DOI:
10.1039/D2NJ04282G
(Paper)
New J. Chem., 2023,
47, 75-83
A novel delivery vehicle for copper peptides†
Received
28th August 2022
, Accepted 13th November 2022
First published on 24th November 2022
Abstract
While glycyl-L-histidyl-L-lysine-copper (GHK-Cu) has a variety of biological functions, it is not easily absorbed by skin directly, which along with several contraindications, greatly limit its clinical use. We developed an ionic-liquid-based delivery vehicle composed of betaine and tartaric acid to address the difficulties associated with the use of GHK-Cu. The prepared ionic liquid is safe, stable, and able to deliver a high GHK-Cu drug-loading capacity while maintaining the activity of GHK-Cu and ensuring its biological functions. In addition, it substantially improves the permeability of GHK-Cu to deeper skin layers. The ionic-liquid delivery vehicle, which was developed by combining experimental and theoretical studies, provides a simple, low-cost, and effective method for applying GHK-Cu while providing design ideas for active peptides in general.
1. Introduction
Skin, the largest human organ, is essential for physiological and natural interactions involving the body, while also protecting its functions. However, skin ages as the body ages, with the external environment damaging this important body barrier to a certain degree, which not only affects its apparent morphology but, more importantly, results in skin fragility and delayed wound healing, increasing the risk of infection and skin cancer.1,2 Skin ages slowly but continuously, particularly through exogenous photoaging, which is the leading cause of skin aging.3 Daily exposure to UV light can result in the production of matrix metalloproteinases (MMPs) in the skin. MMPs belong to a family of proteins that include at least 14 secretory or transmembrane proteins that age skin and increase its fragility by degrading extracellular-matrix and basement-membrane components.4 Due to the presence of matrix metalloproteinase tissue inhibitors (TIMPs) that inhibit its secretion, healthy skin contains extremely low levels of MMP-1, thereby retarding the breakdown of collagen (the main extracellular matrix backbone).5–7 In addition, exposing skin to UV radiation produces reactive oxygen species (ROS) that damage the skin barrier and/or DNA.8,9 Excessive accumulation of ROS leads to oxidative stress, which further accelerates premature skin aging.10 Glycyl-L-histidyl-L-lysine (GHK) is a tripeptide that was first extracted from human plasma by Pickart and Thaler in 1973. This small peptide has a strong affinity for copper(II) ions and spontaneously forms GHK-Cu, a tripeptide-copper complex.11,12 Copper is an essential cofactor involved in the formation of collagen and elastin; it downregulates MMP, upregulates TIMP-1 and -2, and diminishes collagenase activity.13
The copper atoms in GHK-Cu chelate with the α-amino nitrogen of glycine, the amido nitrogen between the amide and histidine residues, and the bound nitrogen on the imidazole ring of the histidine residue, which engenders GHK with favorable Cu-carrier properties.12,14 The antioxidant activity of GHK-Cu is primarily mediated by blocking the formation of reactive carbonyl products, inactivating the toxic products of lipid peroxidation, and protecting keratin-forming cells from lethal UV radiation.15,16 Meanwhile, GHK-Cu inhibits ferritin-iron release by 87%, which ameliorates the triggering-impact of iron during lipid peroxidation. Skin also benefits from the abovementioned processes through their antioxidant effects.11 In addition, GHK-Cu has been demonstrated to positively affect fibroblasts, which are key cells involved in skin-repair and renewal processes. Unfortunately, GHK-Cu is highly hydrophilic, with log P values of −2.49 ± 0.33 and −2.49 ± 0.35 at pH 4.5 and 7.4, respectively; consequently, it is hardly directly absorbed by skin.11,17,18 Moreover, there are many contraindications for the practical use of GHK-Cu. For example, it cannot be used concurrently with antioxidants or chelating agents; therefore, the existing methods are not sufficient for the practical application of GHK-Cu, and the development of new methods is of great importance (Table S1, ESI†).
In this study, comprehensive analysis and targeted design led to the selection of natural betaine and tartaric acid as the cationic and anionic moieties of a green ionic liquid, which was prepared through ion-exchange. Computational chemistry was used to determine the intermolecular interaction force associated with the ionic liquid. GHK-Cu was encapsulated by the prepared ionic liquid; it was efficiently loaded while maintaining its bioactivity, exhibiting significant antioxidant activity at the cellular level. This ionic-liquid delivery carrier notably promoted the absorption of GHK-Cu by skin, resulting in a substantial increase in GHK-Cu bioavailability (Scheme 1).
