DOI:
10.1039/D3MD00116D
(Review Article)
RSC Med. Chem., 2023,
14, 1446-1471
Antibacterial activities of anthraquinones: structure–activity relationships and action mechanisms
Received
12th March 2023
, Accepted 24th May 2023
First published on 10th July 2023
Abstract
With the increasing prevalence of untreatable infections caused by antibiotic-resistant bacteria, the discovery of new drugs from natural products has become a hot research topic. The antibacterial activity of anthraquinones widely distributed in traditional Chinese medicine has attracted much attention. Herein, the structure and activity relationships (SARs) of anthraquinones as bacteriostatic agents are reviewed and elucidated. The substituents of anthraquinone and its derivatives are closely related to their antibacterial activities. The stronger the polarity of anthraquinone substituents is, the more potent the antibacterial effects appear. The presence of hydroxyl groups is not necessary for the antibacterial activity of hydroxyanthraquinone derivatives. Substitution of di-isopentenyl groups can improve the antibacterial activity of anthraquinone derivatives. The rigid plane structure of anthraquinone lowers its water solubility and results in the reduced activity. Meanwhile, the antibacterial mechanisms of anthraquinone and its analogs are explored, mainly including biofilm formation inhibition, destruction of the cell wall, endotoxin inhibition, inhibition of nucleic acid and protein synthesis, and blockage of energy metabolism and other substances.
1. Introduction
Currently, clinicians are still treating bacteria or fungi causing infections with antibiotics;1,2 however, antibiotics have many serious adverse effects.3,4 Antibiotics are exerting antibacterial efficacy, while pathogenic bacteria are constantly adapting to them, resulting in drug resistance. Coupled with the misuse of drugs, the failure of existing antibiotics leads to the subsequent creation of some superbugs.5,6 The emergence of multidrug-resistant bacteria and antimicrobial resistance genes has become a global medical challenge, which threatens human health and causes huge economic losses to livestock breeding and other industries.7–9 Accordingly, a search for new drugs to combat multidrug-resistant bacteria is highly urgent.10,11 Throughout the history of the discovery of many antimicrobials, natural products as a main source, such as quinones, coumarins, flavonoids, macrolides, xanthones, etc., have attracted the attention of many scholarsapos due to their low toxicity, wide distribution and high activity, which are highly valuable for research and development.12,13
Anthraquinone and its derivatives are widely distributed in nature, e.g. plants and some microorganisms.14,15 Anthraquinones including aloe rhodopsin, aloe glycosides, and rhodo are the active ingredients of many Chinese herbal medicines such as Rheum palmatum L., Fallopia multiflora Harald, and Reynoutria japonica Houtt.16,17 Aloe emodin displayed anti-inflammatory, anti-tumor and antibacterial activities.18–20 Structurally, aloin similar to aloe emodin has antibacterial activity along with neuroprotective and nephroprotective effects.21–23 In addition to antibacterial activities, anthraquinones exhibit antitumor and antidiabetic activities,24,25 highlighting that they are extremely valuable for medicinal research and development. Meanwhile, the marketed anthraquinone antibiotics, such as fluoroquinolones, have a wide antibacterial spectrum, strong antibacterial activity, and stable chemical properties. The broad-spectrum and potent antibacterial activity of tetracycline, the most widely used synthetic antibiotic in the world, has enormously contributed to controlling bacterial infection. In recent years, anthraquinone derivatives with an adjustable activity and selectivity have emerged, and their major advantages are unique: (1) low production cost; (2) the accessibility of diverse structural variations with biologically relevant parts; (3) drug resistance is hard to develop, providing a novel direction for developing selective antibacterial drugs.
Anthraquinone is a kind of polycyclic compound with a quinone structure, that is, it contains a cyclohexadiene diketone or cyclohexadiene dimethyl structure. Its parent nucleus is 9,10-anthraquinone, on which there are methyl, hydroxyl, carboxyl, methoxy and amino substituents. Anthraquinone-type compounds include anthraquinone derivatives and reduction products of different degrees. Anthraquinone is generally divided into the alizarin type and rhein type.24 According to the degree of reduction, anthraquinone can be further divided into three categories: anthraquinone derivatives, anthrone derivatives and anthracenol derivatives (Fig. 1). In terms of anthraquinone parent cores, anthraquinones can be categorized into single anthraquinone, double anthraquinone and anthraquinone glycoside.25,26 With the discovery of more and more plant extracts with antimicrobial activity, anthraquinones identified hold greater potential in developing antimicrobial agents. Although some reviews have documented the pharmacological activity of anthraquinones, few reports are provided regarding their structure and antibacterial activity relationships and action mechanisms.27,28 Therefore, in this review, we will firstly introduce the relationship of the structure and activity of anthraquinone derivatives according to different classifications of anthraquinones, summarized in Fig. 2 and 4–6, and then the antibacterial mechanisms and toxicity profiles of anthraquinones will be systematically discussed. Through the analysis and summary of the structure–activity relationships and antibacterial mechanisms, we hope to provide a meaningful guideline for designing and finding more efficient, low-toxicity and safer anthraquinone-based antibacterial agents.
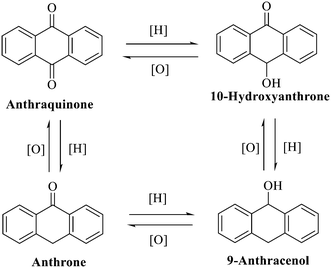 |
| Fig. 1 Interconversion of the anthraquinone parent nucleus with different redox degrees. | |
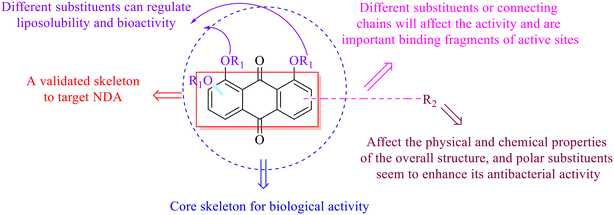 |
| Fig. 2 The structure–activity relationships of anthraquinones. | |
2. Structure and antibacterial activity relationships of anthraquinones
2.1 Monoanthracene nuclei
2.1.1 Anthraquinones.
2.1.1.1 Rhein-type anthraquinones.
The hydroxyl groups in this kind of anthraquinone are distributed on the benzene rings of both sides. Emodin (1) was first discovered at the end of the 18th century. The physiological activity of emodin determines its use in medical treatment, health care and daily chemicals. The antibacterial activity of emodin has been shown to have broad-spectrum antibacterial activity,29 such as against B. subtilis and S. aureus.30 It was also confirmed by experiment31 that, superior to that of the control oxacillin (MIC = 128 μg mL−1), emodin from tigrinum exhibited significant anti-MRSA252 activity (MIC = 4 μg mL−1), and another experiment32 that 0.5–2.0 mg mL−1 emodin inhibited the in vitro activity of Streptococcus mutans and prevented it from secreting acidic substances and synthesizing glucan. The inhibitory effect of emodin on Helicobacter pylori strains SS1 and ATCC 43504 (ref. 33) was observed and an action target to inhibit Helicobacter pylori β-hydroxyacyl ACP dehydratase was found. Numerous reports on the structures and its antibacterial activity of emodin and its derivatives are listed in Tables 1 and 2, respectively, and some conclusions are reached that the broad-spectrum antibacterial effects of emodin were achieved by interacting with biofilms, interfering with DNA or protein synthesis, or inhibiting other substances.
Table 1 Structures of anthraquinones
Table 2
In vitro antibacterial activity of emodin (μg mL−1)
Strain (object) |
MIC (μg mL−1) |
Ref. |
Gram-positive bacteria |
Staphylococcus aureus
|
64 |
34
|
Staphylococcus aureus CMCC26003 |
0.125 |
44
|
Bacillus species |
0.5–2.0 |
35
|
Methicillin-resistant Staphylococcus aureus |
4 |
36 and 39 |
Bacillus subtilis
|
8–32 |
37 and 38 |
Mycobacterium tuberculosis
|
0.9 |
40
|
Bacillus cereus TISTR 687 |
16 |
41
|
Streptococcus suis strain ATCC700794 |
0.125 |
43
|
Gram-negative bacteria |
Escherichia coli
|
>128 |
34
|
Pseudomonas aeruginosa
|
>128 |
34
|
Pseudomonas aeruginosa TISTR 781 |
128 |
41
|
Salmonella typhimurium TISTR780 |
128 |
41
|
Haemophilus parasuis
|
32 |
42
|
Chrysophanol (2) is involved in a variety of biological activities, including anti-bacterial, anti-cancer, anti-virus, anti-diabetes, anti-inflammatory, anti-ulcer, anti-obesity and liver protection.45,46 The antileishmanial activity of chrysophanol against chloroquine-resistant (IC50 = 20.13 μg mL−1) and chloroquine-sensitive strains of P. falciparum (IC50 = 7.80 μg ml−1) was exhibited.47,48 Coopoosamy also observed an inhibitory effect of chlorophenol on intestinal infection in mice (IC50 = 12.70 μg ml−1).49 Its antibacterial effects proposed by recent studies may be achieved by destroying biofilms.50,51 The antibacterial activity of chrysophanol is demonstrated in Table 3.
Table 3 The in vitro antibacterial activity of chrysophanol (μg mL−1)
Strain (object) |
MIC (μg mL−1) |
Ref. |
Gram-positive bacteria |
Bacillus cereus
|
>250 |
52
|
Staphylococcus aureus
|
>250 |
52
|
Staphylococcus epidermidis
|
31.25 |
52
|
Mycobacterium tuberculosis H37Ra |
64 |
60
|
Mycobacterium tuberculosis bovis
|
64 |
60
|
Gram-negative bacteria |
Escherichia coli
|
125 |
52
|
Escherichia coli
|
3.13 |
58
|
Aeromonas hydrophila IB101 |
200 |
53
|
Aeromonas hydrophila
|
200 |
53
|
Micrococcus kristinae
|
>250 |
54
|
Proteus vulgaris
|
128 |
54
|
Enterobacter aerogenes
|
>250 |
54
|
Pseudomonas aeruginosa
|
128 |
55
|
Vibrio harveyi
|
1000 |
56
|
Neisseria gonorrhoeae
|
75 |
59
|
Fungus |
Trichophyton rubrum
|
156 |
52
|
Epidermophyton floccosum
|
625 |
52
|
Candida albicans
|
50 |
57
|
Cryptococcus neoformans
|
50 |
57
|
Aloe emodin (3) displays extensive pharmacological effects. Its antibacterial effect was accomplished by acting on the initial adhesion and proliferation of biofilm development.61,62 When bacterial cells were treated with aloe emodin, the changes of the genes related to cell thiometabolism, lysine and peptidoglycan biosynthesis and biofilm formation took place. The decrease of N-acetyltransferase (NAT) activity in the cytoplasm of Helicobacter pylori was dose-dependently associated with the increase of aloe emodin (Table 4).63 A carbon nanoparticle polymer hybrid hydrogel loaded with an aloe emodin64 quickly generated a large amount of heat and active oxygen with the help of near-infrared radiation, achieving controllable bacteriostasis. The latest research65,66 unfolded that aloe emodin-containing waterborne polyurethane was a good antibacterial agent.
Table 4 The in vitro antibacterial activity of aloe emodin (μg mL−1)
Strain (object) |
MIC (μg mL−1) |
MBC (μg mL−1) |
Ref. |
Gram-positive bacteria |
Staphylococcus aureus species |
16–32 |
64–128 |
62
|
Staphylococcus epidermidis BNCC102555 |
32 |
128 |
Staphylococcus epidermidis ATCC12228 |
4 |
16 |
Streptococcus pneumoniae
|
16 |
64 |
Rhein (4) exhibits good antibacterial activity.67 It was first reported68 that rhein can inhibit NAT activities of the bacteria Helicobacter pylori. At the concentration of 1.5–25 mg mL−1, 4 was able to inhibit staphylococcus, streptococcus,69diphtheria, Bacillus subtilis, paratyphoid bacillus, dysentery bacillus, etc. Its mechanisms included the inhibition of electron transfer in the mitochondrial respiratory chain, a strong inhibitory effect on nucleic acid and protein synthesis. Also, 4 was found to have a very good affinity for bacterial DNA/CpG DNA and was capable of inhibiting LPS-induced TNF-α release from RAW264.7 cells in a dose-dependent manner.70 When treated with rhein,71,72 the bacterial morphology was influenced, the integrity of the cell wall was disrupted, biofilm formation was blocked, and bacterial metabolism was decreased. Additionally, the bacterial glycolysis pathway was significantly affected by rhein,73,74 which was associated with an effect on the activity of type II NADH: quinone oxidoreductase (NDH-2).75,76 The corresponding antibacterial activities are summarized in Table 5.
Table 5 The in vitro antibacterial activity of rhein (μg mL−1)
Strain (object) |
MIC (μg mL−1) |
Ref. |
Gram-positive bacteria |
Staphylococcus aureus
|
4–16 |
69
|
Streptococcus mutans
|
6.25 |
77
|
Gram-negative bacteria |
Escherichia coli
|
125 |
78
|
Salmonella
|
250 |
78
|
Porphyromonas gingivalis
|
2.5 |
79
|
The antibacterial effects of emodin, rhein and aloe emodin are generally more potent than those of emodin methyl ether and chrysophanol. Structurally, these anthraquinones having the same parent nucleus are different from their substituents on C-3 and C-6. The polar substituents including –COOH, –OH and –CH2OH attached to rhein, emodin and aloe emodin are beneficial to the improved antibacterial activity, while the electron-donating groups, –OCH3 and –CH3, introduced to emodin methyl ether and chrysophanol may weaken the antibacterial activity.81 By comparing several anthraquinones against B. adolescentis,82 the stronger the polarity of the substituent is, the better the antibacterial effects occur. A similar result is postulated by the comparison of the antibacterial activities of rhein and aloe emodin.83 1,8-Dihydroxyl groups contribute to the antibacterial activity of anthraquinone derivatives due to the generation of free electrons.80 The antibacterial activity of more anthraquinone derivatives from the root extract of Huangmu Bacopa monniera demonstrated a hydroxyl group introduced on C-8 has a catalytic effect, and its removal led to a decrease in the antibacterial activity.85 However, the introduction of electron-donating groups to 1,8-dihydroxyoxanthraquinone resulted in decreased antibacterial activity, especially against MRSA.84 Interestingly, in Lee's study, two electron-donating group hydroxyl groups of 1,2-dihydroxyanthraquinone were shown to be essential in breaking the bacteria membrane,86 emphasizing an important role of the two hydroxyl groups at positions C-1 and C-2 of anthraquinone played in the antibacterial activity. The long aliphatic chain substituted on C-6 of rhodopsin enhanced its antibacterial properties, while the substitution residing in its C-2 lowered the antibacterial activity.87 The introduction of various long chains to the anthraquinone structure of emodin facilitated the compounds to disrupt the bacterial membrane and increase the antibacterial activity, probably ascribed to the enhanced lipophilicity making it easier to bind the biofilm.88 Although the presence of the long aliphatic chain in the emodin structure increased the antibacterial activity, the methoxy group introduced was not conducive to the bactericidal activity. In terms of the decreasing antimicrobial activity, the rank is 5 > 7 > 8 > 6.
