10 h. Crystals were obtained by slowly cooling the solution down to room temperature over the course of 20 h. White crystals of Cs2SnCl6:Bi could be separated by immediate centrifugation, and were washed three times with ethanol.
Synthesis of Cs2SnCl6:Bi@H3PO2: an amount of 336.72 mg (2 mmol) CsCl, 500 μL (1 mmol) SnCl4 precursor, 50 μL (0.1 mmol) BiCl3 precursor and 0.05 ml H3PO2 were added in a polytetrafluoroethylene (PTFE) container with 15 mL of 37% HCl. The container was placed in a muffle furnace and kept at 180 °C for 10 h. Crystals were obtained by slowly cooling the solution down to room temperature over the course of 20 h. White crystals of Cs2SnCl6:Bi@H3PO2 could be separated by immediate centrifugation and were washed three times with ethanol.
Measurement and characterization: the phase determination of the obtained samples was carried out by powder X-ray diffraction (XRD) (D2, Bruker, using a Cu Kα rotating anode). Depth-profile XPS spectra were recorded on an AXIS Supra, using an X-ray beam focused to an area of 2 mm2 on the sample, and with an etching at the rate of 1 nm for 35 s. The PL, PLE, PLQY spectra and decay curve collection were recorded on an Edinburgh Instruments FS5 spectrometer equipped with a Xenon lamp and an integrating sphere. Inductively coupled plasma optical emission spectrometry (ICP-OES) was performed by using an atomic emission spectrometer (Aglient 5110). UV-vis absorption spectra were collected with a UV-3600i Plus spectrometer.
Conflicts of interest
There are no conflicts to declare.
Acknowledgements
This work was financially supported by the National Natural Science Foundation of China (Grant No. 61874074), Science and Technology Project of Shenzhen JCYJ20220531100815034, Technology and Innovation Commission of Shenzhen (20200810164814001), Natural Science Foundation of Guangdong Province (General Program, Grant No. 2022A1515012055 and 2023A1515030014), and Shenzhen Fundamental Research Projects (Grant No. JCYJ20220531103411026).
References
- M. V. Kovalenko, L. Protesescu and M. I. Bodnarchuk, Science, 2017, 358, 745–750 CrossRef CAS PubMed.
- Y. Wei, Z. Cheng and J. Lin, Chem. Soc. Rev., 2019, 48, 310–350 RSC.
- K. Lin, J. Xing, L. N. Quan, F. P. G. de Arquer, X. Gong, J. Lu, L. Xie, W. Zhao, D. Zhang, C. Yan, W. Li, X. Liu, Y. Lu, J. Kirman, E. H. Sargent, Q. Xiong and Z. Wei, Nature, 2018, 562, 245–248 CrossRef CAS PubMed.
- J. Chen, J. Wang, X. Xu, J. Li, J. Song, S. Lan, S. Liu, B. Cai, B. Han, J. T. Precht, D. Ginger and H. Zeng, Nat. Photonics, 2021, 15, 238–244 CrossRef CAS.
- M. Jeong, I. W. Choi, E. M. Go, Y. Cho, M. Kim, B. Lee, S. Jeong, Y. Jo, H. W. Choi, J. Lee, J.-H. Bae, S. K. Kwak, D. S. Kim and C. Yang, Science, 2020, 369, 1615–1620 CrossRef CAS PubMed.
- Y. Wang, M. I. Dar, L. K. Ono, T. Zhang, M. Kan, Y. Li, L. Zhang, X. Wang, Y. Yang, X. Gao, Y. Qi, M. Grätzel and Y. Zhao, Science, 2019, 365, 591–595 CrossRef CAS PubMed.
- L. Li, S. Ye, J. Qu, F. Zhou, J. Song and G. Shen, Small, 2021, 17, e2005606 CrossRef PubMed.
- W. Wang, D. Zhao, F. Zhang, L. Li, M. Du, C. Wang, Y. Yu, Q. Huang, M. Zhang, L. Li, J. Miao, Z. Lou, G. Shen, Y. Fang and Y. Yan, Adv. Funct. Mater., 2017, 27, 1703953 CrossRef.
- Y. C. Kim, K. H. Kim, D. Y. Son, D. N. Jeong, J. Y. Seo, Y. S. Choi, I. T. Han, S. Y. Lee and N. G. Park, Nature, 2017, 550, 87–91 CrossRef CAS PubMed.
- S. Fang, G. Li, H. Li, Y. Lu and L. Li, Chem. Commun., 2018, 54, 3863–3866 RSC.
- S. Fang, G. Li, Y. Lu and L. Li, Chem. – Eur. J., 2018, 24, 1898–1904 CrossRef CAS PubMed.
- Q. A. Akkerman, G. Rainò, M. V. Kovalenko and L. Manna, Nat. Mater., 2018, 17, 394–405 CrossRef CAS PubMed.
- I. Infante and L. Manna, Nano Lett., 2021, 21, 6–9 CrossRef CAS PubMed.