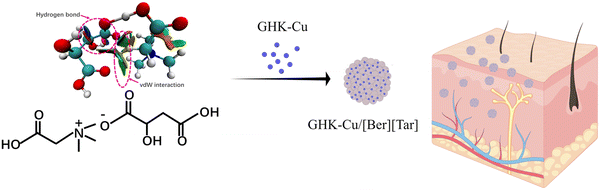 |
| Scheme 1 Schematic diagram of [Bet][Tar] synthesis and application. | |
2. Results and discussion
2.1 Synthesizing, optimizing, and characterizing ionic-liquid-based delivery carriers
We selected betaine and tartaric acid as precursors through comprehensive analysis aided by experimental and DFT methods, and prepared corresponding ionic liquids. Betaine is an alkaloid found in the roots, stems, leaves, and fruits of plants, while tartaric acid is an organic acid present in various plants; both are highly biocompatible, and the prepared ionic liquids are very safe. We synthesized ionic liquids with various molar ratios of betaine and tartaric acid (1
:
1, 1
:
2, 1
:
3, 1
:
4, 2
:
1, and 3
:
1); these ionic liquids are clear liquids. The DFT-generated reduced density gradient (RDG) diagram revealed the presence of van der Waals interactions between betaine and tartaric acid,19,20 while the electrostatic potential (ESP) diagram reveals that betaine/tartaric acid attraction leads to the formation of the ILs monomer, with the positively charged region of betaine observed to be in close proximity to the negatively charged region of tartaric acid (Fig. 1a and b), resulting in a stable [Bet][Tar] configuration. Both the ESP and RDG diagrams show that the prepared ILs are very stable (Fig. 1b). The Fourier-transform infrared (FTIR) spectra are in good agreement with the computational data. An –OH stretching band associated with hydrogen-bonding interactions is observed between 3500 and 3000 cm−1, while an asymmetric stretching peak associated with the carboxyl group (–COOH) is observed between 1600 and 1640 cm−1, indicative of the formation of ionic hydrogen bonds between betaine and tartaric acid (Fig. 1c and d). The 1H NMR spectrum of [Bet][Tar] shows signals of methylene (δ(H) = 4.35) and methyl (δ(H) = 3.3) protons (Fig. S1, ESI†). Based on the data presented above, we conclude that the ILs were successfully synthesized.
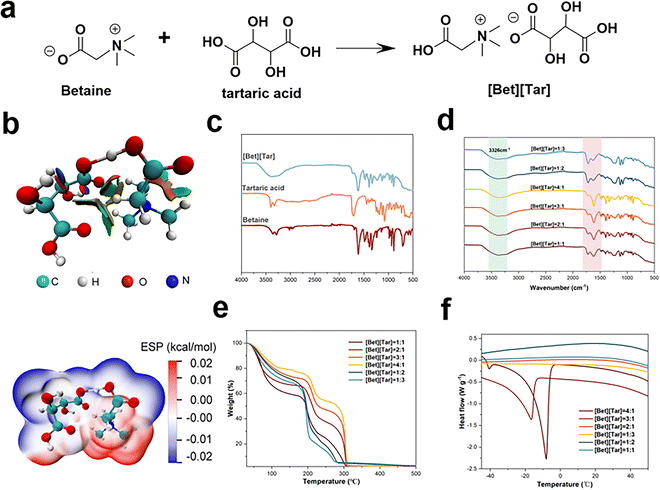 |
| Fig. 1 Synthesizing and characterizing [Bet][Tar]. (a) Preparing [Bet][Tar]. (b) RDG and ESP diagrams. (c) FTIR spectra of betaine, tartaric acid, and [Bet][Tar]. (d) FTIR spectra of [Bet][Tar] in various molar ratios. (e) TGA traces of [Bet][Tar] in various molar ratios. (f) DSC traces of [Bet][Tar] in various molar ratios. | |
The thermal properties of ionic liquids can be used to evaluate their usefulness in delivery applications.21,22 Glass-transition temperatures (Tg) of between −8 and −20 °C were determined (Fig. 1e), while thermogravimetric analyses revealed that the various [Bet][Tar] compositions decompose between 180 and 240 °C. Among the examined ILs, that prepared with a betaine/tartaric acid molar ratio of 3
:
1 exhibited the best thermal stability (Fig. 1f). To summarize, we successfully prepared betaine and tartaric acid ionic liquids with various molar ratios and found that ionic liquids with different molar ratios have higher stability.
2.2 Physicochemical properties of ionic-liquid-based delivery carriers
The physicochemical properties of an ionic liquid is closely related to its constituents and the ratio of each component; consequently, they can be modified to match the required purpose. Rheometer testing revealed that the viscosities of [Bet][Tar] with various molar ratios lie in the 82–170 mPa s range at room temperature (Fig. 2a) and decrease dramatically with increasing temperature (Fig. 2b). The observed viscosity range is suitable for delivery carriers, and indicates that viscosity does not hinder the use of [Bet][Tar] for drug-delivery applications. ILs density was determined to range between 1.16 and 1.18 g m−3, with small differences observed between samples (Fig. 2a). The change in conductivity is closely related to the degree of hydrogen bonding and viscosity (Fig. 2c). The pH variation for different molar ratios is 3.8–6.2 and GHK-Cu is highly sensitive to pH; therefore it is crucial to choose a suitable molar ratio (Fig. 2d). Kamlet–Taft solvatochromic parameters, dipole/polarization rate (π*), and hydrogen-bond alkalinity (β) determine the microscopic properties of ILs.23 All [Bet][Tar] samples were found to exhibit high β values (about 0.4–0.8) (Fig. 2e). As the π* values of weakly polar molecular solvents are generally lower than those of water, [Bet][Tar] greatly enhances affinity for weakly polar and bioactive compounds. In short, [Bet][Tar] exhibits physicochemical properties that clearly depend on the molar ratio.