In addition to the polarizability, the antimicrobial properties of anthraquinone derivatives rely on the pH of the environment and the number of hydrogen bond acceptors.89–92 Basu and Duan's research studies93,94 revealed that the antibacterial activity of 9 was generally better than that of 1, but 1 was inferior to 10. Derivative 11 where the emodin was replaced by iodine displayed improved antibacterial activity against MRSA and other strains, and its increased ability to destroy the bacterial membrane.95 The antibacterial activity of compound 12 having formaldehyde and citrin introduced in the structure of emodin was not reported.96 Comparing compounds 1–4 and 13 against the T. vaginalis G3 strain, the introduction of phenolic hydroxyls at 1,2,4 positions of the benzene ring on the same side of anthraquinone led to reduced activity.97 Compounds 1, 2 and 14, isolated from Senna macranthera roots, exhibited potential antibacterial activities against Staphylococcus aureus strains from animals suffering from mastitis infections with MIC values of 20, 90, and 90 μg mL−1,98 respectively. The design and synthesis of 20 aloe emodin derivatives similar to the structure of 15 were demonstrated in general, and the activity of four compounds was tested, suggesting improved activity ascribed to the electron donor group at positions 1 and 2 of anthraquinone.9916 with novel structural aloe-emodin azoles as a potential antibacterial agent exhibited lower toxicity and higher antibacterial activity.100 A series of emodin derivatives similar to the structure of 17 with anti-MRSA activity were designed and evaluated.101 Additionally, the target compounds 18–22 showed different levels of antifungal activity.102 Noticeably, some showed higher inhibitory activity against R. solani, in comparison with the parent compound rhein. From their preliminary structure–activity relationships, the antifungal activity of rhein amide was higher than that of rhein ester. Replacement of hydroxyl groups at positions 1 and 8 resulted in a decrease in the antibacterial activity. The hydroxyl group at the R1 position was important and necessary for the activity. Aloe emodin conjugated 23 and 24 with sulfonylhydrazone as new antibacterial regulators also highlighted that the introduction of electron-donating substituents at R2 and R3 positions could improve the activity and reduce hemolytic toxicity.103 A series of compounds similar to derivative 25 have been synthesized for antibacterial evaluation, and as a result, the existence of the methylthio substituent at C-3 and the 3,4,5-trimethoxyphenyl group at C-4 of the β-lactam ring significantly increased the antibacterial activity.104 The antibacterial activity of compounds 26–28 and purpurin (41) against S. aureus and C. albicans was evaluated, indicating that 28 and 41 are potential drugs for photodynamic antibacterial chemotherapy.105,106
2.1.1.2 Alizarin type anthraquinones.
This type of anthraquinone has hydroxyl groups distributed on one side of the benzene ring, and includes alizarin, hydroxyalizarin and pseudo hydroxyl alizarin in the traditional Chinese medicine Rubia cordifolia. Alizarin type anthraquinones, the main pharmacodynamic component of Rubia cordifolia and Morinda officinalis, have certain clinical medicinal value and wide application.107,108Rubia anthraquinones primarily originate from Rubia cordifolia Linn (Rubiaceae), including compounds 29–32.109 The antibacterial activity of compounds 33–40 was tested, displaying that 34, 35 and 40 had certain antibacterial ability.11041, one of the two chemical markers, which serves to evaluate the quality of herbal medicines in the Chinese Pharmacopoeia,111 inhibited the growth of Gram-negative and Gram-positive bacteria, Ape with IC50 values ranging from 0.3 to 23 μM.112 Some anthraquinones 42–47 had mutagenic activity against Salmonella typhimurium.113 From the above analysis, the structure–activity relationships of emodin and alizarin and their derivatives are summarized in Fig. 2.
2.1.2 Anthracenol, anthrone and their derivatives.
Anthraquinone can be reduced by zinc powder in alkaline solution to produce reduced anthraquinone and its tautomer anthraquinone. Both reduced anthraquinone and anthraquinone are unstable, the reduced anthraquinones are easily oxidized to anthrone or anthraquinone, and anthrone is easily oxidized to anthraquinone, so the reduced anthraquinones are rarely found in plants. Fresh rhubarb contains anthracene phenols, which are undetectable when stored for more than 2 years. In acidic solution, the reduced anthraquinone and its tautomer anthrone are formed. The anthraquinone derivative is relatively stable when the hydroxyl group at the meso position is condensed with a sugar to form a glycoside. Only removal of a glycosyl group easily leads to oxidation into anthraquinone, hinting an electron transfer occurring between anthracene phenol and anthrone. As shown in Fig. 3, a dissociating monoclinic oxygen-sensitive linker 9,10-dialkoxyanthracene that contains hydrogen or other carbon substituents on 9,10 sites of anthracene is an efficient, reliable, and rapid functional site for capturing singlet oxygen.114–116 Based on this feature, it can be developed into fluorescent dyes or antibacterial agents that inhibit respiratory electron transfer chains. To date, only a few reports have focused on the antibacterial activity of anthracene phenols, anthracene and its derivatives 50–52, for instance, xanthone derivative 50 was demonstrated to display a variety of biological activities and medical value.
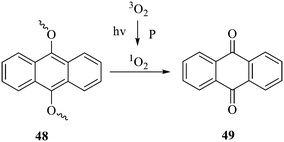 |
| Fig. 3 Cleavage mechanism of 9,10 monoalkoxy anthracene under the action of singlet oxygen. | |
The synthesis of 12 alkyl amino substituted azabenzoanthrone derivatives, like 53, was reported by the Tang research group.117 The antibacterial evaluation unfolded that compounds 53Ia–Ij exhibited strong inhibitory activity against S. aureus, B. megaterium, S. typhimurium and B. subtilis, and the activities of 53Ia and 53Ib were more potent. Compounds 54–76 are listed in Table 6. The antibacterial activity of anthrone derivatives 54–62 is discovered to be related to the number of substituents on the two aromatic rings. The hydroxyl groups of rings A and C are very important for the antibacterial activity, and alkylation of the hydroxyl groups of C-3 and C-6 decreases the antibacterial activity. The longer the alkyl chain is, the more the antibacterial activity decreases.118 Similarly, a series of alkene xanthones 63–71 extracted from Garcinia staudtii Engl. are tested against methicillin-resistant S. aureus, exhibiting strong antibacterial and immunomodulatory abilities.119
Table 6 Structures of anthraquinone derivatives
Of anthrone derivatives 72–76 synthesized, the lowest MIC was below 20 mg L−1.120 The structure–activity relationship analysis showed that the existence of two hydroxyl groups in the amine part was necessary in the anti-Helicobacter pylori activity, and the activity depended more on the structure and configuration than on the hydrophilic properties. Compounds containing a tertiary butylamine substructure displayed higher activity than the ones with an isopropylamine fragment. The structure–activity relationship of oxanthrone derivatives was not discussed here, but refer to ref. 149. The antibacterial activity of 77 and 78 was tested and found to present moderate antibacterial activity against bacteria such as B. subtilis, Bacillus cereus, S. aureus, Escherichia coli, P. aeruginosa and S. sonnei.120,121 The in vitro antibacterial activity of compounds 79–86 was evaluated, and as a result, 83 had obvious antibacterial activity against B. subtilis with a MIC value of 312 nM, and the activity of 85 against Bacillus cereus was more potent (MIC = 8.8 nM).122 Therefore, the antibacterial SAR of hydrogenated anthraquinone derivatives is summarized in Fig. 4.
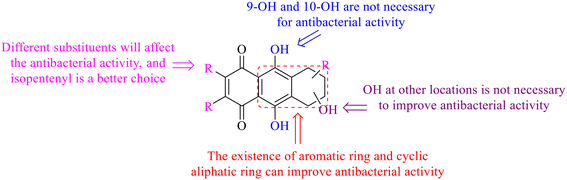 |
| Fig. 4 Antibacterial structure–activity relationship of hydrogenated anthraquinone derivatives. | |
Next, mangostin and its derivatives12387–94 have been explored. The MICs of mangostin (88) against MRSA, MSSA, VRE and VSE are 3.13, 6.25, 6.25 and 6.25 mg mL−1, respectively, along with little toxicity and few side effects. Mangostin having two di-isopentenyl scaffolds and one xanthone core exerts excellent antibacterial activity via a membrane targeting manner, implying mangostin is a promising lead for developing antibacterial candidates. Its SAR is shown in Fig. 5.124,125
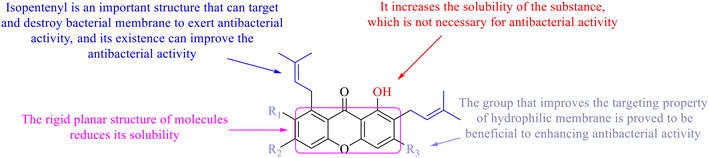 |
| Fig. 5 Antibacterial structure–activity relationship of mangostin derivatives. | |
Compound 95 showed inhibitory activity against S. aureus with the diameter of its bacteriostasis ring equal to 9.58 mm.126 Some ketinone derivatives 96–104 (ref. 127) were prepared, and 99–101 exhibited broad-spectrum antifungal effects. Through the analysis of the structure–activity relationship, the presence of the linear amine at C-1 of the thioxanthone scaffold seemingly was the pharmacological feature, while the nature of the substituent at C-4 failed to inhibit fungal growth. More than 40 anthrone derivatives were screened, and 96, 105 and 106 demonstrated antibacterial activities against a MRSA isolate with MIC values of 32–256 mg ml−1.128,129 The SARs of thioxanthone, acridone and their derivatives are summarized in Fig. 6.
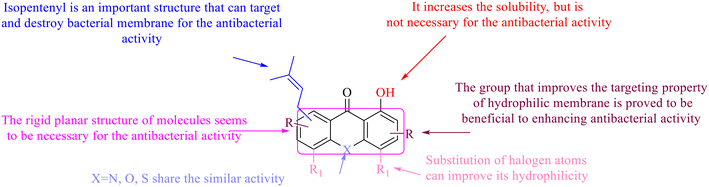 |
| Fig. 6 Structure–activity relationship of oxaanthone, thiaanthone, azaanthone. | |
Recently, cationic anthraquinone analogs (CAAs) have been demonstrated to hold great and excellent potential for antibacterial activity. CAAs 107–113 mainly exerted their antibacterial activity by disrupting the redox process. At high concentrations, these compounds also served as membrane breaking agents.130 The functional groups on N-1 played a crucial role in regulating the biological characteristics and the biological activity of these molecules. Of note, CAAs containing linear alkyl groups had good antibacterial activity, while CAAs carrying aromatic groups exhibited good anticancer activity. Additionally, when the N-1 and N-3 positions of CAAs were replaced by alkyl chains with various lengths, the structural characteristics of naphthoquinones including the core nucleus, cations, and oxygen-containing alkyl chains probably affected the antibacterial activity. Moreover, the antibacterial activity against Gram-positive bacteria was by far higher than that against the Gram-negative ones.131,132 Collectively, CAAs exhibited an adjustable activity and selectivity, opening the way to develop broad-spectrum antibiotics.
We have acquired the whole genome of the Gram-negative bacteria, but the finding does not create new antimicrobial agents. Anthraquinone derivatives serving as antibacterial agents do not benefit from the whole genome. Moderate antibacterial activities of compounds 77 and 78 towards selected Gram-positive and Gram-negative bacteria were observed. 2 can also inhibit Pseudomonas aeruginosa and Escherichia coli, and its growth inhibition zone was 18 mm.42 The MIC of aloe emodin against E. coli and P. aeruginosa ATCC 27853 was 128–259 μg ml−1.62 Interestingly, the antibacterial mechanism of anthraquinone derivatives against Gram negative or positive bacteria was demonstrated to be similar.
2.2 Binuclear anthraquinone
Two single anthraquinones are dehydrated and condensed to form double anthraquinones in two approaches. The structure of anthraquinones is different according to the different dehydration water and dehydration positions. One way is to react between positions C-4, C-5 and C-10 to remove two dihydroxides at the same time; the other way is that the dehydration condensation reaction between positions C-5, C-6 and C-7 of two molecules and removal of one water molecule form hypericin derivatives 114–123.133 Hypericin 114 is a widely-studied natural double anthraquinone from Hypericum japonicum. It is involved in strong biological activities such as antiviral, antidepressant and photodynamic activities.134 Unfortunately, only the cytotoxicity of 114–123 was reported and no data on the antibacterial activity135,136 were referred to. The xanthone dimer derivative garmoxanthone 124 showed strong inhibitory activity against MRSA ATCC43300 (MIC = 3.9 μg mL−1) and MRSA CGMCC1.12409 (ref. 137) (MIC = 3.9 μg mL−1), and exhibited moderate activity to the tested vibrio strain with MICs ranging from 15.6 to 31.2 μg mL−1. 125 and 126 with a rare C–O–C ether bond dimerization demonstrated selective antibacterial activity against Gram-positive Staphylococcus aureus.138 The structure–activity relationship of anthraquinone derivatives including 127 revealed that a long fatty chain and a methoxy group contained in the substituent could improve the antibacterial activity.139 It is noted that the SAR of binuclear anthraquinone is similar to that of mononuclear anthraquinone in the antibacterial activity.
2.3 Anthraquinone glycosides
2.3.1 Aloin-based glycosides.
Aloin, also known as Aloe vera, is a natural organic compound. Extensive attention has turned to its anti-inflammatory, anti-cancer, antibacterial and antioxidant activities. Sennosides 128–130, aloin 131 and mangiferin 132 are common anthraquinone glycosides, holding great antibacterial potential.140,141 For details of anthrone glycosylation, refer to ref. 165. The antibacterial activity of 131 and 133 against 23 kinds of bacteria and four kinds of fungi was tested. As a consequence, 133 showed a certain activity against multi-drug resistant Staphylococcus aureus (NCTC 11994) and Salmonella typhimurium (ATCC 1255) with MIC values of 0.72 and 0.18 mM, respectively.142 However, the antibacterial effect of 134 and 135 was ineffective.143 Generally speaking, the introduction of a glycosyl group improved the water solubility and the activity of anthraquinone, implying glycosylation as an effective method in the antibacterial activity. Similarly, the structure–activity relationship of anthraquinone glycosides followed that of mononuclear anthraquinone. The antibacterial mechanism of aloin as a tetracycline analogue was similar to that of aminoglycosides, inhibiting bacterial protein synthesis by blocking ribosome response sites.144 Aloin can inhibit C. neoformans and display synergistic antibacterial activity when co-administered with amphotericin B.145 Also, the activity of anthraquinone glycosides against mycobacterium146 was observed. Recently, aloe has been reported to show a significant inhibition effect on plaque formation of Porphyromonas gingivalis and Actinobacillus actinomycetes in 30 patients suffering from periodontitis.147 The detailed antibacterial activity of anthraquinone glycosides is shown in Table 7.
Table 7 The in vitro antibacterial activity of aloin and its glycosides (μg mL−1)
|
Strain (object) |
MIC (μg mL−1) |
Ref. |
Gram-positive bacteria |
Bacillus subtilis
|
240 |
148
|
Streptococcus
|
120 |
148
|
Staphylococcus aureus ML267 |
1910 |
148
|
Mycobacterium tuberculosis H37Ra |
32 |
146
|
Streptococcus sobrinus
|
2.5 |
149
|
Gram-negative bacteria |
Bacillus pumilus
|
120 |
148
|
Escherichia coli
|
60–120 |
148
|
Salmonella typhi Ty2 |
60 |
148
|
Shigella boydii D13629 |
960 |
148
|
Vibrio cholerae
|
120 |
148
|
Mycobacterium bovis
|
128 |
146
|
Fungus |
Cryptococcus neoformans
|
64 |
145
|
3. Antibacterial mechanisms and toxicity of anthraquinones
3.1 Antibacterial mechanisms of anthraquinones
3.1.1 Intervention or destruction of biofilms.
A biofilm is a special form of bacteria (or fungi) in response to adverse environments. For example, the formation of biofilms increases bacteria resistant to antibiotics.150 The process of biofilm formation is involved in many factors,151 depending on the strain, nutrient composition, and growth environment. For P. putida, the formation of biofilms was mainly controlled by the adhesion protein LapA,152–154 while the biofilm formation of P. aeroginosa mainly relied on the extracellular polysaccharides Psl and Pel.155–157 Traditionally, the formation of biofilms is considered as a five-step model (Fig. 7A1); however, some limitations of this model exist, failing to describe the biofilm complexity158–160 from industrial, natural and clinical environments. A dynamic model shown in Fig. 7A2 was later on proposed by K. Sauer.161 Up to now, due to limited technologies, it is impossible to track a single cell in the process of biofilm formation, hindering the research on formation of high-resolution biofilms.162 As a consequence, the composition required for biofilm formation and the regulation mechanism still remain unknown. Currently, the mechanisms of biofilm resistance include the following points, providing solutions to find possible countermeasures:163 1) antibacterial drug penetration barrier; 2) nutrient restriction; 3) gene phenotype change in the biofilm; 4) QS signal generation; 5) activation of tight response; 6) activation of an efflux pump system; 7) secretion of antibiotic hydrolase, etc. In view of the variability and drug resistance of biofilms, modern drugs or strategies for treating bacterial infection caused by biofilm formation are emerging. Among the reported new potential drugs, antibacterial peptides (AMPs),164 bacteriophages,165 quorum sensing inhibitors (QSIs),166 aptamers,167 nanoparticles (NPs),168 peptide nucleic acids (PNAs),169 and anthraquinone-type compounds170–172 are attractive and promising solutions.