- Q. Liu, J. Yin, B. B. Zhang, J. K. Chen, Y. Zhou, L. M. Zhang, L. M. Wang, Q. Zhao, J. Hou, J. Shu, B. Song, N. Shirahata, O. M. Bakr, O. F. Mohammed and H. T. Sun, J. Am. Chem. Soc., 2021, 143, 5470–5480 CrossRef CAS PubMed.
- T. C. Jellicoe, J. M. Richter, H. F. Glass, M. Tabachnyk, R. Brady, S. E. Dutton, A. Rao, R. H. Friend, D. Credgington, N. C. Greenham and M. L. Bohm, J. Am. Chem. Soc., 2016, 138, 2941–2944 CrossRef CAS PubMed.
- L. J. Chen, C. R. Lee, Y. J. Chuang, Z. H. Wu and C. Chen, J. Phys. Chem. Lett., 2016, 7, 5028–5035 CrossRef CAS PubMed.
- W. Ke and M. G. Kanatzidis, Nat. Commun., 2019, 10, 965 CrossRef PubMed.
- B. Zhou, Z. Liu, S. Fang, H. Zhong, B. Tian, Y. Wang, H. Li, H. Hu and Y. Shi, ACS Energy Lett., 2021, 6, 3343–3351 CrossRef CAS.
- S. Fang, Y. Wang, H. Li, F. Fang, K. Jiang, Z. Liu, H. Li and Y. Shi, J. Mater. Chem. C, 2020, 8, 4895–4901 RSC.
- S. Fang, B. Zhou, H. Li, H. Hu, H. Zhong, H. Li and Y. Shi, Adv. Opt. Mater., 2022, 10, 2200605 CrossRef CAS.
- Y. Lin, Y. Jing, J. Zhao, Q. Liu and Z. Xia, Chem. Mater., 2019, 31, 3333–3339 CrossRef.
- K. Han, J. Qiao, S. Zhang, B. Su, B. Lou, C. Ma and Z. Xia, Laser Photonics Rev., 2023, 17, 2200458 CrossRef CAS.
- J. Zhou, J. Luo, X. Rong, P. Wei, M. S. Molokeev, Y. Huang, J. Zhao, Q. Liu, X. Zhang, J. Tang and Z. Xia, Adv. Opt. Mater., 2019, 7, 1900139 CrossRef.
- H. Zhu, Y. Pan, C. Peng, Y. Ding, H. Lian, J. Lin and L. Li, Small, 2023, 2300862 CrossRef PubMed.
- Z. Hu, K. Nie, X. Wang, X. Duan, R. Zhou, M. Wu, X. Ma, X. Zhang, L. Wang, L. Mei and H. Wang, Nanoscale, 2023, 15, 4893 RSC.
- R. Zeng, K. Bai, Q. Wei, T. Chang, J. Yan, B. Ke, J. Huang, L. Wang, W. Zhou, S. Cao, J. Zhao and B. Zou, Nano Res., 2020, 14, 1551–1558 CrossRef.
- H. Arfin and A. Nag, J. Phys. Chem. Lett., 2021, 12, 10002–10008 CrossRef CAS PubMed.
- A. Yan, K. Li, Y. Zhou, Y. Ye, X. Zhao and C. Liu, J. Alloys Compd., 2020, 822, 153528 CrossRef CAS.
- M. Jin, W. Zheng, Z. Gong, P. Huang, R. Li, J. Xu, X. Cheng, W. Zhang and X. Chen, Nano Res., 2022, 15, 6422–6429 CrossRef CAS.
- Z. Tan, J. Li, C. Zhang, Z. Li, Q. Hu, Z. Xiao, T. Kamiya, H. Hosono, G. Niu, E. Lifshitz, Y. Cheng and J. Tang, Adv. Funct. Mater., 2018, 28, 1801131 CrossRef.
- J. Li, Z. Tan, M. Hu, C. Chen, J. Luo, S. Li, L. Gao, Z. Xiao, G. Niu and J. Tang, Front. Optoelectron., 2019, 12, 352–364 CrossRef.
- Y. Jing, Y. Liu, J. Zhao and Z. Xia, J. Phys. Chem. Lett., 2019, 10, 7439–7444 CrossRef CAS PubMed.
- W. Zhang, W. Zheng, L. Li, P. Huang, Z. Gong, Z. Zhou, J. Sun, Y. Yu and X. Chen, Angew. Chem., Int. Ed., 2022, 61, e202116085 CAS.
- Y. Zhong, Y. E. Huang, T. Deng, Y. T. Lin, X. Y. Huang, Z. H. Deng and K. Z. Du, Inorg. Chem., 2021, 60, 17357–17363 CrossRef CAS PubMed.
- S. Das Adhikari, C. Echeverria-Arrondo, R. S. Sanchez, V. S. Chirvony, J. P. Martinez-Pastor, S. Agouram, V. Munoz-Sanjose and I. Mora-Sero, Nanoscale, 2022, 14, 1468–1479 RSC.
- S. Li, J. Luo, J. Liu and J. Tang, J. Phys. Chem. Lett., 2019, 10, 1999–2007 CrossRef CAS PubMed.
|
This journal is © The Royal Society of Chemistry 2023 |
Click here to see how this site uses Cookies. View our privacy policy here.