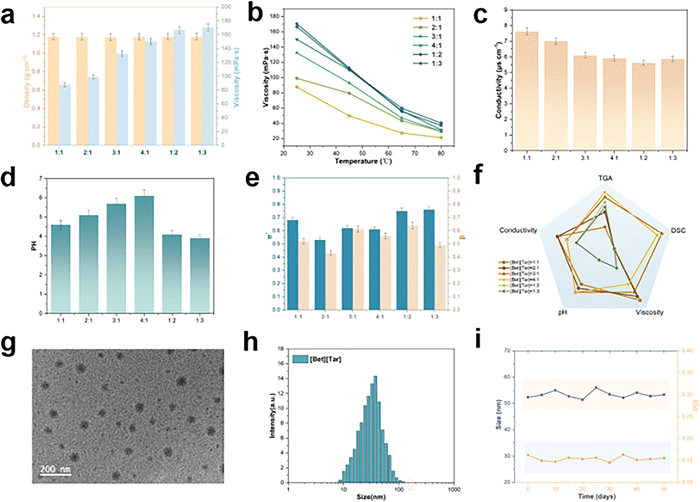 |
| Fig. 2 Physicochemical properties of ionic-liquid-based delivery carriers. (a and b) [Bet][Tar] density and viscosity as functions of molar ratio and temperature. [Bet][Tar] (c) conductivity and (d) pH as functions of molar ratio. (e) Kamlet–Taft parameters: dipolarity/polarizability (π*) and hydrogen-bond basicity (β) of [Bet][Tar] at room temperature. (f) Overview summarizing the physicochemical properties of [Bet][Tar] in various molar ratios. (g) TEM image and (h) particle-size distribution of [Bet][Tar]. (i) [Bet][Tar] particle size as a functions of time over 50 d. | |
Considering the demands of GHK-Cu delivery carriers in terms of viscosity and pH, 3
:
1 [Bet][Tar] was chosen for use in subsequent experiments (Fig. 2f). [Bet][Tar] exhibited a uniform spherical morphology by cryogenic transmission electron microscopy (Cryo-TEM) (Fig. 2g). DLS experiments revealed particles 52.3 ± 1.68 nm in size with a dispersion index of 0.136 (Fig. 2h); particle size was observed to differ only slightly after 50 d of storage at room temperature, indicative of good stability (Fig. 2i). Taken together, the physicochemical properties, morphology, size, and stability of the prepared [Bet][Tar] meet the requirements of a GHK-Cu delivery carrier.
2.3 Evaluating the stability and safety of GHK-Cu encapsulated in an ionic-liquid-based delivery carrier
We used [Bet][Tar] in a 3
:
1 molar ratio to encapsulate GHK-Cu. An aqueous solution of GHK-Cu was dark blue, while GHK-Cu encapsulated by [Bet][Tar] was crystal blue and translucent (Fig. 3a). DLS revealed that encapsulation led to larger well-dispersed particles (65.58 ± 2.23 vs. 52.3 ± 1.68 nm) (Fig. 3b), and stability testing showed that particle size changed minimally at 0–32 °C over a 50 d period, with good dispersion consistently observed (Fig. 3c and d). In addition, In addition, we evaluated the stability of different levels of GHK-cu in IL by storage, high-speed centrifugation, particle size and FTIR changes, respectively, and all showed good stability (Fig. S2–S5 and Table S2, ESI†). [Bet][Tar] encapsulated up to 82.7% of the GHK-Cu, as by determined high-performance liquid chromatography (HPLC). Considering the requirement for carrier safety, we evaluated how toxic GHK-Cu/[Bet][Tar] is toward fibroblast (NHF) and breast-cancer cells (MCF-7). Fig. 3e and f shows that 0–800 μM GHK-Cu/[Bet][Tar] is effectively non-toxic toward both cell types, with cell viabilities of more than 95% observed in both cases, which indicates that GHK-Cu/[Bet][Tar] is highly safe.
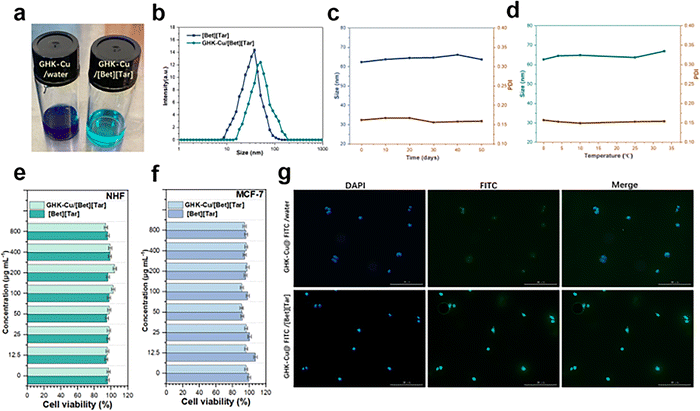 |
| Fig. 3 Evaluating the stability and safety of GHK-Cu encapsulated in an ionic-liquid-based delivery carrier. (a) Photographic images of GHK-Cu in water and [Bet][Tar]. (b) Particle-size distributions of CHK-Cu/water and CHK-Cu/[Bet][Tar]. (c and d) Particle size and PDI of CHK-Cu/[Bet][Tar] as functions of (c) time and (d) temperature. (e and f) Cytotoxicities of [Bet][Tar] and CHK-Cu/[Bet][Tar] toward (c) NHF cells and (f) MCF-7 cells. (g) Intracellular fluorescence images of NHF cells acquired by flow cytometry. | |
In addition, we labeled GHK-Cu with fluorescein isothiocyanate (FITC) and determined the cellular uptake of the FITC-labeled GHK-Cu by monitoring the absorption peak at 485 nm by UV spectrophotometry, which revealed that uptake was significantly improved (by a factor of 4.3) after [Bet][Tar] encapsulation (Fig. 3g). We conclude that [Bet][Tar] effectively encapsulates GHK-Cu, is highly stable and safe, and contributes greatly to the cellular uptake of GHK-Cu.