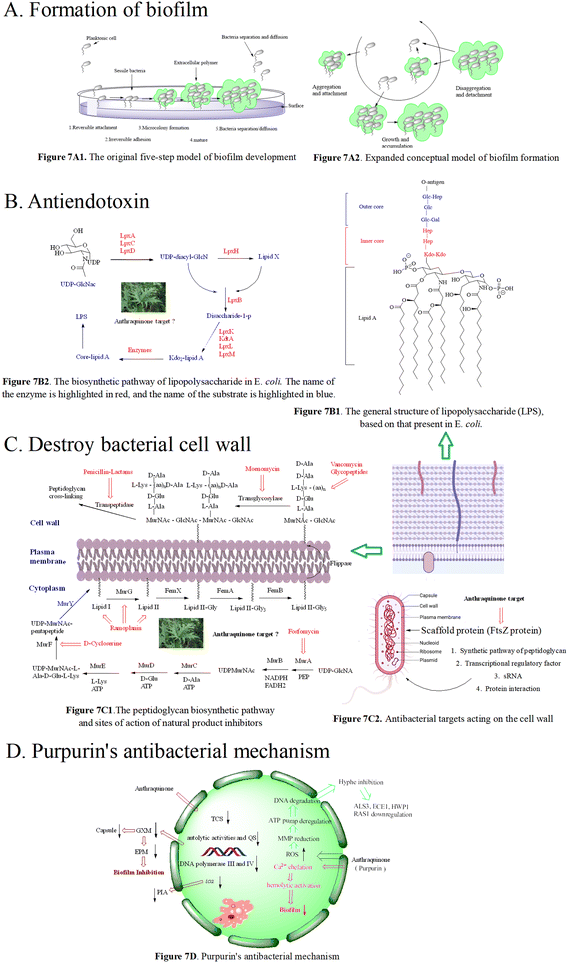 |
| Fig. 7 7A1 The original five-step model of biofilm development. 1) In the reversible attachment stage, bacteria attach to the substrate surface non-specifically; 2) in the irreversible attachment stage, bacteria interact with the substrate surface through adhesion protein or adhesion factor; 3) at the micro colony formation stage, bacteria produce extracellular polymers; 4) in the mature stage of the biofilm, bacteria synthesize and release signal molecules; 5) in the bacterial abscission/diffusion stage, bacteria leave the biofilm and return to an independent planktonic lifestyle; 7A2. Expanded conceptual model of biofilm formation; 7B1. The general structure of lipopolysaccharide (LPS), based on that present in E. coli; 7B2. The biosynthetic pathway of lipopolysaccharide in E. coli. The name of the enzyme is highlighted in red, and the name of the substrate is highlighted in blue; 7C1. The peptidoglycan biosynthetic pathway and sites of action of natural product inhibitors. MurA, known as enol acetone transferase, MurB flavin dependent reductase, MurC, MurD, MurE, and MurF, are four kinds of amino acid ligases, bacterial transposase (MraY), and MurG are transferases responsible for the synthesis of lipid II. 7C2. Antibacterial targets acting on the cell wall. 7D. Purpurin's antibacterial mechanisms. | |
We herein focus on the anti-biofilm mechanism of anthraquinones. In general, membrane-damaging agents exert the activities through a variety of ways, including the interaction of lipophilic groups and membrane proteins, or the change of the proton dynamics and the inhibition of the electron transfer chain. Anthraquinone and its derivatives as hydrophobic substances are traditionally considered to reduce the hydrophobic interaction between hydrocarbon chains in the phospholipid bilayer, weaken the fluidity of the cell membrane, enhance permeability, and then destroy the biological membrane structure. At the same time, mitochondrial depolarization generating a higher level of reactive oxygen species activates lipid peroxidation and antioxidant defense systems, and the oxidative stress further leads to a significant decrease in the amount of extracellular polymeric matrix and capsular sugars (mannose, xylose and glucuronic acid). This is possibly one of the important anti-MRSA mechanisms of anthraquinone.173–175 Biofilm formation is affected by many factors, such as the quorum sensing signal system and a variety of regulating protein genes. The biofilm formation of Staphylococcus aureus inhibited by emodin is achieved by blocking cell adhesion. Polysaccharide intercellular adhesion (PIA) is an important component of the Staphylococcus aureus biofilm. Synthesis of PIA and the expression of ica genes dominate the biofilm formation ability.176Ica is composed of icaA, icaB, icaC and icaD. IcaA and icaD are central to the PIA generation,177 and emodin can reduce the expression of ica genes. Besides, emodin stimulation leads to the reduction of DNA polymerases III and IV,178,179 and affects gene repair and bacterial resistance. Simultaneous reduction of DNA polymerase III can change bacteria from virulent forms to quiescent ones.180 The decrease in the biofilm formation179,181 may be caused by the down-regulation of two-component signal transduction systems (TCSs) affecting the autolytic activity and QS. In addition to gene regulation, the anti-biofilm activity of anthraquinone may be related to the polysaccharide of the bacterial complex capsule, ascribed to the biofilm formation of this yeast complex as a capsule-dependent event.182 Release of glucuronic xylan (GXM) from the capsule is blocked by interaction of the anthraquinone and capsule, thus affecting the adhesion of yeast cells to the surface and the formation of the extracellular polymer matrix.183 Therefore, we infer the anti-biofilm mechanism of anthraquinone shown in Fig. 7D.
3.1.2 Anti endotoxin.
The main chemical component of bacterial endotoxin, discovered at the end of the 19th century, is lipopolysaccharide (LPS). Gram negative bacteria have two different membranes, an inner membrane and an outer membrane. LPS distributed in the outer membrane, shown in Fig. 7B2, is toxic.184 As the main component of the outer membrane, LPS is crucial to survival of most Gram-negative bacteria. LPS includes three parts: lipid A, a core polysaccharide and an antigen repeating sequence. Lipid A represents the hydrophobic component of LPS located on the surface of the outer membrane, while the core polysaccharide and antigen repeating sequence reside in the surface of bacterial cells.185,186 Lipid A is believed to be responsible for the toxic effect of Gram-negative bacteria.187 The structure of LPS responsible for the virulence of bacteria varies from bacteria to bacteria.188 Accordingly, the enzymes involved in the biosynthesis and transportation of lipid A or LPS are the promising targets for developing new antibiotics. As shown in Fig. 7B1, the purification and characterization of the first three enzymes, LpxA, LpxC and LpxD residing in the lipid A biosynthesis pathway, have been accomplished,189–191 providing the structural information of these proteins for designing and developing new antibiotics,192,193e.g., to modify the structure of lipid A, to develop new LPS antagonists, or to improve the traditional Gram-negative bacteria vaccine.194–196 Unfortunately, although it has been reported that anthraquinones can inhibit bacteria growth via blocking the biofunctions of LPS, the mechanisms are poorly studied. The release of endotoxin from E. coli197 is reduced by the methanol extract of rhubarb. Moreover, the greater the volume fraction of aloe containing serum is, the less the endotoxin residue occurs, indicating that aloe has an inhibitory effect on endotoxin.198 Taken together, the antiendotoxin of anthraquinones representing the new antibacterial mechanism deserves to be explored in future research.
3.1.3 Destruction of the bacterial cell wall.
Anthraquinones can disrupt the integrity of the bacterial cell membrane and cell wall to achieve their bactericidal activity. They mainly behave in the following two aspects, on the one hand, the structural integrity of the cell wall and cell membrane is destroyed to cause intracellular material outflow, reduction of various intracellular bioactive components, and synthesis or functional impairment of nucleic acids, proteins, ATP, etc.; on the other hand, the absorption of nutrients, the excretion of metabolic wastes, the active transport, the passive transport, and the transmission of information rely completely on the cell wall and cell membrane. Although anthraquinones able to disrupt the cell wall are proposed by many papers, the specific targets and action mechanisms have not been systematically reviewed. Therefore, we herein analyze and summarize them on the basis of the previous related results.
The cell wall of Gram-negative and -positive bacteria is mainly reticulated balloons formed by peptidoglycans, i.e. high-strength reticulated scaffold structures formed by alternating N-acetylcytidylic acid and N-acetylglucosamine linked by β-1,4 glycosidic bonds.199 It mainly consists of lipopolysaccharide (LPS), peptidoglycan (PG), lipid A-associated protein (LAP), surface-associated material (SAM), phosphopeptidic acid (TA), and other active components.200 Basically, the peptidoglycan skeletons of different bacterial cell walls are identical, mainly differing in the composition of amino acids in the tetrapeptide tails and the cross-linking way. As shown in Fig. 7C1, the synthesis of peptidoglycan occurs in three stages at three different bacterial locations.201 Since intact peptidoglycan is essential for bacterial survival, all the proteins responsible for cell wall synthesis and regulation are considered important targets in the discovery of new antibacterial drugs.202 To date, there are 5 antimicrobial targets reported to participate in the synthesis of the cell wall: 1. enzymes in the synthesis pathway of peptidoglycan,203–206 such as MurA–MurG, transglycosylase, and transpeptidase; 2. scaffolding proteins,207 including FtsZ protein that mediates bacterial cells to produce Z-loop and regulate cell division, GpsB protein that regulates cell division, DivIVA protein that regulates cell division and sporulation, and EzrA protein that acts in conjunction with GpsB protein to regulate cell wall synthesis; 3. transcriptional regulatory factor, upon exposure to pressure response, bacteria use σ gene expression levels regulated by two-component systems (TCSs) and transcription regulators. The bacteria with the airSR gene to be knocked out had autolysis, and the gene could directly combine with other genes (cap, pbp1, ddl, etc.) to regulate cell wall metabolism;208 4. post transcriptional modification, SRNA regulates the cell wall, for instance, in Listeria monocytogenes,209,210 the protein Lmo0514 related to the cell wall synthesis recognizes the structure of the classified protease LPXTG, which can covalently connect itself to the cell wall; 5. protein–protein interactions affecting cell wall synthesis and hydrolysis. The dynamic flow of peptidoglycan synthesis and degradation is the main factor responsible for the morphology of bacterial cells. The proteins MreC and MreD are related to peptidoglycan synthesis, and penicillin binding proteins (PBPs) are referred to as peptidoglycan synthetases.211 PBPs synthesize cell walls as the main members of the peptidoglycan synthetase system. Penicillin targeting PBPs can inactivate their enzymatic activity, leading to the disorder of the peptidoglycan metabolic flow and thus eliciting the bacteria death.212 In addition to peptidoglycan synthetases, the hydrolase activity is crucial for the regulation of peptidoglycan growth, cell division and bacterial morphological changes.
At present, there are two types of antibiotics widely used to inhibit cell wall synthesis: (1) fosfomycin that inhibits the production of the disaccharide oligopeptide precursor in the cytoplasm;213 (2) β-lactams that have inhibitory effects on connexin PBPs to block cell wall assembly.214 However, some multidrug-resistant bacteria appear insensitive to β-lactams. In the face of antibiotics that can damage the synthesis of the bacterial cell wall, including penicillin and cephalosporin, MRSA escaped the threat of antibiotics by thickening the cell wall via enzyme mutant and hydrolase generation.215,216 MRSA also has other drug-resistant mechanisms where the decreased sensitivity of MRSA to antibiotics was achieved by the change of cell wall components.217 Emodin increased the ability to eliminate drug resistance of S. aureus in vitro and in vivo, and the antibacterial effect of emodin is the same as that of linezolid, and is superior to that of imipenem, cefepime and other antibiotics.218 Using scanning electron microscopy and transmission electron microscopy, the activity of emodin is demonstrated to be closely related to its disruption of the bacterial cell wall and cell membrane integrity.224 Treated with emodin, the cell wall and cell membrane became thick and cracked, resulting in the loss of intracellular components. According to the time growth curve, emodin exhibits a time-dependent reduction of bacteria, and the MBC/MIC values of emodin are mostly in the range of 1–2 μM,219 suggesting the antibacterial mode belonging to a bactericide function. Exposed to a long dosing of emodin, the MIC of bacteria tested fail to increase.220 Moreover, emodin has low toxicity to normal cells, presenting a good safety profile within the range of effective bactericidal concentration.221–223 In addition to direct observation means such as electron microscopy, the conductivity of the cell wall and cell membrane is another evaluation approach. When bacteria are treated with anthraquinone derivatives, the conductivity increases significantly,225,226 accompanied with the leakage of cell contents, an indication that anthraquinone derivatives can change the permeability of the cell wall. For instance, purpurin227 inhibited bacterial growth by interfering with the assembly of the Z ring in the middle of the cell, but not affecting the nucleoid separation, hinting its selectivity to FtsZ. The inhibitory effect of purpurin on mammalian cells is weaker than that on bacterial cells, emphasizing that the antibacterial target of anthraquinones may be FtsZ as shown in Fig. 7C2. In recent years, the scaffold protein FtsZ regulating cell wall division is demonstrated to be a promising target, and FtsZ inhibitors are mainly natural products, small molecular peptides and nucleic acids.
3.1.4 Inhibiting protein synthesis and nucleic acids.
Anthraquinones also exert their antibacterial activity by inhibiting corresponding proteins or nucleic acids. Aloe emodin attenuated S. aureus pathogenicity by interfering with the oligomerization of α-toxin.228 The strategy of targeting virulence factors229,230 may give us some inspiration in the design and development of antibacterial drugs. As we mentioned above, rhein can reduce the pathogenicity of Pseudomonas gingivalis by reducing the transcriptional genes encoding important virulence factors.231,232 Anthraquinones inhibited cell function by penetrating the cell membrane binding with DNA, leading to cell death.233 This was supported by Ankita's study234,235 that anthraquinones extracted from aloe could inhibit nucleic acid synthesis of Bacillus subtilis, affect DNA replication and transcription, and block the protein expression. It has also been found that236 rhein can inhibit some oxygen respiration and fermentation genes of S. aureus and genes of the ribonucleic acid reductase system, achieving its bacteriostasis. Moreover, anthraquinones can be used as an inhibitor of QS,237 preventing the agr signal transmission of the agr allele of S. aureus. Purpurin and quinalizarin can inhibit the expression of the hla gene that plays an important role in the biofilm formation.238 Additionally, various reports on the antibacterial effects of anthraquinones via protein inhibition have been published. Emodin inhibited the growth of Haematococcus parasuis by suppressing the expression of key proteins distributed in the ribosome synthesis, ABC transport system, carbohydrate metabolism pathway and bacterial cell division.239 Anthraquinones are discovered to inhibit FtsZ protein,240 and interfere with the activity of the pyruvate pathway, and inhibit ribosome proteins and the aminoacyl tRNA synthetase of MRSA.241
Purpurin inhibits biofilm-related genes (spa, psmα and rbf) and the α-hemolysin hla gene and controls the expression of the cid/lrg gene. In another study, purpurin can inhibit the growth of Gram-negative and -positive bacteria producing O-acetylated peptidoglycan and APE with IC50 values ranging from 0.3 to 23 μM.242,243 Purpurin displayed antibacterial activity against 24 strains of 6 Candida species with MICs ranging from 1.28 to 5.12 μg mL−1. Anti-bacterial mechanisms showed that purpurin induced apoptosis of Candida cells through depolarizing mitochondrial membrane potentials, one of the biochemical checkpoints controlling cell death in eukaryotic cells, and formed biofilm and mycelium by blocking an energy dependent efflux pump.244–247 The mechanisms are presented in Fig. 7D.
3.1.5 Inhibition of bacteria respiratory metabolism.
Respiratory metabolism is a main way for organisms to obtain required energy for life activity, including the tricarboxylic acid cycle, glycolysis, and pentose phosphate. The respiratory metabolic process of microorganisms is inhibited, leading to the reduced generation of energy and the carbon skeleton in the metabolic activity, and thus affecting the normal growth and reproduction of microorganisms. The microorganism growth inhibited by antibiotics was proved to be related to the suppression of cell respiration,248 while the cell death caused by most bactericidal antibiotics was associated with the acceleration of respiration. Knockout of cytochrome oxidases inhibiting cellular respiration is sufficient to attenuate bactericidal lethality, whereas acceleration of basal respiration by genetically uncoupling ATP synthesis from electron transport chains results in potentiation in the killing effect of bactericidal antibiotics. Anthraquinones reduce the respiratory control index and P/O value (the relationship of ATP synthesis and oxygen consumption) of rat liver mitochondria through the uncoupling mode and enhance the antibacterial effects.249 Also, the anthraquinones from rhubarb exhibit anti-coliform activities via inhibiting electron transfer and decoupling effects.250 Emodin has a potential inhibitory effect on a variety of human liver cancer cell lines, stimulating the expression of p53 and p21 genes to inhibit respiration and arrest the cell cycle.251,252 Besides, the key enzymes are inhibited by anthraquinones in the tricarboxylic acid cycle and cell energy metabolism of eukaryotic cells and prokaryotic cells.253,254 For example, blockage of SDH (successive dehydrogenase) and MDH (malate dehydrogenase) by anthraquinones can significantly inhibit the respiration of Staphylococcus aureus, accounting for a respiratory inhibition rate of 40%.
3.1.6 Inhibiting other substances.
Anthraquinones has other antibacterial mechanisms, including the regulation of efflux pumps, enzymes, and active oxygen species. The antibacterial activity of rhein is realized by regulating the enzyme in microorganisms,255 as the concentration increases, the N-acetyltransferase activity of Helicobacter pylori decreases, and then the nucleic acid synthesis is inhibited accordingly. Anthraquinones improve the inhibitory activity of efflux pumps, along with low activity against some multidrug-resistant bacteria.256,257 A convincing example is taken that emodin has poor antibacterial activity against Escherichia coli, but is resistant to PaβN (outflow pump inhibitor) and significantly increases the antibacterial activity of other antibiotics, indicating that the antibacterial mechanism of emodin is possibly associated with regulating the activity of outflow pumps.258 In addition, some anthraquinone derivatives generating reactive oxygen species (ROS)259–262 with photosensitization, especially singlet molecular oxygen (1O2), superoxide anions, and hydroxyl radicals, produce oxidative damage to cause physiological reactions in bacteria,263–265 thus achieving bactericidal effects.
Besides, according to recent reports, good inhibitory effects of anthraquinone-type derivatives on fungi have been observed. In terms of mechanism, it not only can induce apoptosis of fungal mitochondria by depolarizing the membrane potential, and but also can inhibit the function of efflux pumps.266 Anthraquinone derivatives restrict C. dubliniensis biofilm production in a concentration-dependent manner; this was supported by mature biofilms less susceptible to purpurin. Their MMP-independent apoptosis is triggered by the increased intracellular ROS levels in fungal biofilms and MMP depolarization, followed by DNA degradation. In the C. albicans biofilm under hypha-inducing conditions, anthraquinone derivatives block the yeast-to-hypha transition followed by the distortion of biofilm synthesis, resulting in decreased metabolic activities. Anthraquinone derivatives reduce the expression of hypha-specific genes including ALS3, HYR1, HWP1, and the hyphal regulator RAS1.267,268
3.2 Toxicity of anthraquinones
Safety is a prerequisite for a therapeutic drug. Although anthraquinone drugs are reported to have no toxic and side effects when used reasonably, the antibacterial activity of anthraquinone is related to its toxicity. The toxicity of some anthraquinone derivatives is undeniable, in spite of the unconfirmed correlation of the long-term use and cancer induction. Cumulative anthracyclines such as doxorubicin result in cardiotoxicity strongly associated with redox cycling and generation of free radicals, primarily limiting clinical applications. The toxicity and mutagenicity of hydroxyanthraquinones used as laxative agents have been demonstrated in vitro and in vivo. The main toxicity of anthraquinones comes from the ability to act as a Michael receptor interacting with some nucleophilic reagents in cells, such as NADPH, producing toxic substances to damage cells. The redox reaction generates some superoxide radical anions, but the occurrence of oxidation–reduction is influenced by environmental factors including oxygen and pH.269,270 The interaction of anthraquinones with some nucleophilic reagents may also produce thiols that interfere with the regulation of normal cells. Noticeably, anthraquinone derivatives are usually not prone to the Michael addition reaction due to the quinone positions α and β blocked by two benzene rings.271 Moreover, the role269–271 of anthraquinone as an oxidant or a reducing agent in the in vivo redox process remains to be identified. Accordingly, despite the large body of evidence on the involvement of anthracyclines in redox reactions, the exact degree of contribution to the antibacterial activity and toxicity in the clinic remains to be explored.
4. Concluding remarks and perspectives
In this review, the structure–activity relationships of anthraquinone and its derivatives are summarized in detail in Fig. 2 and 4–6 for the first time, and the antibacterial mechanisms and toxicity of anthraquinones are systematically analyzed. From analysis of structure–activity relationships, some conclusions are reached that the hydroxyl groups on the anthraquinone ring relate to a variety of pharmacological activities, including antibacterial, anti-cancer, and anti-inflammatory, and the polarity of substituents on anthraquinones obviously affects the antibacterial activity of anthraquinones, and the stronger the polarity is, the more potent the antibacterial effect is, and the existence of hydroxyl groups is not necessary in the antibacterial activity of hydrogenated anthraquinone derivatives. Anthraquinones have obvious antibacterial effects on many clinical drug resistant bacteria. However, most of the studies on the antibacterial activity of anthraquinone derivatives against microorganisms remain on in vitro evaluation, and relatively few in vivo tests of antibacterial anthraquinones are reported. Therefore, an appropriate experimental method selected and in vivo and in vitro comprehensive efficacy evaluation are vital to obtain antibacterial anthraquinone-based agents with better pharmacokinetics and more potent efficacy.272
The structure determines the function and action mechanism. A rigid planar structure is necessary for the antibacterial activity. The skeleton structure of the benzene ring in hydroxy-anthraquinones, as a core part quinone responsible for the biological activity, affects DNA biology. The replacement of the benzene ring in anthraquinone with an aliphatic ring has no significant effects on bacterial DNA biology. The presence of electron-rich substituents seems to be more conducive to the biofilm inhibition; the hypothesis was confirmed by the decreased activity due to an isopentane substituent, and a targeted elimination ability of an isopentene group to biofilms, along with no effect on the antibacterial mechanism in the presence of electron-deficient substituents. Noticeably, the antibacterial effects of anthraquinone glycosides are accomplished by mainly inhibiting corresponding enzymes or proteins, and the improved water solubility.
It is noted that the long-term and extensive abuse of any drug inevitably leads to drug resistance, thus reasonable drug use and drug combination are optimal options. There are many reasons causing drug resistance, anthraquinone drugs are no exception for resistance development. The significantly reduced susceptibility of fluoroquinolones to bacteria occurs in clinics due to the corresponding gene mutations in pathogens. Tetracycline as an anthraquinone-type drug inhibits protein synthesis by interfering with the 30S subunit of ribosomes, and its drug resistance mechanism is usually involved in the active removal from bacterial cells by outflux pumps, and no effect due to ribosome protection. The antibacterial mechanisms of anthraquinone derivatives reported vary a lot, and biofilm formation of outside bacteria is inhibited and eliminated by anthraquinone derivatives, and they also can interact with genes and proteins inside bacteria, demonstrating an advantage of multi-target antibacterial mechanisms. However, the drug resistance development of anthraquinone derivatives is reported to have a close relationship with the production of inactivated enzymes and changes in their target sites. Meanwhile, anthraquinone derivatives exert the elimination effects of other multi-drug resistance. A good example is illustrated where 87 reverses multidrug resistance by weakening the function of the ABCG2 transporter causing multidrug resistance.273
In other words, anthraquinone derivatives widely distributed in the plant kingdom exhibit significant pharmacological effects and broad market prospects. Our future research focuses might as well be shifted according to the pharmacological activities. Identification of structural similarities between natural product structures and protein sub-folding are a powerful tool for developing natural product-derived drugs.274,275 More importantly, the development of traditional Chinese medicine or traditional Chinese medicine preparations containing anthraquinones in combating drug resistant bacteria has great application prospects.
Conflicts of interest
The authors confirm that this review article has no conflicts of interest.
Acknowledgements
This work is partially financed by the National Natural Science Foundation of China (Grant No. U22A20518, 31872516, 32172913).
References
- J. Beneš, Initial antibiotic treatment of serious bacterial infections, Vnitr. Lek., 2019, 65, 204–209 CrossRef.
- P. Reddy, Empiric Antibiotic Therapy of Nosocomial Bacterial Infections, Am. J. Ther., 2016, 23, 982–994 CrossRef PubMed.
- I. Y. Jung, Antibiotic-Related Adverse Drug Reactions at a Tertiary Care Hospital in South Korea, BioMed Res. Int., 2017, 65, 430–497 Search PubMed.
- E. M. Sokolewicz and M. Rogowska, Antibiotic-Related Adverse Drug Reactions in Patients Treated on the Dermatology Ward of Medical University of Gdańsk, Antibiotics, 2021, 10, 114–118 CrossRef PubMed.
- T. K. Burki, Superbugs: An Arms Race Against Bacteria, Lancet Respir. Med., 2018, 6, 668–691 CrossRef PubMed.
- L. T. Dong and H. V. Espinoza, Emerging superbugs: The threat of Carbapenem Resistant Enterobacteriaceae, AIMS Microbiol., 2020, 6, 176–182 Search PubMed.
- S. Buder, H. Schöfer, T. Meyer, V. Bremer, P. K. Kohl, A. Skaletz-Rorowski and N. Brockmeyer, Bacterial sexually transmitted infections, J. Dtsch. Dermatol. Ges., 2019, 17, 287–315 Search PubMed.
- M. Dropa and Z. Daoud, Editorial: The global threat of carbapenem-resistant gram-negative bacteria, Front. Cell. Infect. Microbiol., 2022, 12, 268–274 Search PubMed.
- M. Usui, Y. Tamura and T. Asai, Current status and future perspective of antimicrobial-resistant bacteria and resistance genes in animal-breeding environments, J. Vet. Med. Sci., 2022, 84, 1292–1298 CrossRef PubMed.
- N. Khardori, C. Stevaux and K. Ripley, Antibiotics: From the Beginning to the Future: Part 2, Indian J. Pediatr., 2020, 87, 43–47 CrossRef PubMed.
- J. Emberger, D. Tassone, M. P. Stevens and J. D. Markley, The Current State of Antimicrobial Stewardship: Challenges, Successes, and Future Directions, Curr. Infect. Dis. Rep., 2018, 20, 31–40 CrossRef PubMed.
- A. G. Atanasov, S. B. Zotchev and V. M. Dirsch, International Natural Product Sciences Taskforce, Supuran CT. Natural products in drug discovery: advances and opportunities, Nat. Rev. Drug Discovery, 2021, 20, 200–216 CrossRef CAS PubMed.
- D. G. Brown, T. Lister and T. L. May-Dracka, New natural products as new leads for antibacterial drug discovery, Bioorg. Med. Chem. Lett., 2014, 24, 413–418 CrossRef CAS PubMed.
- J. V. Duval, Lesellier, Research advances for the extraction, analysis and uses of anthraquinones: A review, Ind. Crops Prod., 2016, 94, 812–833 CrossRef CAS.
- H. Huang, F. Wang and M. Luo,
et al., Halogenated anthraquinones from the marine-derived fungus Aspergillus sp. SCSIO F063, J. Nat. Prod., 2012, 75, 1346–1352 CrossRef CAS PubMed.
- B. Salehi and S. Albayrak,
et al., Aloe genus plants:from farm to food applications and phytopharmacotherapy, Food Funct., 2018, 19, 2843–2849 Search PubMed.
- M. Sanchez and E. I. Gonzalez, Pharmacological update properties of Aloe vera and its major active constituents, Ind. Crops Prod., 2020, 25, 132–137 Search PubMed.
- X. Dong, Y. Zeng, Y. Liu, L. You, X. Yin and J. Ni, Aloe-emodin: a review of its pharmacology, toxicity and 816 D. De souza collares maia castelo-branco et al., pharmacokinetics, Phytother. Res., 2020, 34, 270–281 CrossRef CAS PubMed.
- Y. Chen, B. Feng, Y. Yuan, J. Hu and Z. Du, Aloe emodin reduces cardiac inflammation induced by a high-fat diet through the TLR4 sig-naling pathway, Mediators Inflammation, 2020, 25, 631–635 CrossRef PubMed.
- Y. Jing, D. X. Yang, W. Wang, F. Yuan, H. Chen and H. L. Tian, Aloin protects against blood-brain barrier damage after traumatic brain injury in mice, Neurosci. Bull., 2020, 36, 625–638 CrossRef CAS PubMed.
- W. Lee and J. S. Bae, Renal pro-tective effects of aloin in a mouse model of sepsis, Food Chem. Toxicol., 2019, 132, 110–132 CrossRef PubMed.
- A. M. Donkor, D. M. Nonkor and N. Kuubabongnaa, Evaluation of anti-infective potencies of formulated
aloin A ointment and aloin A isolated from Aloe barbadensis Miller, BMC Chem., 2020, 14, 8–43 CrossRef PubMed.
- Y. Jing, D. X. Yang, W. Wang, F. Yuan, H. Chen, J. Ding, Z. Geng and H. L. Tian, Aloin protects against blood-brain barrier damage after traumatic brain injury in mice, Neurosci. Bull., 2020, 36, 625–638 CrossRef CAS PubMed.
- L. Xie, H. Tang, J. Song, J. Long and L. Zhang, Chrysophanol: a review of its pharmacology, toxicity and pharmacokinetics, J. Pharm. Pharmacol., 2019, 71, 1475–1487 CrossRef CAS PubMed.
- S. Su, J. Wu, Y. Gao, Y. Luo and P. Wang, The pharmacological properties of chrysophanol, the recent advances, Biomed. Pharmacother., 2020, 125, 110002 CrossRef CAS PubMed.
- H. Dave and L. Ledwani, A review on anthraquinones isolated from Cassia species and their applications, Ind. Crops Prod., 2012, 27, 291–319 Search PubMed.
- M. Fouillaud, M. Venkatachalam and L. Dufossé, Anthraquinones and Derivatives from Marine-Derived Fungi: Structural Diversity and Selected Biological Activities, Mar. Drugs, 2016, 14, 64–68 CrossRef PubMed.
- Y. Li and J. G. Jiang, Health functions and structure–activity relationships of natural anthrauinones from plants, Food Funct., 2018, 9, 6063–6080 RSC.
- N. H. Lee, S. M. Lee, D. H. Song, J. Y. Yang and H. S. Lee, Antimicrobial effect of emodin isolated from Cassia tora Linn. seeds against food-borne bacteria, J. Appl. Biol. Chem., 2013, 56, 187–189 CrossRef.
- J. C. Chukwujekwu, P. H. Coombes and D. A. Mulholland, Emodin, an antibacterial anthraquinone from the roots of Cassia occidentalis, S. Afr. J. Bot., 2006, 72, 295–297 CrossRef CAS.
- F. Cao, W. Peng and X. Li,
et al., Emodin is identified as the active component of ether extracts from Rhizoma Polygoni Cuspidati, for anti-MRSA activity, Can. J. Physiol. Pharmacol., 2015, 93, 1–9 CrossRef PubMed.
- J. S. Xu, Y. Cui and X. M. Liao,
et al., Effect of emodin on the cariogenic properties of Streptococcus mutans and the development of caries in rats, Exp. Ther. Med., 2014, 8, 1308–1312 CrossRef CAS PubMed.
- J. Chen and L. Zhang,
et al., Emodin targets the β-hydroxyacyl-acyl carrier protein dehydratase from Helicobacter pylori: enzymatic inhibition assay with crystal structural and thermodynamic characterization, BMC Microbiol., 2009, 9, 91 CrossRef PubMed.
- T. Hatano, H. Uebayashi, H. Ito, S. Shiota, T. Tsuchiya and T. Yoshida, Phenolic constituents of cassia seeds and antibacterial effect of some naphthalenes and anthraquinones on methicillin-resistant Staphylococcus aureus, Chem. Pharm., 1999, 47, 1121–1127 CrossRef CAS PubMed.
- S. Basu, A. Ghosh and B. Hazra, Evaluation of the antibacterial, activity of Ventilago madraspatana Gaertn., Rubia cordifolia Linn., and Lantana camara Linn: isolation of emodin and physcion as active antibacterial agents, Phytother. Res., 2005, 19, 888–894 CrossRef CAS PubMed.
- N. Khamthong, V. Rukachaisirikul, K. Tadpetch and J. Sakayaroj, Tetrahydroanthraquinone and xanthone derivatives from the marine-derived fungus Trichoderma aureoviride PSU-F95, Arch. Pharmacal Res., 2012, 35, 461–468 CrossRef CAS PubMed.