2.4 Ionic-liquid-based delivery carriers enhance GHK-Cu transdermal permeation efficiency
Absorption by the skin is one of the biggest issues associated with use of peptide treatments, including GHK-Cu, which is not only ascribable to hydrophilicity but also to the highly dense brick-wall structure of the outermost stratum corneum of the skin that blocks most substances from penetrating.24–27 Therefore, in vitro permeation experiments using isolated animal skin were used to effectively predict in vivo substance-permeation efficiency. We examined [Bet][Tar]-encapsulated GHK-Cu, with an aqueous solution of GHK-Cu used as the control. CLSM revealed that FITC-labeled GHK-Cu is brightly (green) fluorescent, and that the effectively permeated area of the mouse skin in the experimental group is 3.6-fold more fluorescent than that of the control group (Fig. 4a–c). Cumulative permeation curves show that the FITC-labeled GHK-Cu transdermally permeated mouse skin by a factor of 4.7 over the control (Fig. 4d). Further quantitative analysis showed that the total amount of GHK-Cu in the control mouse skin was not only low but also mainly concentrated in the stratum corneum. In contrast, GHK-Cu was significantly more retained in the mouse skin of the experimental group and mostly concentrated in the dermis (Fig. 4e). These trends are more intuitively observed in the tissue-section images, which show that [Bet][Tar] successfully delivers GHK-Cu to deeper layers of the skin. FTIR spectroscopy showed that the intensities of the peaks corresponding to the lipids in the stratum corneum of mouse skin (2850 cm−1, symmetric CH2 bond stretching; 2919 cm−1, asymmetric CH2 bond stretching) are different following transdermal experiment (Fig. 4f), consistent with lipid fluidization or extraction, which is most likely the mechanism by which [Bet][Tar] permeates GHK-Cu through the skin. In addition, no damage, irritation, or other alterations were observed when mouse skin was H&E stained after transdermal delivery (Fig. 4g). In other words, [Bet][Tar], as a transdermal-delivery carrier, helps GHK-Cu break through the barrier of the stratum corneum to smoothly reach deep skin layers, which is possibly ascribable to nanoparticles encapsulated with the [Bet][Tar] that greatly improve the ability of the drug to enter the skin; moreover, [Bet][Tar] is able to disturb lipids in the stratum corneum of skin, which further contributes to the skin-penetration rate of GHK-Cu.
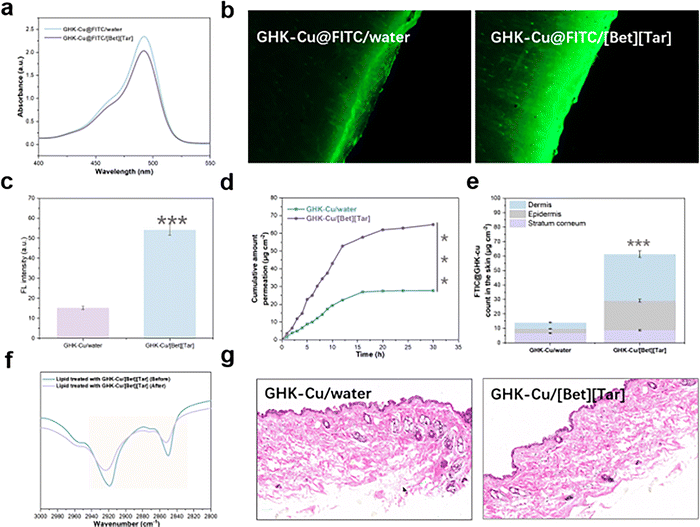 |
| Fig. 4 Ionic-liquid-based delivery carrier enhances GHK-Cu transdermal permeation efficiency. (a) Absorption spectra of GHK-Cu@FITC/water and GHK-Cu@FITC/[Bet][Tar]. (b and c) CLSM images of GHK-Cu@FITC/water and GHK-Cu@FITC/[Bet][Tar] on mouse skin and quantitative analyses (scalebars: 200 μm). (d) Cumulative permeation of GHK-Cu@FITC/water and GHK-Cu@FITC/[Bet][Tar]. (e) Quantifying GHK-Cu in skin layers. (f) FTIR spectra of the lipid region of stratum corneum samples before (blue) and after (red) 30 h of incubation with GHK-Cu@FITC/[Bet][Tar]. (g) H&E-stained sections of mouse skin after treatment with GHK-Cu@FITC/water and GHK-Cu@FITC/[Bet][Tar] (scalebars: 100 μm). | |
2.5 Biological efficacy of GHK-Cu after ionic-liquid loading
Assuring drug delivery is important for a drug-delivery carrier. Hence, validating the biological function of GHK-Cu after its encapsulation by [Bet][Tar] is essential. Senescence-associated β-galactosidase (SA-β-gal) staining revealed that UV, H2O2, and HU in the positive treatment group led to a marked increase in the proportion of NHF-senescent cells.28,29 The addition of GHK-Cu/[Bet][Tar] led to 35.7%, 58.1%, and 22.3% fewer senescent cells in the three groups, respectively, confirming that GHK-Cu/[Bet][Tar] effectively inhibits stress-induced cell senescence (Fig. 5a). The cell-proliferation curve reveals that GHK-Cu/[Bet][Tar] not only rescued cell-proliferation disorder caused by H2O2 but also further improved (by 11%) the abilities of uninjured cells to proliferate in