- J. C. Chukwujekwu, P. H. Coombes, D. A. Mulholland and J. Staden, Emodin, an antibacterial anthraquinone from the roots of Cassia occidentalis, S. Afr. J. Bot., 2006, 72, 295–297 CrossRef CAS.
- M. H. Chang, S. C. Chang and W. H. Chan, Injurious effects of emodin on maturation of mouse oocytes, fertilization and fetal development via apoptosis, Int. J. Mol. Sci., 2012, 13, 13911–13925 CrossRef CAS PubMed.
- Y. S. Lee, O. H. Kang and J. G. Choi, Synergistic effect of emodin in combination with ampicillin or oxacillin against methicillin-resistant Staphylococcus aureus, Pharm. Biol., 2010, 48, 1285–1290 CrossRef CAS PubMed.
- D. Dey, R. Ray and B. Hazra, Antitubercular and antibacterial activity of quinonoid natural products against multi-drug resistant clinical isolates, Phytother. Res., 2014, 28, 1014–1021 CrossRef CAS PubMed.
- T. Promgool, O. Pancharoen and S. Deachathai, Antibacterial and antioxidative compounds from Cassia alata Linn, Songklanakarin J. Sci. Technol., 2014, 36, 459–463 CAS.
- L. Li, X. Song, Z. Yin, R. Jia, Z. Li, X. Zhou, Y. Zou, L. Li, L. Yin, G. Yue, G. Ye, C. Lv, W. Shi and Y. Fu, The antibacterial activity and action mechanism of emodin from Polygonum cuspidatum against Haemophilus parasuis in vitro, Microbiol. Res., 2016, 186, 139–145 CrossRef PubMed.
- Y. B. Yang, S. Wang, C. Wang and Q. Y. Huang,
et al., Emodin affects biofilm formation and expression of virulence factors in Streptococcus suis ATCC700794, Arch. Microbiol., 2015, 197, 1173–1180 CrossRef CAS PubMed.
- X. Yan and J. Ge, The effect of emodin on Staphylococcus aureus strains in planktonic form and biofilm formation in vitro, Arch. Microbiol., 2017, 199, 1267–1275 CrossRef CAS PubMed.
- M. A. Yusuf, B. N. Singh, S. Sudheer, R. N. Kharwar and V. K. Gupta, Chrysophanol: A Natural Anthraquinone with Multifaceted Biotherapeutic Potential, Biomolecules, 2019, 9(2), 68–73 CrossRef PubMed.
- L. Xie, H. Tang, J. Song, J. Long, L. Zhang and X. Li, Chrysophanol: a review of its pharmacology, toxicity and pharmacokinetics, J. Pharm. Pharmacol., 2019, 71, 1475–1487 CrossRef CAS PubMed.
- M. M. Ghoneim,
et al., Biologically active secondary metabolites from Asphodelus microcarpus, Planta Med., 2013, 8, 1117–1130 CAS.
- D. Abdissa,
et al., Phytochemical investigation of Aloe pulcherrima roots and evaluation for its antibacterial and antiplasmodial activities, PLoS One, 2017, 12, 10–23 CrossRef PubMed.
- R. M. Coopoosamy and M. L. Magwa, Antibacterial activity of chrysophanol isolated from Aloe excelsa (Berger), Afr. J. Biotechnol., 2006, 5, 1508–1510 CAS.
- S. Singh, S. K. Singh, I. Chowdhury and R. Singh, Understanding the Mechanism of Bacterial Biofilms Resistance to Antimicrobial Agents, Open Microbiol. J., 2017, 11, 53–62 CrossRef CAS PubMed.
- C. F. Rodrigues, M. E. Rodrigues, S. Silva and M. Henriques, Candida glabrata Biofilms: How Far Have We Come, J. Fungi, 2017, 3, 11–20 CrossRef PubMed.
- E. M. Malik and C. E. Muller, Anthraquinones As Pharmacological Tools and Drugs, Med. Res. Rev., 2016, 36, 705–748 CrossRef CAS PubMed.
- C. X. Lu, H. X. Wang, W. P. Lv, P. Xu, J. Zhu, J. Xie, B. Liu and Z. X. Lou, Antibacterial properties of anthraquinones extracted from rhubarb against Aeromonas hydrophila, Fish. Sci., 2011, 77, 375–384 CrossRef CAS.
- E. E. Caamalfuentes,
et al., Anti-giardia activity and acute toxicity of a methanol extract of Senna racemosa bark, J. Ethnopharmacol., 2016, 193, 604–606 CrossRef PubMed.
- D. P. Overy, F. Berrue, H. Correa, N. Hanif, K. Hay, M. Lanteigne, K. Mquilian, S. Duffy, B. P. Oland and R. Jagannathan,
et al., Sea foam as a source of fungal inoculum for the isolation of biologically active naturalproducts, Mycology, 2014, 5, 130–144 CrossRef CAS PubMed.
- L. Guo, J. C. Guo and F. Q. Xu, Optimized extraction process and identification of antibacterial substances from Rhubarb against aquatic pathogenic Vibrio harveyi, 3 Biotech, 2017, 7, 377–394 CrossRef PubMed.
- S. K. Agarwal, S. S. Singh and S. Kumar, Antifungal activity of anthraquinone derivatives from Rheum emodi, J. Ethnopharmacol., 2000, 72, 43–46 CrossRef CAS PubMed.
- M. Malmir,
et al., In vitro anti-Neisseria gonorrhoeae activity of Senna podocarpa root extracts, Ind. Crops Prod., 2015, 76, 467–471 CrossRef CAS.
- A. H. Shi,
et al., Separation, antioxidant and antimicrobial activities of chemical constituents from exocarp of Juglans mandshurica Maxim, Asian J. Chem., 2013, 25, 3361–3365 CrossRef CAS.
- F. I. Andrade,
et al., Chemical constituents and an alternative medicinal veterinary herbal soap made from Senna macranthera, J. Evidence-Based Complementary Altern. Med., 2015, 15(5), 1–6 Search PubMed.
- H. Xiang, F. Cao, D. Ming, Y. Y. Zhang, X. Y. Dong and X. B. Zhong, Aloe-emodin inhibits Staphylococcus aureus biofilms and extracellular protein production at the initial adhesion stage of biofilm development, Appl. Microbiol. Biotechnol., 2017, 101, 6671–6681 CrossRef CAS PubMed.
- T. Li, Y. Lu, H. Zhang, L. Wang and X. Hou, Antibacterial Activity and Membrane-Targeting Mechanism of Aloe-Emodin Against Staphylococcus epidermidis, Front. Microbiol., 2021, 16, 6218–6266 Search PubMed.
- H. H. Wang, J. G. Chung, C. C. Ho and L. T. Wu, Aloe-emodin effects on arylamine N-acetyltransferase activity in the bacterium Helicobacter pylori, Planta Med., 1998, 64, 176–178 CrossRef CAS PubMed.
- J. Xi and Q. Wu,
et al., Aloe-emodin/Carbon nanoparticle hybrid gels with light-induced and long-term antibacterial activity, ACS Biomater. Sci. Eng., 2018, 4, 4391–4400 CrossRef CAS PubMed.
- X. Xiong and X. Li,
et al., Antibacterial and Alkali-responsive Cationic Waterborne Polyurethane Based on Modification of Aloe Emodin, Chem. Res. Chin. Univ., 2022, 4, 1–10 Search PubMed.
- T. Li and Y. Lu,
et al., Antibacterial activity and membrane-targeting mechanism of aloe-emodin against Staphylococcus epidermidis, Front. Microbiol., 2021, 12, 6218–6266 Search PubMed.
- Y. X. Zhou, W. Xia, W. Yue and C. Peng, Rhein: A Review of Pharmacological Activities, J. Evidence-Based Complementary Altern. Med., 2015, 15, 5781–5797 Search PubMed.
- G. Chung, M. F. Tsou and H. H. Wangetal, Rheinaffects arylamine N-acetyltransferase activity in Helicobacter pylori from peptic ulcer patients, J. Appl. Toxicol., 1998, 18, 117–123 CrossRef PubMed.
- L. Yu, H. Xiang and J. Fan,
et al., Global transcriptional response of Staphylococcus aureus to rhein, a natural plant product, J. Biotechnol., 2008, 135, 304–308 CrossRef CAS PubMed.
- X. Liu, J. Cheng, X. Zheng and H. Zhou, Targeting CpG DNA to screen and isolate anti-sepsis fraction and monomers from traditional Chinese herbs using affinity biosensor technology, Int. Immunopharmacol., 2009, 9, 1021–1031 CrossRef CAS PubMed.
- E. Serretiello, V. Folliero, B. Santella and G. Boccia, Trend of Bacterial Uropathogens and Their Susceptibility Pattern: Study of Single Academic High-Volume Center in Italy (2015–2019), Int. J. Microbiol., 2021, 5, 170–186 Search PubMed.
- A. Priya, C. B. M. Kumar, A. Valliammai, A. Selvaraj and S. K. Pandian, Usnic acid deteriorates acidogenicity, acidurance and glucose metabolism of Streptococcus mutans through downregulation of two-component signal transduction systems, Sci. Rep., 2021, 11, 1374–1383 CrossRef CAS PubMed.
- W. H. Bowen, R. A. Burne, H. Wu and H. Koo, Oral biofilms: pathogens, matrix, and polymicrobial interactions in microenvironments, Trends Microbiol., 2018, 26, 229–242 CrossRef CAS PubMed.
- J. Zhang, J. Liu, J. Ling, Z. Tong, Y. Fu and M. Liang, Inactivation of glutamate racemase (MurI) eliminates virulence in Streptococcus mutans, Microbiol. Res., 2016, 18, 1–8 CrossRef CAS PubMed.
- D. Kim and K. Kim, Combinatorial treatment of sophoraflavanone G and rhein with ampicillin, oxacillin, or oxytetracycline synergistically increased antibacterial activity against oral bacteria, Rev. Med. Microbiol., 2021, 32, 211–218 CrossRef.
- A. T. Nguyen and K. Y. Kim, Rhein inhibits the growth of Propionibacterium acnes by blocking NADH dehydrogenase-2 activity, J. Med. Microbiol., 2020, 69, 689–696 CrossRef CAS PubMed.
- V. Folliero, G. Franci and M. Galdiero, Rhein: A novel antibacterial compound against Streptococcus mutans infection, Microbiol. Res., 2022, 82, 127–162 Search PubMed.
- Y. Hou,
et al., Study on the active components of rhubarb inhibiting foodborne pathogens, Shipin Gongye Keji, 2015, 36, 4–17 Search PubMed.
- J. Azelmat, J. F. Larente and D. Grenier, The anthraquinone rhein exhibits synergistic antibacterial activity in association with metronidazole or natural compounds and attenuates virulence gene expression in Porphyromonas gingivalis, Arch. Oral Biol., 2015, 60, 342–346 CrossRef CAS PubMed.
- S. M. Arwish and M. Ateyyat, The Pharmacological and Pesticidal Actions of Naturally Occurring 1, 8-dihydroxyanthraquinones Derivatives, Helicobacter, 2008, 4, 495–505 Search PubMed.
- L. Chunxia, W. Hongxin and X. Jun, Antibacterial properties of anthraquinones extracted from rhubarb against Aeromonas hydrophila, Fish. Sci., 2011, 77, 375–384 CrossRef.
- J. Wang and H. Zhao, Microcalorimetric assay on the antimicrobial property of five hydroxyanthraquinone derivatives in rhubarb to Bifidobacterium adoles-centis, Phytomedicine, 2010, 17, 684–689 CrossRef CAS PubMed.
- J. Y. Yao, L. Y. Lin and X. M. Yuan, Antifungal Activity of Rhein and Aloe-Emodin from Rheum palmatum on Fish Pathogenic Saprolegniasp, J. World Aquacult. Soc., 2017, 48, 137–144 CrossRef CAS.
- L. K. Omosa, J. O. Midiwo, A. T. M. Baveng, S. B. Tankeo, J. A. Seukep, I. K. Voukeng, J. K. Dzotam, J. Isemeki, S. Derese, R. A. Omolle, T. Efferth and V. Kuete, Antibacterial activities and structure–activity relationships of a panel of 48 compounds from Kenyan plants against multidrug resistant phenotypes, SpringerPlus, 2016, 5, 901–930 CrossRef PubMed.
- W. Xiang, Q. Song and H. Zhang, Antimicrobial anthraquinones from Morinda angustifolia, Fitoterapia, 2008, 79, 501–504 CrossRef CAS PubMed.
- J. Lee, Y. Kim, S. Y. Ryu and J. Lee, Calcium-chelating alizarin and other anthraquinones inhibit biofilm formation and the hemolytic activity of Staphylococcus aureus, Sci. Rep., 2016, 14, 162–197 Search PubMed.
- Y. G. Lee, S. Y. Kim and J. Ryu, Calcium-chelat-ing alizarin and other anthraquinones inhibit biofilm formation and the hemolytic activity of Staphylococcus aureus, Sci. Rep., 2016, 6, 1–11 CrossRef PubMed.
- G. A. Kemegne, P. Mkounga, S. L. S. Kamdem and A. E. Nkengfack, Antimicrobial structure activity relationship of five anthraquinones of emodine type isolated from Vismia laurentii, BMC Microbiol., 2017, 5, 17–40 CrossRef PubMed.
- H. K. Hall, K. L. Karem and J. W. Foster, Molecular responses of microbes to environmental pH stress, Adv. Microb. Physiol., 1995, 37, 229–264 CrossRef CAS PubMed.
- N. Beales, Adaptation of microorganisms to cold temperatures, weak acid preservatives, low pH, and osmotic stress: A Review, Compr. Rev. Food Sci. Food Saf., 2004, 3, 1–20 CrossRef CAS PubMed.
- N. T. Manojlovic and S. S. Solujic, Anthraquinones from the lichen Xanthoria parietina, J. Serb. Chem. Soc., 1998, 63, 7–11 CAS.
- G. A. Kemegne, P. Mkounga and A. E. Nkengfack, Antimicrobial structure activity relationship of five anthraquinones of emodine type isolated from Vismia laurentii, BMC Microbiol., 2017, 17, 41–53 CrossRef PubMed.
- S. Basu, A. Ghosh and B. Hazra, Evaluation of the antibacterial activity of Ventilago madraspatana Gaertn. Rubia cordifolia Linn. and Lantana camara Linn.: isolation of emodin and physcion as active antibacterial agents, Phytother. Res., 2005, 19, 88–94 CrossRef PubMed.
- F. Duan, G. Xin, H. Niu and W. Huang, Chlorinated emodin as a natural antibacterial agent against drug-resistant bacteria through dual influence on bacterial cell membranes and DNA, Sci. Rep., 2017, 7, 121–127 CrossRef PubMed.
- C. Ji, G. Xin, F. Duan and W. Huang, Study on the antibacterial activities of emodin derivatives against clinical drug-resistant bacterial strains and their interaction with proteins, Ann. Transl. Med., 2020, 8, 92 CrossRef CAS PubMed.
- J. L. Liang, H. C. Cha, Y. Kwon and Y. Jahng, A facile synthesis of emodin derivatives, emodin carbaldehyde, citreorosein, and their 10-deoxygenated derivatives and their inhibitory activities on μ-calpain, Arch. Pharmacal Res., 2012, 35, 447–454 CrossRef CAS PubMed.
- F. M. Riedman, A. Xu, R. Lee, D. N. Nguyen and T. A. Phan, The Inhibitory Activity of Anthraquinones against Pathogenic Protozoa, Bacteria, and Fungi and the Relationship to Structure, Molecules, 2020, 25, 3101–3120 CrossRef PubMed.
- A. F. Inoue, G. A. Purgato and S. R. Pais, Chemical Constituents and an Alternative Medicinal Veterinary Herbal Soap Made from Senna macranthera, J. Evidence-Based Complementary Altern. Med., 2015, 15, 217–238 CrossRef PubMed.
- J. Liu, F. Wu and C. Chen, Design and synthesis of aloe-emodin derivatives as potent anti-tyrosinase, antibacterial and anti-inflammatory agents, Bioorg. Med. Chem. Lett., 2015, 25, 5142–5146 CrossRef CAS PubMed.