the first six days (Fig. 5b). We also used flow cytometry to analyze ROS levels. H2O2 effectively increased the level of ROS in cells in the positive control group, while GHK-Cu/[Bet][Tar] downregulated ROS by 35.6% (Fig. 5c). This observation is further supported by DPPH radical-scavenging experiments (Fig. 5d). Various methodologies showed that GHK-Cu/[Bet][Tar] exhibits dramatic antioxidant effects. γ-H2AX is often used as a marker of DNA damage, with the amount present proportional to the degree of damage. Statistical analyses revealed that GHK-Cu/[bet][tar] reduces the damage caused by H2O2 and HU from 58.3% to 24.6%, and by 22.01%, respectively, confirming that GHK-Cu/[Bet][Tar] promotes DNA repair (Fig. 5e). MMP-1 expression is inversely proportional to collagen synthesis; the data show that H2O2 induces a striking increase in MMP-1 expression, and that the addition of GHK-Cu/[Bet][Tar] effectively inhibits this expression. VC is a recognized antioxidant that boosts collagen production. Testing revealed that both GHK-Cu/[Bet][Tar] and VC promote collagen production. In terms of anti-inflammatory effects, GHK-Cu/[Bet][Tar] was observed to inhibit the expression of UV-induced inflammatory factor IL-6 (inhibition rate = 18.06%), thereby demonstrating significant anti-inflammatory effects (Fig. 5f). We conclude that GHK-Cu/[Bet][Tar] is an excellent antioxidant, promotes DNA repair, and exhibits biological anti-inflammatory effects at the cellular level, consistent with previous results for GHK-Cu; consequently, [Bet][Tar] is a carrier that ensures the activity of GHK-Cu.
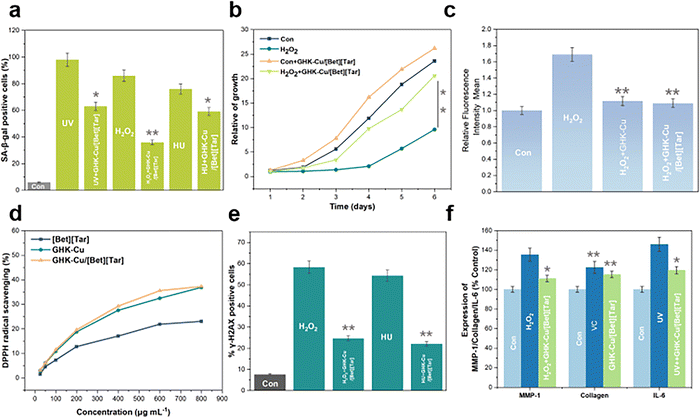 |
| Fig. 5 Biological efficacy of GHK-Cu after ionic-liquid loading. (a) Cellular senescence determined by SA-β-gal staining, with quantitative summaries of senescent cells shown (H2O2: 200 μM, HU: 200 μM). (b) NHF-cell growth curves during treatment with H2O2 and GHK-Cu/[Bet][Tar] for 6 d. (c) Intracellular ROS levels determined by flow cytometry. (d) DPPH radical-scavenging data for [Bet][Tar], GHK-Cu, and GHK-Cu/[Bet][Tar] in NHF cells. (e) Immunofluorescence staining results for γ-H2AX in MCF-7 cells treated with H2O2 and GHK-Cu/[Bet][Tar], with quantitative positive-cell summaries shown. (f) Effect of GHK-Cu/[Bet][Tar] on the production of MMP-1, collagen, and IL-6 in NHF cells. | |
3. Conclusion
In this study, we designed and synthesized a green ionic-liquid delivery carrier for GHK-Cu by considering its functional and applications challenges, and comprehensively characterized, analyzed, and verified its structure using both experimental and computational methods. The prepared ionic-liquid delivery vehicle is safe and stable, and efficiently loads and transports GHK-Cu to deeper layers of the skin. Furthermore, it maintains the activity of GHK-Cu and exhibits synergy, with excellent antioxidant, anti-wrinkle, and anti-inflammatory effects observed. Our study multi-dimensionally modulated mechanism, material, and application to construct an efficient transdermal delivery vehicle for GHK-Cu. This work solves intractable problems associated with applying GHK-Cu; the simplicity, green nature, and safety of the developed process greatly facilitate the clinical use of GHK-Cu while also providing a reference for the delivery of similar types of peptide.
4. Experimental section
4.1. Materials
Betaine and tartaric acid were obtained from Sigma–Aldrich (St. Louis, MO, USA), while DMEM cell-culture medium, fetal bovine serum (FBS), and trypsin were purchased from Gibco (BRL, MD, USA). FITC-labeled GHK-Cu was purchased from the Shanghai Chu Peptide Biotechnology Co., Ltd (Shanghai, China), and the CCK-8 kit, ROS test kit were purchased from the Biyuntian Biotechnology Co., Ltd (Shanghai, China). The DPPH kit was purchased from the Shanghai McLean Biotechnology Co., Ltd (Shanghai, China), Sirius Scarlet Stain (S8060) (Solarbio, Beijing, China), human (NHF) fibroblast cells and human mammary cancer (MCF-7) cells were purchased from the China Shanghai iCell Biotechnology Co., Ltd.