- X. Y. Liang, N. Battinim and Y. F. Sui, Aloe-emodin derived azoles as a new structural type of potential antibacterial agents: design, synthesis, and evaluation of the action on membrane, DNA, and MRSA DNA isomerase, RSC Med. Chem., 2021, 12, 602–608 RSC.
- J. Yang, S. Zhao, J. An, L. Deng and T. Lei, Synthesis and bioactivity of nover ester derivatives of rhein, Chin. Pharm. J., 2019, 54, 1216–1220 Search PubMed.
- X. Zhu, S. Chen, Y. Zheng, Y. Zhang and T. Hsiang, Antifungal and insecticidal activities of rhein derivatives: synthesis, characterization and preliminary structure-activity relationship studies, Nat. Prod. Res., 2022, 36, 4140–4146 CrossRef CAS PubMed.
- Z. Deng and R. R. Y. Bheemanaboina, Aloe emodin-conjugated sulfonyl hydrazones as novel type of antibacterial modulators against S. aureus 25923 through multifaceted synergistic effects, Bioorg. Chem., 2022, 12, 106–135 Search PubMed.
- M. Mohamadzadeh, M. Zarei and M. Vessal, Synthesis, in vitro biological evaluation and in silico molecular docking studies of novel β-lactam-anthraquinone hybrids, Bioorg. Chem., 2020, 95, 103–115 CrossRef PubMed.
- J. T. Fan, B. Kuang, G. Z. Zeng, S. M. Zhao and N. H. Tan, Biologically active arborinane-type triterpenoids and anthraquinones from Rubia yunnanensis, J. Nat. Prod., 2011, 74, 2069–2080 CrossRef CAS PubMed.
- L. R. Comini, S. C. Montoya, P. L. Páez, G. A. Argüello, I. Albesa and J. L. Cabrera, Antibacterial activity of anthraquinone derivatives from Heterophyllaea pustulata (Rubiaceae), J. Photochem. Photobiol., B, 2011, 102, 108–114 CrossRef CAS PubMed.
- M. Shan, S. Yu, H. Yan, P. Chen, Z. Lang and A. Ding, A Review of the Botany, Phytochemistry, Pharmacology and Toxicology of Rubiae Radix et Rhizoma, Molecules, 2016, 21, 1747–1756 CrossRef PubMed.
- J. Singh, Y. Hussain and S. Luqman, Purpurin: A natural anthraquinone with multifaceted pharmacological activities, Phytother. Res., 2020, 12, 107–114 Search PubMed.
- M. N. Watroly, S. Mekar and S. Fuloria, Chemistry, Biosynthesis, Physicochemical and Biological Properties of Rubiadin: A Promising Natural Anthraquinone for New Drug Discovery and Development, Drug Des., Dev. Ther., 2021, 15, 4527–4549 CrossRef CAS PubMed.
- A. Ali, N. Ismail and M. Mackeen, Antiviral, cyototoxic and antimicrobial activities of anthraquinones isolated from the roots of morinda elliptica, Pharm. Biol., 2000, 38, 298–301 CrossRef CAS PubMed.
- H. Zhou, S. J. Ma and Z. Z. Han, QR code labeling system for Xueteng-related herbs based on DNA barcode, Chin. Herb. Med., 2019, 11, 52–59 CrossRef.
- J. M. Pfeffer and A. J. Clarke, Identification of the first known inhibitors of O-acetylpeptidoglycan esterase: a potential new antibacterial target, ChemBioChem, 2012, 13, 722–731 CrossRef CAS PubMed.
- K. Xu, P. Wang, L. Wang and H. Lei, Quinone derivatives from the genus Rubia and their bioactivities, Chem. Biodiversity, 2014, 11, 341–363 CrossRef CAS PubMed.
- K. Tanaka, T. Miura, U. Nezawa, Y. Urano and T. Nagano, Rational design of fluorescein-based fluorescence probes. Mechanism-based design of a maximum fluorescence probe for singlet oxygen, J. Am. Chem. Soc., 2001, 123, 2530–2536 CrossRef CAS PubMed.
- B. Song, G. Wang and M. Tan, A europium(III) complex as an efficient singlet oxygen luminescence probe, J. Am. Chem. Soc., 2006, 128, 13442–13450 CrossRef CAS PubMed.
- X. Ragàs, X. Batllori and S. Nonell, Singlet oxygen photosensitisation by the fluorescent probe Singlet Oxygen Sensor Green, Chem. Commun., 2009, 2920–2922 RSC.
- H. Tang and H. Zhao,
et al., Studies on antitumor and antibacterial activities of 10-substituted 1-azabenzoanthrone derivatives, Huaxi Yaoxue Zazhi, 2014, 29, 3–12 Search PubMed.
- M. Cecile, S. Denis and M. O. Jean,
et al., New Xanthones from Calophyllum caledonicum, J. Nat. Prod., 2000, 63, 1471–1474 CrossRef PubMed.
- J. Ngoupayo and T. K. Tabopda,
et al., Antimicrobial and immuno-modulatory properties of prenylated xanthones from twigs of Garcinia staudtii, Bioorg. Med. Chem., 2009, 17, 5688–5695 CrossRef CAS PubMed.
- K. Klesiewicz, E. Karczewska, A. Budak, H. Marona and N. Szkaradek, Anti-Helicobacter pylori activity of some newly synthesized derivatives of xanthone, J. Antibiot., 2016, 69, 825–834 CrossRef CAS PubMed.
- A. Wisetsai, R. Lekphrom and F. T. Schevenels, New anthracene and anthraquinone metabolites from Prismatomeris filamentosa and their antibacterial activities, Nat. Prod. Res., 2021, 35, 1582–1589 CrossRef CAS PubMed.
- K. L. Yang, M. Y. Wei and C. L. Shao, Antibacterial anthraquinone derivatives from a sea anemone-derived fungus Nigrospora sp, J. Nat. Prod., 2012, 75, 935–941 CrossRef CAS PubMed.
- X. Liu, J. Shen and K. Zhu, Antibacterial activities of plant-derived xanthones, RSC Med. Chem., 2022, 13, 107–116 RSC.
- H. R. Dharmaratne, Y. Sakagami and K. G. Piyasena, Antibacterial activity of xanthones from Garcinia mangostana (L.) and their structure-activity relationship studies, Nat. Prod. Res., 2013, 27, 938–941 CrossRef CAS PubMed.
- J. J. Koh, S. Lin, Y. Bai, W. W. L. Sin, T. T. T. Aung, J. Li, V. Chandra, K. Pervushin, R. W. Beuerman and S. Liu, Antimicrobial activity profiles of Amphiphilic Xanthone derivatives are a function of their molecular Oligomerization, Biochim. Biophys. Acta, Biomembr., 2018, 1860, 2281–2298 CrossRef CAS PubMed.
- Y. Y. Yang and W. Zuo, Chemical constituents and antibacterial activity of bougainvillea bougainvillea branches and leaves, Zhongguo Yaowu Huaxue Zazhi, 2013, 23, 305–308 CAS.
- J. Cardoso, J. Freitas, F. Durães, D. T. Carvalho, L. Gales, M. Pinto, E. Sousa and E. Pinto, Antifungal Activity of a Library of Aminothioxanthones, Antibiotics, 2022, 11, 1488–1530 CrossRef CAS PubMed.
- L. J. Bessa, A. Palmeira, A. S. Gomes, V. Vasconcelos, E. Sousa, M. Pinto and P. M. Costa, Synergistic Effects Between Thioxanthones and Oxacillin Against Methicillin-Resistant Staphylococcus aureus, Microb. Drug Resist., 2015, 21, 404–415 CrossRef CAS PubMed.
- F. Durães, A. Palmeira, B. Cruz, J. Freitas-Silva, N. Szemerédi, L. Gales, P. M. Costa, F. Remião, R. Silva, M. Pinto, G. Spengler and E. Sousa, Antimicrobial Activity of a Library of Thioxanthones and Their Potential as Efflux Pump Inhibitors, Pharmaceuticals, 2021, 14, 572–579 CrossRef PubMed.
- K. Y. Chan, J. Zhang and C. W. T. Chang, Mode of action investigation for the antibacterial cationic anthraquinone analogs, Bioorg. Med. Chem. Lett., 2011, 21, 6353–6356 CrossRef CAS PubMed.
- Y. P. Subedi and C. T. Chang, Cationic Anthraquinone Analogs as Selective Antimicrobials, Microbiol. Insights, 2019, 12, 1–4 Search PubMed.
- M. Y. Fosso, K. Y. Chan, R. Gregory and C. W. Chang, Library synthesis and antibacterial investigation of cationic anthraquinone analogs, ACS Comb. Sci., 2012, 14, 231–235 CrossRef CAS PubMed.
- L. Gao, Y. Meng, X. Zhao and X. Liu, Inhibitory effect of photoactivated hypericin on growth of human prostate cancer cell PC3M, Jilin Daxue Xuebao, Yixueban, 2004, 30, 97–99 CAS.
- S. Noell, D. Mayer, W. S. L. Strauss, M. S. Tatagiba and R. Rainer, Selective enrichment of hypericin in malignant glioma: pioneering in vivo results, Int. J. Oncol., 2011, 38, 1343–1348 CAS.
- H. Gao, J. Yang, X. Wang, Y. Song, X. Cheng, F. Wei, Y. Wang, D. Gu, H. Sun and S. Ma, Exploratory Quality Control Study for Polygonum multiflorum Thunb. Using Dinuclear Anthraquinones with Potential Hepatotoxicity, Molecules, 2022, 27, 6760–6788 CrossRef CAS PubMed.
- L. P. Mai, F. Gueritte, V. Dumontet, M. V. Tri, B. Hill, O. Thoison, D. Guenard and T. Sevenet, Cytotoxicity of Rhamnosylanthraquinones and Rhamnosylanthrones from Rhamnus nepalensis, J. Nat. Prod., 2001, 64, 1162–1168 CrossRef CAS PubMed.
- W. Wang, Y. Liao, X. Huang, C. Tang and P. Cai, A novel xanthone dimer derivative with antibacterial activity isolated from the bark of Garcinia mangostana, Nat. Prod. Res., 2018, 32, 1769–1774 CrossRef CAS PubMed.
- J. Li, X. Jiang, X. Liu, C. He, Y. Di, S. Lu, H. Huang, B. Lin, D. Wang and B. Fan, Antibacterial anthraquinone dimers from marine derived fungus Aspergillus sp, Fitoterapia, 2019, 133, 1–4 CrossRef CAS PubMed.
- G. A. Kemegne, P. Mkounga, J. J. E. Ngang, S. L. S. Kamdem and A. E. Nkengfack, Antimicrobial structure activity relationship of five anthraquinones of emodine type isolated from Vismia laurentii, BMC Microbiol., 2017, 17, 41–63 CrossRef PubMed.
- M. Friedman, A. Xu, R. Lee, D. N. Nguyen, T. A. Phan, S. M. Hamada, R. Panchel, C. C. Tam, J. H. Kim, L. W. Cheng and K. M. Land, The Inhibitory Activity of Anthraquinones against Pathogenic Protozoa, Bacteria, and Fungi and the Relationship to Structure, Molecules, 2020, 25, 3101–3130 CrossRef CAS PubMed.
- Q. Huang, Y. Wang, H. Wu, M. Yuan, C. Zheng and H. Xu, Xanthone Glucosides: Isolation, Bioactivity and Synthesis, Molecules, 2021, 26, 5575–5593 CrossRef CAS PubMed.
- G. Asamenew, D. Bisrat, A. Mazumder and K. Asres, In vitro antimicrobial and antioxidant activities of anthrone and chromone from the latex of Aloe harlana Reynolds, Phytother. Res., 2011, 25, 1756–1760 CrossRef CAS PubMed.
- A. Golcu, D. Gitmisoglu, M. Dolaz and S. Serin, Isolation of colour components from Rubia tinctorum L: chromatographic determination, spectrophotometric investigation, dyeing properties and antimicrobial activity, Asian J. Chem., 2009, 5, 321–336 Search PubMed.
-
J. Lawless, Aloe Vera: Natural wonder cure, Harper-Collins, London, England, 2014 Search PubMed.
- R. S. N. Brilhante, G. D. S. Araújo, X. M. Q. C. Fonseca, G. M. D. M. Guedes, L. D. Aguiar, D. D. S. C. M. Castelo-Branco, R. D. A. Cordeiro, J. J. C. Sidrim, W. A. P. Neto and M. F. G. Rocha, Antifungal effect of anthraquinones against Cryptococcus neoformans: Detection of synergism with amphotericin B, Med. Mycol., 2020, 59, 564–570 CrossRef PubMed.
- H. D. Smolarz, M. Swatko-Ossor, G. Ginalska and E. Medyńska, Antimycobacterial effect of extract and its components from Rheum rhaponticum, J. AOAC Int., 2013, 96, 155–160 CrossRef CAS PubMed.
- A. Choudhary, A. Choudhary, K. T. Chandrashekar, R. Mishra, V. D. Tripathi, V. Hazari and A. Trivedi, Effect of aloin (Aloe vera extract) on the levels of Porphyromonas gingivalis and Aggregatibacter actinomycetemcomitans in chronic generalized periodontitis: A clinical & microbiological study, Int. J. Adv. Res., 2019, 7, 693–701 CrossRef CAS PubMed.
- G. Asamenew, D. Bisrat, A. Mazumder and K. Asres, In vitro antimicrobial and antioxidant activities of anthrone and chromone from the latex of aloe harlana Reynolds, Phytother. Res., 2011, 25, 1756–1760 CrossRef CAS PubMed.
- M. Li and Z. Liu, In vitro effect of Chinese herb extracts on caries-related bacteria and glucan, J. Vet. Dent., 2008, 25, 236–239 CrossRef CAS PubMed.
- A. M. Shenkutie, M. Z. Yao, G. K. H. Siu, B. K. C. Wong and P. H. M. Leung, Biofilm-Induced Antibiotic Resistance in Clinical Acinetobacter baumannii Isolates, Antibiotics, 2020, 9, 817–830 CrossRef CAS PubMed.
- M. Klausen, M. Klausen, A. Heydorn, P. Ragas, L. Lambertsen, A. Aaes-Jørgensen, S. Molin and T. Tolker-Nielsen, Biofilm formation by Pseudomonas aeruginosa wild type, flagella and type IV pili mutants, Mol. Microbiol., 2003, 48, 1511–1524 CrossRef CAS PubMed.
- M. Gjermansen, M. Nilsson, L. Yang and T. Tolker-Nielsen, Characterization of starvation-induced dispersion in Pseudomonas putidabiofilms: genetic elements and molecular mechanisms, Mol. Microbiol., 2010, 75, 815–826 CrossRef CAS PubMed.
- M. Gjermansen, P. Ragas, C. Sternberg, S. Molin and T. Tolker-Nielsen, Characterization of starvation-induced dispersion in Pseudomonas putida biofilms, Environ. Microbiol., 2005, 7, 894–906 CrossRef CAS PubMed.
- M. Nilsson, W. C. Chiang, M. Fazli, M. Gjermansen, M. Givskov and T. Tolker-Nielsen, Influence of putative exopolysaccharide genes on Pseu-domonas putida KT2440 biofilm stability, Environ. Microbiol., 2011, 13, 1357–1369 CrossRef CAS PubMed.
- K. D. Jackson, M. Starkey, S. Kremer, M. R. Parsek and D. J. Wozniak, Identification of psl, a locus encoding a potential exopolysaccharide that is essential for Pseudomonas aeruginosa PAO1 biofilm formation, J. Bacteriol., 2004, 186, 4466–4475 CrossRef CAS PubMed.
- M. Matsukawa and E. P. Greenberg, Putative exopolysaccharide synthesis genes influence Pseudomonas aeruginosa biofilm development, J. Bacteriol., 2004, 186, 4449–4456 CrossRef CAS PubMed.
- D. J. Wozniak, Alginate is not a significant component of the extra-cellular polysaccharide matrix of PA14 and PAO1 Pseudomonas aeruginosa biofilms, Proc. Natl. Acad. Sci. U. S. A., 2003, 100, 7907–7912 CrossRef CAS PubMed.
- J. Liu, H. Lu, L. Wu, P. G. Kerr and Y. Wu, Interactions between periphytic biofilms and dissolved organic matter at soil-water interface and the consequent effects on soil phosphorus fraction changes, Sci. Total Environ., 2021, 801, 149–173 Search PubMed.
- B. Wu, E. F. Haney, N. Akhoundsadegh, D. Pletzer, M. J. Trimble, A. E. Adriaans, P. H. Nibbering and R. E. W. Hancock,
et al., Human organoid biofilm model for assessing antibiofilm activity of novel agents, npj Biofilms Microbiomes, 2021, 7, 8–16 CrossRef CAS PubMed.
- Y. Zhao, H. Liu, R. Wang and C. Wu, Interactions between dicyandiamide and periphytic biofilms in paddy soils and subsequent effects on nitrogen cycling, Sci. Total Environ., 2020, 718, 137–147 CrossRef PubMed.
- K. Sauer, P. Stoodley, D. M. Goeres, L. Hall-Stoodley, M. Burmølle, P. S. Stewart and T. Bjarnsholt, The biofilm life cycle: expanding the conceptual model of biofilm formation, Nat. Rev. Microbiol., 2022, 20, 608–620 CrossRef CAS PubMed.
- U. Hofer, How to build a biofilm, Nat. Rev. Microbiol., 2020, 18, 476–477 CrossRef CAS PubMed.
- S. Zhang, Research progress in the formation of bacterial biofilm and the antibiofilm activity of antimicrobial peptides, Dongwu Yingyang Xuebao, 2021, 5, 8–16 Search PubMed.
- D. A. Somma, A. Moretta, C. Canè, A. Cirillo and A. Duilio, Antimicrobial and antibiofilm peptides, Biomolecules, 2020, 10, 652–660 CrossRef PubMed.
- C. Ferriol-González and P. Domingo-Calap, Phages for biofilm removal, Antibiotics, 2020, 9, 268–273 CrossRef PubMed.
- S. Haque, F. Ahmad, S.
A. Dar, A. Jawed, R. K. Mandal, M. Wahid, M. Lohani, S. Khan, V. Singh and N. Akhter, Developments in strategies for Quorum Sensing virulence factor inhibition to combat bacterial drug resistance, Microb. Pathog., 2018, 121, 293–302 CrossRef CAS PubMed.
- F. Shatila, İ. Yaşa and H. T. Yalçın, Inhibition of Salmo-nella enteritidis biofilms by Salmonella invasion protein-tar-geting aptamer, Biotechnol. Lett., 2020, 7, 12–16 Search PubMed.
- S. Fulaz, S. Vitale, L. Quinn and E. Casey, Nanoparticle–biofilm interactions: The role of the EPS matrix, Trends Microbiol., 2019, 27, 915–926 CrossRef CAS PubMed.
- H. Narenji, O. Teymournejad, M. A. Rezaee, S. Taghizadeh, B. Mehramuz, M. Aghazadeh, M. Asgharzadeh, M. Madhi, P. Gholizadeh, K. Ganbarov, M. Yousefi, A. Pakravan, T. Dal, R. Ahmadi and H. S. Kafil, Antisense peptide nucleic acids againstftsZ andefaA genes inhibit growth and bio-film formation of Enterococcus faecalis, Microb. Pathog., 2020, 139, 103–107 CrossRef PubMed.
- L. R. Martinez and A. Casadevall, Susceptibility of Cryptococcus neoformans biofilms to antifungal agents invitro, Antimicrob. Agents Chemother., 2006, 50, 1021–1033 CrossRef CAS PubMed.
- D. Li, F. Cao, D. Ming, Y. Zheng, X. Dong, X. Zhong, D. Mu, B. Li, L. Zhong, J. Cao, L. Wang, H. Ma, T. Wang and D. Wang, Aloe-emodin inhibits Staphylococcus aureus biofilms and extracellular protein production at the initial adhesion stage of biofilm development, Appl. Microbiol. Biotechnol., 2017, 101, 6671–6681 CrossRef PubMed.
- A. Mourad and J. R. Perfect, The war on cryptococcosis: a review of the antifungal arsenal, Mem. Inst. Oswaldo Cruz, 2018, 113, 170–191 Search PubMed.
- M. Liu, W. Peng, R. Qin, Z. Yan, Y. Cen, X. Zheng, X. Pan, W. Jiang, B. Li, X. Li and H. Zhou, The direct anti-MRSA effect of emodin via damaging cell membrane, Appl. Microbiol. Biotechnol., 2015, 99, 7699–7709 CrossRef CAS PubMed.
- P. Kumari, N. Arora, A. Chatrath, R. Gangwar, V. Pruthi, K. M. Poluri and R. Prasad, Delineating the Biofilm Inhibition Mechanisms of Phenolic and Aldehydic Terpenes against Cryptococcus neoformans, ACS Omega, 2019, 4, 17634–17648 CrossRef CAS PubMed.
- E. Camacho and A. Casadevall, Cryptococcal Traits Mediating Adherence to Biotic and Abiotic Surfaces, J. Fungi, 2018, 4, 88 CrossRef CAS PubMed.
- J. Haaber, M. T. Cohn, D. Frees, T. J. Andersen and H. Ingmer, Planktonic aggregates of Staphylococcus aureus protect against common antibiotics, PLoS One, 2012, 7, 41075 CrossRef PubMed.
- K. Schilcher and A. R. Horswill, Staphylococcal biofilm development: structure, regulation, and treatment strategies, Microbiol. Mol. Biol. Rev., 2020, 84, 119–126 CrossRef PubMed.
- S. Đukanović, T. Ganić I, B. Lončarević, S. Cvetković, B. Nikolić, D. Tenji, D. Randjelović and D. Mitić-Ćulafić, Elucidating the antibiofilm activity of Frangula emodin against Staphylococcus aureus biofilms, J. Appl. Microbiol., 2022, 132, 1840–1855 CrossRef PubMed.
- W. Ding, Y. Li, H. Lian, X. Ai, Y. Zhao, Y. Yang, Q. Han, X. Liu, X. Chen and Z. He, Sub-Minimum Inhibitory Concentrations of Rhubarb Water Extracts Inhibit Streptococcus suis Biofilm Formation, Front. Pharmacol., 2017, 7, 425–433 CrossRef PubMed.
- L. Tuchscherr, E. Medina, M. Hussain, W. Völker, V. Heitmann, S. Niemann, D. Holzinger, J. Roth, R. A. Proctor, K. Becker, G. Peters and B. Löffler, Staphylococcus aureus phenotype switching: an effective bacterial strategy to escape host immune response and establish a chronic infection, EMBO Mol. Med., 2011, 3, 129–141 CrossRef CAS PubMed.
- Y. Yang, S. Wang, C. Wang, Q. Huang, J. Bai, J. Chen, X. Chen and Y. Li, Emodin affects biofilm formation and expression of virulence factors in Streptococcus suis ATCC700794, Arch. Microbiol., 2015, 197, 1173–1180 CrossRef CAS PubMed.
- E. Camacho and A. Casadevall, Cryptococcal traits mediating adherence to biotic and abiotic surfaces, J. Fungi, 2018, 4, 88–99 CrossRef CAS PubMed.
- D. D. S. C. M. Castelo-Branco, G. D. S. Araújo, X. M. Q. C. Fonseca, G. M. D. M. Guedes, M. Gl, D. Rocha, R. S. N. Brilhante, R. D. A. Cordeiro, J. J. C. Sidrim, W. A. Pereira-Neto and M. F. G. Rocha, Anthraquinones from Aloe spp. Inhibit Cryptococcus neoformans sensu stricto: effects against growing and mature biofilms, Biofouling, 2021, 37, 809–817 CrossRef PubMed.
- B. Beutler and E. T. Rietschel, Innate immune sensing and its roots: the story of endotoxin, Nat. Rev. Immunol., 2003, 3, 169–176 CrossRef CAS PubMed.
- C. R. A. Raetz, C. M. Reynolds, M. S. Trent and R. E. Bishop, Lipid A modification systems in Gram-negative bacteria, Annu. Rev. Biochem., 2007, 76, 295–329 CrossRef CAS PubMed.
- C. R. H. Raetz and C. Whitfield, Lipopolysaccharide endotoxins, Annu. Rev. Biochem., 2002, 71, 635–700 CrossRef CAS PubMed.
- C. Galanos,
et al., Synthetic and natural Escherichia coli free lipid A express identical endotoxic activities, Eur. J. Biochem., 1985, 148, 1–5 CrossRef CAS PubMed.
- S. G. Wilkinson, Bacterial lipopolysaccharides-themes and variations, Prog. Lipid Res., 1996, 35, 283–343 CrossRef CAS PubMed.
- A. H. Williams and C. R. H. Raetz, Structural basis for the acyl chain selectivity and mechanism of UDP-N-acetylglucosamine acyltransferase, Proc. Natl. Acad. Sci. U. S. A., 2007, 104, 13543–13550 CrossRef CAS PubMed.
- L. Buetow, T. K. Smith, A. Dawson, S. Fyffe and W. N. Hunter, Structure and reactivity of LpxD, the N-acyltransferase of lipid A biosynthesis, Proc. Natl. Acad. Sci. U. S. A., 2007, 104, 4321–4326 CrossRef CAS PubMed.
- B. E. Coggins, X. Li, A. L. McClerren, O. Hindsgaul, C. R. H. Raetz and P. Zhou, Structure of the LpxC deacetylase with a bound substrate-analog inhibitor, Nat. Struct. Biol., 2003, 10, 645–651 CrossRef CAS PubMed.
- A. H. Williams, R. M. Immormino, D. T. Gewirth and C. R. H. Raetz, Structure of UDP-N-acetylglucosamine acyltransferase with a bound antibacterial pentadecapeptide, Proc. Natl. Acad. Sci. U. S. A., 2006, 103, 10877–10882 CrossRef CAS PubMed.
- A. W. Barb, A. L. McClerren, K. Snehelatha, C. M. Reynolds, P. Zhou and C. R. H. Raetz, Inhibition of lipid A biosynthesis as the primary mechanism of CHIR-090 antibiotic activity in Escherichia coli, Biochemistry, 2007, 46, 3793–3802 CrossRef CAS PubMed.
- D. H. Persing, R. N. Coler, M. J. Lacy, D. A. Johnson, J. R. Baldridge, R. M. Hershberg and S. G. Reed, Taking toll: lipid A mimetics as adjuvants and immunomodulators, Trends Microbiol., 2002, 10, 32–37 CrossRef PubMed.
- L. D. Hawkins, W. J. Christ and D. P. Rossignol, Inhibition of endotoxin response by synthetic TLR4 antagonists, Curr. Top. Med. Chem., 2004, 4, 1147–11471 CrossRef CAS PubMed.
- A. G. Stöver, J. D. S. Correia, J. T. Evans, C. W. Cluff, M. W. Elliott, E. W. Jeffery, D. A. Johnson, M. J. Lacy, J. R. Baldridge, P. Probst, R. J. Ulevitch, D. H. Persing and R. M. Hershberg, Structure–activity relationship of synthetic toll-like receptor 4 agonists, J. Biol. Chem., 2004, 279, 4440–4449 CrossRef PubMed.
- M. Chang, Effects of Coptis chinensis, Radix Paeoniae Rubra and Rhubarb on endotoxin release of Escherichia coli, Zhongcaoyao, 2007, 29, 752–753 Search PubMed.
- W. Cui, In vitro inhibitory effect of aloe medicated serum on endotoxin, Chin. Herb. Med., 2004, 35, 1163–1164 Search PubMed.
- M. P. Chapotchartier and S. Kulakauskas, Cell wall structure and function in lactic acid bacteria, Microb. Cell Fact., 2014, 13, 1–23 CrossRef PubMed.
- M. G. Pinho, M. Kjos and J. W. Veening, How to get (a)round: mechanisms controlling growth and division of coccoid bacteria, Nat. Rev. Microbiol., 2013, 11, 601–614 CrossRef CAS PubMed.
- T. D. Bugg, D. Braddick, C. G. Dowson and D. I. Roper, Bacterial cell wall assembly: still an attractive antibacterial target, Trends Biotechnol., 2011, 29, 167–173 CrossRef CAS PubMed.
- A. Gautam, R. Vyas and R. Tewari, Peptidoglycan biosynthesis machinery: a rich source of drug targets, Crit. Rev. Biotechnol., 2011, 31, 295–336 CrossRef CAS PubMed.
- H. Barreteau, A. Kovac, A. Boniface, M. Sova, S. Gobec and D. Blanot, Cytoplasmic steps of peptidoglycan biosynthesis, FEMS Microbiol. Rev., 2008, 32, 168–207 CrossRef CAS PubMed.
- N. Ruiz, Bioinformatics identification of MurJ (MviN) as the peptidoglycan lipid II flippase in Escherichia coli, Proc. Natl. Acad. Sci. U. S. A., 2008, 105, 15553–15557 CrossRef CAS PubMed.
- E. Sauvage, F. Kerff, M. Terrak, J. A. Ayala and P. Charlier, The penicillin-binding proteins: structure and role in peptidoglycan biosynthesis, FEMS Microbiol. Rev., 2008, 32, 234–258 CrossRef CAS PubMed.
- P. Macheboeuf, C. Contreras-Martel, V. Job, O. Dideberg and A. Dessen, Penicillin binding proteins: key players in bacterial cell cycle and drug resistance processes, FEMS Microbiol. Rev., 2006, 30, 673–691 CrossRef CAS PubMed.
- R. A. Daniel and J. Errington, Control of cell morphogenesis in bacteria: two distinct ways to make a rod-shaped cell, Cell, 2003, 113, 767–776 CrossRef CAS PubMed.
- H. Sun, Y. Yang, T. Xue and B. Sun, Modulation of cell wall synthesis and susceptibility to vancomycin by the two-component system AirSR in Staphylococcus aureus NCTC8325, BMC Microbiol., 2013, 10, 213–286 Search PubMed.
- J. F. Mariscotti, J. J. Quereda, F. G. D. Portillo and M. G. Pucciarelli, The Listeria monocytogenes LPXTG surface protein Lmo1413 is an invasin with capacity to bind mucin, Int. J. Med. Microbiol., 2014, 304, 393–404 CrossRef CAS PubMed.
- J. J. Quereda, A. D. Ortega, M. G. Pucciarelli and F. G. D. Portillo, The Listeria Small RNA Rli27 regulates a cell wall protein inside eukaryotic cells by targeting a long 5′-UTR variant, PLoS Genet., 2014, 10, 1004765 CrossRef PubMed.
- A. J. F. Egan, J. Biboy, I. V. Veer, E. Breukink and W. Vollmer, Activities and regulation of peptidoglycan synthases, Philos. Trans. R. Soc., B, 2015, 370, 1–20 CrossRef PubMed.
- A. Typas, J. Errington and W. Vollmer, Regulation of peptidoglycan synthesis by outer-membrane proteins, Cell, 2010, 143, 1097–1109 CrossRef CAS PubMed.
- A. S. Michalopoulos, I. G. Livaditis and V. Gougoutas, The revival of fosfomycin, Int. J. Infect. Dis., 2011, 15, 732–737 CrossRef PubMed.
- K. Bush, Introduction to antimicrobial therapeutics reviews: the bacterial cell wall as an antimicrobial target, Ann. N. Y. Acad. Sci., 2013, 1277, V–VII CrossRef PubMed.
-
C. G. Gemmell, Effects of low concentrations of antibiotics on bacterial ultrastructure, virulence, and susceptibility to immunodefenses: clinical significance, Antibiotics in laboratory medicine, 1996, pp. 397–452 Search PubMed.
- J. M. T. Hamilton-Miller and S. Shah, Disorganization of cell division of methicillin-resistant Staphylococcus aureus by a component of tea (Camelliasinensis): a study by electron microscopy, FEMS Microbiol. Lett., 1999, 176, 463–469 CrossRef CAS PubMed.
- P. Bernal, M. Zloh and P. W. Taylor, Disruption of D-alanyl esterification of Staphylococcus aureus cell wall teichoic acid by the-lactam resistance modifier (−)-epicatechin gallate, J. Antimicrob. Chemother., 2009, 63, 1156–1162 CrossRef CAS PubMed.
- M. Liu, W. Peng, R. Qin, Z. Yan, Y. Cen, X. Zheng, X. Pan, W. Jiang, B. Li, X. Li and H. Zhou, The direct anti-MRSA effect of emodin via damaging cell membrane, Appl. Microbiol. Biotechnol., 2015, 99, 7699–7709 CrossRef CAS PubMed.