4.2. Synthesis and characterization
4.2.1 [Bet][Tar].
ILs were synthesized using different molar ratios (1
:
1, 1
:
2, 1
:
3, 1
:
4, 2
:
1, and 3
:
1) of betaine and tartaric acid at room temperature. The reaction mixture was maintained at 40 °C overnight in a water bath, after which it was dried under vacuum for 12 h and stored under argon.
4.2.2 GHK-Cu/[Bet][Tar].
The ILs was placed in a beaker and a GHK-Cu solution was slowly added dropwise with stirring using a pressure-equalizing funnel. The precipitate was collected by centrifugation, and GHK-Cu/ILs was obtained by dialysis with a dialysis bag.
4.3. Cell experiments
4.3.1
In vitro cytotoxicity assays.
A 96-well cell culture plate was inoculated with cells in the logarithmic growth phase (5000 cells per well) and incubated at 37 °C and in 5% CO2 for 24 h. Samples of various concentration were added to each column of the 96-well plate, after which the plate was returned to the incubator for 24 h (37 °C and 5% CO2). CCK-8 solution was then added to each well, and incubation was continued for 1–4 h with absorbance (450 nm) monitored using an enzyme marker (BioTek, Gen5). The cell-proliferation rate was calculated using the following equation: |  | (1) |
where Ablank is the absorbance of cell-free wells, Atest is the absorbance of cells in the test treatment group, and Acontrol is the absorbance of cells in the control treatment group.
4.3.2 Cellular uptake.
Logarithmic growth-phase cells were inoculated in six-well plates and incubated at 37 °C in 5% CO2 for 24 h. Various samples to be tested (100 μL) were added, and incubation was continued for 4 h. The medium was discarded and the cells were washed with PBS and fixed with 4% paraformaldehyde for 10 min, after which 10 μL of anti-quenching agent containing DAPI was added after washing with PBS. Cells were then examined by confocal microscopy (Nikon, Japan).
4.3.3 ROS levels.
Intracellular ROS levels were determined using the ROS detection kit, while intracellular superoxide radical levels were determined using dihydrobromoethane. For each sample group, 1 × 104 cells were harvested and immediately used in flow-cytometry experiments (CytoFLEX; Beckman, USA).
4.3.4 Immunofluorescence staining.
Cells grown on coverslips were fixed with a fixative for 10 min, after which they were permeabilized in 0.2% Triton X-100 for 10 min and incubated with 10% normal goat serum at 4 °C overnight. Coverslips were incubated with anti-phospho-H2AX antibody overnight at 4 °C, washed with PBS, and incubated with goat anti-mouse secondary antibody for 1 h at room temperature. The cells were washed three-times with PBS and stained with DAPI. Fluorescence images were acquired using the abovementioned fluorescence microscope.
4.4.
In vitro transdermal diffusion testing
4.4.1 Skin preparation.
The healthy female BALB/c mice (6–8 weeks old) used in this study were obtained from the Animal Center of Peking University Shenzhen Research Institute. All animal procedures were performed in accordance with the Guidelines for Care and Use of Laboratory Animals of Peking University (Shenzhen Research Institute) and experiments were approved by the Animal Ethics Committee of Peking University (Shenzhen Research Institute). The mice were first anesthetized with ether. Long mouse hairs were removed with an electric razor, and the remaining abdominal hair was removed using hair-removal cream, and the skin was peeled. The residual fat and mucus in the skin tissue were removed, rinsed repeatedly with saline, wrapped in plastic wrap, and stored at 4 °C in a refrigerator for one week.
4.4.2
In vitro skin-penetration testing.
In vitro permeation experiments were performed using a Franz diffusion cell. Fresh mouse skin was fixed between the diffusion cell and the receiving cell, with the dermis facing the latter. The receiving cell was filled with PBS buffer (pH 6.8), and magnets were placed. The sample to be tested was loaded into the diffusion cell, covered with skin, and then covered with cling film. The temperature and speed were set to 32 ± 2 °C and 320 rpm, respectively. Samples were collected from the receiving cell at intervals (V0 ≥ 2 mL per time) and replenished. Cumulative permeability was determined by HPLC using the following equation: | 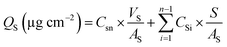 | (2) |
where Qs is the cumulative amount of drug penetrated per unit area (μg cm−2), Csn is the drug concentration (μg mL−1) in the received body fluid measured over n sampling intervals, and VS is the volume of the receptor pool (13 mL).
is the accumulated drug concentration in the receiving body fluid, S is the sample volume (2 mL), and AS is the effective diffusion area (1.0 cm2). Finally, the skins of percutaneous mice were collected and washed repeatedly with buffer. Sections of skin were fixed with 4% paraformaldehyde and embedded in paraffin for immunohistochemical staining.
4.4.3 Quantifying drug content in skin.
Transdermal mouse skin was collected, cleaned with phosphate buffer solution, and separated as follows. Ten strips of 3 M transparent adhesive were used to obtain the cuticle by lightly scraping the epidermis with a knife, with the remaining skin cut into effective diffusion zones and then further cut to obtain the dermis. The skin of each layer was separately suspended in methanol and GHK-Cu was ultrasonically extracted. The supernatant was collected by centrifugation at 1300 g for 15 min and filtered through a 0.45 μm membrane filter. Lastly, the drug content in each skin layer was determined by HPLC.
4.5. Computational chemistry
Molecular structures were optimized at the B3LYP-D3/6-311G** level of theory using Gaussian 09 software, with frequencies and ESPs analyzed.30 RDG analyses was performed using the Multiwfn and Winvmd programs.
4.6. Statistical analysis
Results are presented as means with standard deviations. One-way ANOVA was used to determine the significances of the differences. Statistical significance was set to: *p < 0.05 and **p < 0.01.
Data availability
Relevant data are available from the respective authors upon reasonable request.
Author contributions
Tianqi Liu: investigation, methodology, writing original draft, supervision, validation, formal analysis, project administration. Lu Hu: conceptualization, revise original draft, supervision, validation, formal analysis. Beibei Lu: writing – review & editing. Yiyang Bo: writing – review & editing. Ya Liao: writing – review & editing. Jingbo Zhan: writing – review & editing. Yunlin Pei: writing – review & editing. Huaiqing Sun: writing – review & editing. Zhenyuan Wang: writing – review & editing. Chao wan Guo: funding acquisition, project administration, supervision. Jiaheng Zhang: funding acquisition, project administration, resources, supervision.
Conflicts of interest
The authors delare no competing interest.
Acknowledgements
This work was financially supported by the National Natural Science Foundation of China (Grant No. 21905069, U21A20307), the Shenzhen Science and Technology Innovation Committee (Grant No. ZDSYS20190902093220279, KQTD20170809110344233, GXWD20201230155427003-20200821181245001, GXWD20201230155427003-20200821181809001, ZX20200151), the Department of Science and Technology of Guangdong Province (Grant No. 2020A1515110879).
References
- A. C. Franco, C. Aveleira and C. Cavadas, Skin senescence: mechanisms and impact on whole-body aging, Trends Mol. Med., 2022, 28(2), 97–109 CrossRef.
- M. E. C. Waaijer, D. Goldeck, D. A. Gunn, D. van Heemst, R. G. J. Westendorp, G. Pawelec and A. B. Maier, Are skin senescence and immunosenescence linked within individuals?, Aging Cell, 2019, 18(4), e12956 CrossRef.
- T. Kanaki, E. Makrantonaki and C. C. Zouboulis, Biomarkers of skin aging, Rev. Endocr. Metab. Disord., 2016, 17(3), 433–442 CrossRef CAS.
- T. Duellman, X. Chen, R. Wakamiya and J. Yang, Nucleic acid-induced potentiation of matrix metalloproteinase-9 enzymatic activity, Biochem. J., 2018, 475(9), 1597–1610 CrossRef CAS PubMed.
- I. Y. Dolmatov, V. A. Nizhnichenko and L. S. Dolmatova, Matrix Metalloproteinases and Tissue Inhibitors of Metalloproteinases in Echinoderms: Structure and Possible Functions, Cells, 2021, 10(9), 2331 CrossRef CAS PubMed.
- Y. Tao, L. Zhang, R. Yang, Y. Yang, H. Jin, X. Zhang, Q. Hu, B. He, Z. Shen and P. Chen, Corilagin ameliorates atherosclerosis by regulating MMP-1, -2, and -9 expression in vitro and in vivo, Eur. J. Pharmacol., 2021, 906, 174200 CrossRef CAS PubMed.
- J. H. Kim, H. D. Jeong, M. J. Song, D. H. Lee, J. H. Chung and S.-T. Lee, SOD3 Suppresses the Expression of MMP-1 and Increases the Integrity of Extracellular Matrix in Fibroblasts, Antioxidants, 2022, 11(5), 928 CrossRef CAS.
- B. Yang, Y. Chen and J. Shi, Reactive Oxygen Species (ROS)-Based Nanomedicine, Chem. Rev., 2019, 119(8), 4881–4985 CrossRef CAS PubMed.
- H. Sies and D. P. Jones, Reactive oxygen species (ROS) as pleiotropic physiological signalling agents, Nat. Rev. Mol. Cell Biol., 2020, 21(7), 363–383 CrossRef CAS PubMed.
- Y. Li, H. Bai, H. Wang, Y. Shen, G. Tang and Y. Ping, Reactive oxygen species (ROS)-responsive nanomedicine for RNAi-based cancer therapy, Nanoscale, 2018, 10(1), 203–214 RSC.
- L. Pickart and A. Margolina, Regenerative and Protective Actions of the GHK-Cu Peptide in the Light of the New Gene Data, Int. J. Mol. Sci., 2018, 19(7), 1987 CrossRef PubMed.
- L. Pickart, J. M. Vasquez-Soltero and A. Margolina, The Effect of the Human Peptide GHK on Gene Expression Relevant to Nervous System Function and Cognitive Decline, Brain Sci., 2017, 7(2), 20 CrossRef.
- R. Saito, Y. Miki, N. Ishida, C. Inoue, M. Kobayashi, S. Hata, H. Yamada-Okabe, Y. Okada and H. Sasano, The Significance of MMP-1 in EGFR-TKI–Resistant Lung Adenocarcinoma: Potential for Therapeutic Targeting, Int. J. Mol. Sci., 2018, 19(2), 609 CrossRef PubMed.