- H. S. Sader, R. N. Jones, K. L. Rossi and M. J. Rybak, Occurrence of vancomycin-tolerant and heterogeneous vancomycin-intermediate strains (hVISA) among Staphylococcus aureus causing bloodstream infections in nine USA hospitals, J. Antimicrob. Chemother., 2009, 64, 1024–1028 CrossRef CAS PubMed.
- D. J. Farrell, M. Robbins, W. Williams and W. G. Love, Investigation of the potential for mutational resistance to XF-73, retapamulin, mupirocin, fusidic acid, daptomycin, and vancomycin in methicillin-resistant Staphylococcus aureus isolates during a 55-passage study, Antimicrob. Agents Chemother., 2011, 55, 1177–1181 CrossRef CAS PubMed.
- J. Sui, K. Xie, W. Zou and M. Xie, Emodin inhibits breast cancer cellproliferation through the ERalpha-MAPK/Akt-cyclin D1/Bcl-2 signaling pathway, Asian Pac. J. Cancer Prev., 2014, 15, 6247–6251 CrossRef PubMed.
- L. Ma and W. Li, Emodin inhibits LOVO colorectal cancer cell proliferation via the regulation of the Bcl-2/Bax ratio and cytochrome, Exp. Ther. Med., 2014, 4, 1225–1228 CrossRef PubMed.
- L. Zhao, L. Zhang, J. Liu, L. Wan, Y. Chen, S. Zhang, Z. Yan and J. Jiang, Synthesis and antitumor activity of conjugates of 5-Fluorouracil and emodin, Eur. J. Med. Chem., 2012, 1, 255–260 CrossRef PubMed.
- F. Cao, W. Peng, X. Li, M. Liu, B. Li, R. Qin, W. Jiang, Y. Cen, X. Pan, Z. Yan, K. Xiao and H. Zhou, Emodin is identified as the active component of ether extracts from Rhizoma Polygoni Cuspidati, for anti-MRSA activity, Can. J. Physiol. Pharmacol., 2015, 93, 485–493 CrossRef CAS PubMed.
- C. Zheng, Study on antibacterial activity and mechanism of acetone extract from madder, Shipin Gongye Keji, 2015, 36, 116–119 Search PubMed.
- Y. Wang, Bacteriostatic mechanism of Polygonum cuspidatum extract on apple rot pathogen, Chinese Journal of Biological Control, 2015, 31, 148–156 Search PubMed.
- S. Mahanty and K. Rathinasamy, The natural anthraquinone dye purpurin exerts antibacterial activity by perturbing the FtsZ assembly, Bioorg. Med. Chem., 2021, 50, 116–150 CrossRef PubMed.
- L. Jiang, T. Yi, Z. Shen, Z. Teng and J. Wang, Aloe-emodin attenuates Staphylococcus aureus pathogenicity by interfering with the oligomerization of α-toxin, Front. Cell. Infect. Microbiol., 2019, 9, 157–188 CrossRef CAS PubMed.
- F. Xu, R. Diao, J. Liu, Y. Kang, X. Wang and L. Shi, Curcumin attenuates Staphylococcus aureus-induced acute lung injury, Clin. Respir. J., 2015, 9, 87–97 CrossRef CAS PubMed.
- J. Qiu, X. Niu, J. Dong, D. Wang, J. Wang, H. Li, M. Luo, S. Li, H. Feng and X. Deng, Baicalin protects mice from Staphylococcus aureus pneumonia via inhibition of the cytolytic activity of α-hemolysin, J. Infect. Dis., 2012, 15, 292–301 CrossRef PubMed.
- J. Azelmat, F. Larente and D. Grenier, The anthraquinone rhein exhibits synergistic antibacterial activity in association with metronidazole or natural compounds and attenuates virulence gene expression in Porphyromonas gingivalis, Arch. Oral Biol., 2015, 60, 342–346 CrossRef CAS PubMed.
- J. Liao, L. Zhao, M. Yoshioka, D. Hinode and D. Grenier, Effects of Japanese traditional herbal medicines (Kampo) on growth and virulence properties of Porphyromonas gingivalis and viability of oral epithelial cells, Pharm. Biol., 2013, 51, 1538–1544 CrossRef CAS PubMed.
- C. Lu, H. Wang, W. Lv, P. Xu and J. Zhu, Antibacterial properties of anthraquinones extracted from rhubarb against Aeromonas hydrophila, Fish. Sci., 2011, 77, 375–384 CrossRef CAS.
- S. Khan, S. A. A. Nami, K. S. Siddiqi, E. Husain and I. Naseem, Synthesis and characterization of transition metal 2,6-pyridine-dicarboxylic acid derivatives, interactions of Cu(II) and Ni(II) complexes with DNA in vitro, Spectrochim. Acta, Part A, 2009, 72, 421–428 CrossRef PubMed.
- Y. Ankita and B. R. A. S. Richa, Phytochemical screening and antimicrobial activity of anthraquinones isolated from different parts of Cassia nodosa, Res. J. Med. Plant, 2013, 7, 150–157 CrossRef.
- L. Yu, H. Xiang, J. Fan, D. Wang, F. Yang, N. Guo, Q. Jin and X. Deng, Global transcriptional response of Staphylococcus aureus to rhein, a natural plant product, J. Biotechnol., 2008, 135, 304–308 CrossRef CAS PubMed.
- S. M. Daly, B. O. Elmore, J. S. Kavanaugh, K. D. Triplett, N. H. Oberlies, M. Figueroa, H. A. Raja, T. E. Elimat, H. A. Crosby, J. K. Femling, N. B. Cech, A. R. Horswill, N. H. Oberlies and P. R. Hall, ω-Hydroxyemodin limits staphylococcus aureus quorum sensing-mediated pathogenesis and inflammation, Antimicrob. Agents Chemother., 2015, 59, 2223–2235 CrossRef CAS PubMed.
- L. Li, Y. Tian, J. Yu, X. Song, R. Jia, Q. Cui, W. Tong, Y. Zou, L. Li, L. Yin, X. Liang, C. He, G. Yue, G. Ye, L. Zhao, F. Shi, C. Lv, S. Cao and Z. Yin, iTRAQ-based quantitative proteomic analysis reveals multiple effects of Emodin to Haemophilus parasuis, J. Proteomics, 2017, 23, 39–47 CrossRef PubMed.
- S. Mahanty and K. Rathinasamy, The natural anthraquinone dye purpurin exerts antibacterial activity by perturbing the FtsZ assembly, Bioorg. Med. Chem., 2021, 15, 116463 CrossRef PubMed.
- X. Ji, X. Liu, Y. Peng, R. Zhan, H. Xu and X. Ge, Comparative analysis of methicillin-sensitive and resistant Staphylococcus aureus exposed to emodin based on proteomic profiling, Biochem. Biophys. Res. Commun., 2017, 494, 318–324 CrossRef CAS PubMed.
- J. Lee, Y. Kim, S. Y. Ryu and J. Lee, Calcium-chelating alizarin and other anthraquinones inhibit biofilm formation and the hemolytic activity of Staphylococcus aureus, Sci. Rep., 2016, 14, 192–197 Search PubMed.
- L. Zhao, L. Zhang, J. Liu, L. Wan, Y. Chen, S. Zhang, Z. Yan and J. Jiang, Synthesis and antitumor activity of conjugates of 5-Fluorouracil and emodin, Eur. J. Med. Chem., 2012, 1, 255–260 CrossRef PubMed.
- J. Pfeffer and A. J. Clarke, Identification of the first known inhibitors of O-acetylpeptidoglycan esterase: A potential new anti-bacterial target, ChemBioChem, 2012, 13, 722–731 CrossRef CAS PubMed.
- K. Kang, W. Fong and P. W. Tsang, Novel antifungal activity of purpurin against Candida species in vitro, Med. Mycol., 2010, 48, 904–911 CrossRef CAS PubMed.
- P. W. Tsang, H. M. Bandara and W. P. Fong, Purpurin suppresses Candida albicans biofilm formation and hyphal development, PLoS One, 2012, 7, 508–566 Search PubMed.
- P. W. Tsang, A. P. Wong, H. S. Jung and W. P. Fong, Sub-MIC levels of purpurin inhibit membrane ATPase-mediated proton efflux activity in the human fungal pathogen Candida albicans, J. Antibiot., 2014, 67, 349–350 CrossRef CAS PubMed.
- P. W. Tsang, A. P. Wong, H. Yang and N. Li, Purpurin triggers caspase-independent apoptosis in Candida dubliniensis biofilms, PLoS One, 2013, 8, 86032 CrossRef PubMed.
- M. A. Lobritz, P. Belenky, C. B. M. Porter, A. Gutierrez, J. H. Yang, D. J. Dwyer, A. S. Khalil and J. J. Collins, Antibiotic efficacy is linked to bacterial cellular respiration, Proc. Natl. Acad. Sci. U. S. A., 2015, 112, 8173–8180 CrossRef CAS PubMed.
- K. Kawai, T. Kato, H. Mori, J. Kitamura and Y. Nozawa, A comparative study on cytotoxicities and biochemical properties of anthraquinone mycotoxins emodin and skyrin from Penicillium islandicum Sopp, Toxicol. Lett., 1984, 20, 155–160 CrossRef CAS PubMed.
- T. Ubbink-Kok, J. A. Anderson and W. N. Konings, Inhibition of electron transfer and uncoupling effects by emodin and emodinanthrone in Escherichia coli, Antimicrob. Agents Chemother., 1986, 30, 147–151 CrossRef CAS PubMed.
- J. Liu, X. Gao, T. Lian, A. Zhao and K. Li, Apoptosis of human hepatoma HepG2 cells induced by emodin in vitro, Aizheng, 2003, 22, 1280–1283 CAS.
- D. E. Shieh, Y. Chen, M. Yen, L. Chiang and C. Lin, Emodin-induced apoptosis through p53-dependent pathway in human hepatoma cells, Life Sci., 2004, 74, 2279–2290 CrossRef CAS PubMed.
- L. Zhou, B. Yun and Y. Wang, Antimicrobial mechanism of emodin on Staphylococcus aureus, Zhongguo Shengwu Huaxue Yu Fenzi Shengwu Xuebao, 2011, 27, 5–18 Search PubMed.
- J. Wang, Y. Cheng, R. Wu, D. Jiang, B. Bai, D. Tan, T. Yan, X. Sun, Q. Zhang and Z. Wu, Antibacterial Activity of Juglone against Staphylococcus aureus: From Apparent to Proteomic, Int. J. Mol. Sci., 2016, 17, 965–983 CrossRef PubMed.
- S. M. Abu-darwish, M. Ateyyata and A. Salt, The Pharmacological and Pesticidal Actions of Naturally Occurring 1, 8-dihydroxyanthraquinones Derivatives, Helicobacter, 2008, 4, 495–505 Search PubMed.
- L. K. Omosa, J. O. Midiwo, A. T. Mbaveng, S. B. Tankeo, J. A. Seukep, I. K. Voukeng, J. K. Dzotam, J. Isemeki, S. Derese, R. A. Omolle, T. Efferth and V. Kuete, Antibacterial activities and structure – activity relationships of a panel of 48 compounds from Kenyan plants against multidrug resistant phenotypes, SpringerPlus, 2016, 5, 901 CrossRef PubMed.
- D. J. Farrell, M. Robbins, W. Rhys-Williams and W. G. Love, Investigation of the potential for mutational resistance to XF-73, retapamulin, mupirocin, fusidic acid, daptomycin, and vancomycin in methicillin-resistant Staphylococcus aureus isolates during a 55-passage study, Antimicrob. Agents Chemother., 2011, 55, 1177–1181 CrossRef CAS PubMed.
- Y. Huang, G. Huang, M. Wu, H. Tang, Z. Huang, X. Zhou, W. Yu, J. Su, X. Mo, B. Chen, L. Zhao, X. Huang, H. Wei and L. Wei, Inhibitory effects of emodin, baicalin, schizandrin and berberine on hefA gene : Treatment of Helicobacter pylori – induced multidrug resistance, World J. Gastroenterol., 2015, 21, 4225–4231 CrossRef CAS PubMed.
- L. R. Comini, S. C. N. Montoya, P. L. Páez, G. A. Argüello, I. Albesa and J. L. Cabrera, Antibacterial activity of anthraquinone derivatives from Heterophyllaea pustulata (Rubiaceae), J. Photochem. Photobiol., B, 2011, 102, 108–114 CrossRef CAS PubMed.
- C. Pellieux, A. Dewilde, C. Pierlot and J. M. Aubry, Bactericidal and virucidal activities of singlet oxygen generated by thermolysis of naphthalene endoperoxides, Methods Enzymol., 2000, 3, 197–207 Search PubMed.
- K. Zerdin, M. Horsham and R. Durham, Photodynamic inactivation of bacterial spores on the surface of a photoactive polymer, React. Funct. Polym., 2009, 69, 821–827 CrossRef CAS.
- Z. Luksiené and T. Maisch, New approach to inactivation of harmful and pathogenic microorganisms by photosensitization, Food Technol. Biotechnol., 2005, 43, 411–418 Search PubMed.
- M. A. Kohanski, D. J. Dwyer, B. Hayete, C. A. Lawrence and J. J. Collins, A common mechanism of cellular death induced by bactericidal antibiotics, Cell, 2007, 130, 797–810 CrossRef CAS PubMed.
- M. C. Becerra and I. Albesa, Oxidative stress induced by criprofloxacin in Staphylococcus aureus, Biochem. Biophys. Res. Commun., 2002, 297, 1003–1007 CrossRef CAS PubMed.
- I. Albesa, M. C. Becerra, P. C. Battán and P. L. Páez, Oxidative stress involved in the antibacterial action of different antibiotics, Biochem. Biophys. Res. Commun., 2004, 317, 605–609 CrossRef CAS PubMed.
- K. Kang, W. P. Fong and P. W. Tsang, Novel anti-fungal activity of purpurin against Candida species in vitro, Med. Mycol., 2010, 48, 904–911 CrossRef CAS PubMed.
- P. W. Tsang, A. P. Wong and H. P. Yang, Purpurin triggers caspase-independent apoptosis in Candida dubliniensis biofilms, PLoS One, 2013, 8, e86032 CrossRef PubMed.
- P. W. Tsang, A. P. Wong, H. S. Jung and W. P. Fong, Sub-MIC levels of purpurin inhibit membrane ATPase-mediated proton efflux activity in the human fungal pathogen Candida albicans, J. Antibiot., 2014, 67, 349–350 CrossRef CAS PubMed.
- P. L. Gutierrez, The metabolism of quinone-containing alkylating agents: Free radical production and measurement, Front. Biosci.-Landmark, 2000, 5, 629–638 Search PubMed.
- J. L. Cape, M. K. Bowman and D. M. Kramer, Computation of the redox and protonation properties of quinones: Towards the prediction of redox cycling natural products, Phytochemistry, 2006, 67, 1781–1788 CrossRef CAS PubMed.
- M. Ishimur, M. Suda, K. Morizumi, S. Kataoka, T. Maeda, S. Kurokawa and Y. Hiyama, Effects of KP-496, a novel dual antagonist at the cysteinyl leukotriene receptor 1 and the thromboxane A(2) receptor, on airway obstruction in guinea pigs, Br. J. Pharmacol., 2008, 153, 669–675 CrossRef PubMed.
- D. Wang, X. Wang, X. Yu, F. Cao, X. Cai, P. Chen, M. Li, Y. Feng, H. Li and X. Wang, Pharmacokinetics of anthraquinones from medicinal plants, Front. Pharmacol., 2021, 12, 638–693 Search PubMed.
- C. P. Wu, S. H. Hsiao and M. Murakami,
et al., Alpha-mangostin reverses multidrug resistance by attenuating the function of the multidrug resistance-linked ABCG2 transporter, Mol. Pharmaceutics, 2017, 14, 2805–2814 CrossRef CAS PubMed.
- R. J. Young and P. D. Leeson, Mapping the Efficiency and Physicochemical Trajectories of Successful Optimizations, J. Med. Chem., 2018, 61, 6421–6467 CrossRef CAS PubMed.
- G. S. Cremosnik, J. Liu and H. Waldmann, Guided by evolution: from biology oriented synthesis to pseudo natural products, Nat. Prod. Rep., 2020, 37, 1497–1510 RSC.
|
This journal is © The Royal Society of Chemistry 2023 |
Click here to see how this site uses Cookies. View our privacy policy here.