- Y. Meng, S. Kuang, H. Liu, Q. Fan, X. Ma and S. Zhang, Recent Advances in Electrochemical CO2 Reduction Using Copper-Based Catalysts, Acta Phys.-Chim. Sin., 2021, 37(5), 2006034 Search PubMed.
- S. Sharma, A. Dua and A. Malik, Biocompatible stimuli responsive superabsorbent polymer for controlled release of GHK-Cu peptide for wound dressing application, J. Polym. Res., 2017, 24(7), 104 CrossRef.
- Y.-F. Li, S.-H. Ouyang, L.-F. Tu, X. Wang, W.-L. Yuan, G.-E. Wang, Y.-P. Wu, W.-J. Duan, H.-M. Yu, Z.-Z. Fang, H. Kurihara, Y. Zhang and R.-R. He, Caffeine Protects Skin from Oxidative Stress-Induced Senescence through the Activation of Autophagy, Theranostics, 2018, 8(20), 5713–5730 CrossRef CAS.
- W.-h Ma, M. Li, H.-f Ma, W. Li, L. Liu, Y. Yin, X.-m Zhou and G. Hou, Protective effects of GHK-Cu in bleomycin-induced pulmonary fibrosis via anti-oxidative stress and anti-inflammation pathways, Life Sci., 2020, 241, 117139 CrossRef CAS PubMed.
- L. Sun, A. Li, Y. Hu, Y. Li, L. Shang and L. Zhang, Self-Assembled Fluorescent and Antibacterial GHK-Cu Nanoparticles for Wound Healing Applications, Part. Part. Syst. Charact., 2019, 36(4), 1800420 CrossRef.
- P. Verma and D. G. Truhlar, Status and Challenges of Density Functional Theory, Trends Chem., 2020, 2(4), 302–318 CrossRef CAS.
- S. Lim, J.-W. Choi, D. J. Suh, K. H. Song, H. C. Ham and J.-M. Ha, Combined experimental and density functional theory (DFT) studies on the catalyst design for the oxidative coupling of methane, J. Catal., 2019, 375, 478–492 CrossRef CAS.
- X. Xu, L. Si, X. Zhou, F. Tu, X. Zhu and J. Bao, Chemical bonding between antimony and ionic liquid-derived nitrogen-doped carbon for sodium-ion battery anode, J. Power Sources, 2017, 349, 37–44 CrossRef CAS.
- X. Xu, Z. Dou, E. Gu, L. Si, X. Zhou and J. Bao, Uniformly-distributed Sb nanoparticles in ionic liquid-derived nitrogen-enriched carbon for highly reversible sodium storage, J. Mater. Chem. A, 2017, 5(26), 13411–13420 RSC.
- R. Mera-Adasme, M. C. Rezende and M. Domínguez, On the physical-chemical nature of solvent polarizability and dipolarity, Spectrochim. Acta, Part A, 2020, 229, 118008 CrossRef CAS PubMed.
- M. Gorcea, M. E. Lane and D. J. Moore, Exploratory in vivo biophysical studies of stratum corneum lipid organization in human face and arm skin, Int. J. Pharm., 2022, 622, 121887 CrossRef CAS PubMed.
- M. Gunnarsson, E. H. Mojumdar, D. Topgaard and E. Sparr, Extraction of natural moisturizing factor from the stratum corneum and its implication on skin molecular mobility, J. Colloid Interface Sci., 2021, 604, 480–491 CrossRef CAS PubMed.
- W. P. Raab, The skin surface and stratum corneum, Br. J. Dermatol., 1990, 122(s35), 37–41 CrossRef.
-
Skin to e-skin, Nat. Nanotechnol., 2017, 1211, 1017 Search PubMed.
- X. Li, W. Qiu, J. Li, X. Chen, Y. Hu, Y. Gao, D. Shi, X. Li, H. Lin, Z. Hu, G. Dong, C. Sheng, B. Jiang, C. Xia, C.-Y. Kim, Y. Guo and J. Li, First-generation species-selective chemical probes for fluorescence imaging of human senescence-associated β-galactosidase, Chem. Sci., 2020, 11(28), 7292–7301 RSC.
- C. Rouault, G. Marcelin, S. Adriouch, C. Rose, L. Genser, M. Ambrosini, J.-C. Bichet, Y. Zhang, F. Marquet, J. Aron-Wisnewsky, C. Poitou, S. André, G. Dérumeaux, M. Guerre-Millo and K. Clément, Senescence-associated β-galactosidase in subcutaneous adipose tissue associates with altered glycaemic status and truncal fat in severe obesity, Diabetologia, 2021, 64(1), 240–254 CrossRef CAS PubMed.
- X. Zhao, G. Zhu, L. Jiao, F. Yu and C. Xie, Formation and Extractive Desulfurization Mechanisms of Aromatic Acid Based Deep Eutectic Solvents: An Experimental and Theoretical Study, Chem. – Eur. J., 2018, 24(43), 11021–11032 CrossRef CAS.
Footnotes |
† Electronic supplementary information (ESI) available: Physical and chemical properties of ILs and related test experimental data. See DOI: https://doi.org/10.1039/d2nj04282g |
‡ Tianqi Liu and Lu Hu contributed equally to this work. |
|
This journal is © The Royal Society of Chemistry and the Centre National de la Recherche Scientifique 2023 |
Click here to see how this site uses Cookies. View our privacy policy here.