DOI:
10.1039/D2QI01830F
(Research Article)
Inorg. Chem. Front., 2023,
10, 184-191
Identifying the intrinsic active site in bimetallic Co3S4/Ni3S2 feathers on MXene nanosheets as a heterostructure for efficient oxygen evolution reaction†
Received
13th September 2022
, Accepted 8th November 2022
First published on 9th November 2022
Abstract
Exploring economical, efficient and stable electrocatalysts toward the oxygen evolution reaction (OER) is crucial for the advancement of water splitting to optimize sustainable energy conversion technologies. Herein, Co3S4/Ni3S2 feathers are fixed on MXene nanosheets supported by nickel foam as a NF@MXene@Co3S4/Ni3S2 heterogeneous electrocatalyst through hydrothermal reaction. The catalyst exhibits excellent OER performance in alkaline medium (1M KOH) with a low overpotential of 186 mV at the current density of 10 mA cm−2 and a small Tafel slope of 97 mV dec−1, showing long-term durability over 24 h and good stability after 1000 cyclic voltammetry (CV) cycles. Theoretical calculation further identified that the key intrinsic active catalytic site in this bimetallic catalyst is the nickel atom of Ni3S2, which enables adsorption of the intermediates, due to a lower Gibbs free energy change of the rate-determining step of *O to *OOH. The effective identification of the intrinsic catalytically active site in the heterostructure contributes to the regulation of composites and the development of electrocatalysis applications in the OER.
Introduction
Due to the increasingly severe issues of environmental pollution and energy shortages caused by the massive consumption of fossil fuels, numerous investigations have been devoted to developing clean, sustainable and renewable energy sources, among which electrochemical water splitting for hydrogen production is an ecologically friendly and economically viable solution.1–5 However, the oxygen evolution reaction (OER) suffers from sluggish kinetics involving four-electron-proton coupled transfer, compared with the two-electron-transfer hydrogen evolution reaction (HER), and it is regarded as the rate-determining step for water splitting.6–10 Accordingly, the design and synthesis of high-efficiency electrocatalysts for OER is essential to improve the conversion efficiency of water splitting.
Currently, precious metal catalysts such as RuO2 and IrO2 exhibit high catalytic activity for OER under alkaline conditions, but their exorbitant price and scarce abundance restrict their large-scale practical applications.11–15 Thus, developing an earth-abundant water oxidation catalyst with the properties of low-cost, effectiveness, high energy efficiency, and long-term durability is of great importance for improvement of the sluggish kinetics of OER.16,17 Hence, researchers have concentrated on the design of alternative OER catalysts based on 3d transition metals, for instance, forming mixed metal oxides,18,19 sulfides,20–22 selenides,23–25 nitrides,26–28 phosphides,29,30 their hybrids31–33 and so on. Among the above alternatives, transition metal sulfides (TMSs) with semiconductor properties and better conductivity can facilitate the charge transfer process,34 and are regarded as the most promising candidate to replace precious metals. Co3S4 and Ni3S2 often respectively emerge as ideal OER catalysts.35,36 However, when these two active TMSs are combined in bimetallic heterojunction materials, the real active site of Co3S4/Ni3S2 for OER has not been probed. Moreover, since TMSs suffer from the tendency of common agglomeration, resulting in poor durability and a reduced chemically active area,37,38 suitable support materials are required. The two-dimensional (2D) material MXene shows excellent electrocatalytic properties due to its high conductivity, elemental diversity and rich surface terminations, which can be introduced into a heterostructure employed as the substrate.39–41 Hao et al., grew CoFe-LDH (layered dihydroxide) vertically on the MXene substrate, effectively inhibiting the aggregation of CoFe-LDH, which exposed more active sites of the heterostructure and enhanced the efficiency of OER.42 In addition, Yu et al., verified that the interfacial interaction between FeNi-LDH and MXene gives rise to electronic coupling with the formation of prominent charge transfer.43 Inspired by these works, integrating TMSs with MXene to form a heterostructure is anticipated to produce enhanced performance OER electrocatalysts.
In this work, we vertically grew bimetallic Co3S4/Ni3S2 feathers on MXene nanosheets supported by nickel foam, forming a multidimensional feather structure, NF@MXene@Co3S4/Ni3S2, through a simple hydrothermal reaction. With the two precursors NF@MXene and NF@MXene@CoNi-LDH, and the control samples NF@MXene@Co3S4, NF@MXene@Ni3S2, NF@Co3S4/Ni3S2 for comparison, these heterostructures were investigated for their OER performance under alkaline conditions. The results demonstrate that the NF@MXene@Co3S4/Ni3S2 electrode can achieve an impressive overpotential of 186 mV at the current density of 10 mA cm−2 and a Tafel slope of 97 mV dec−1 in contrast to NF@MXene (260 mV and 147 mV dec−1) and NF@MXene@CoNi-LDH (236 mV and 122 mV dec−1). MXene prevents the Co3S4/Ni3S2 feathers from agglomeration and improves the conductivity of the catalyst, providing more numerous rich reactive sites compared to the control samples. Density functional theory (DFT) further revealed that the real intrinsic active sites of the bimetallic Co3S4/Ni3S2 feathers are mainly concentrated on the nickel atom of Ni3S2. This study contributes insight to the fabrication of heterostructures as more effective OER electrocatalysts.
Experimental
Preparation of Ti3C2Tx MXene
The delaminated Ti3C2Tx MXene was synthesized by etching Ti3AlC2 powder with HCl/LiF as previously reported.44–46 2 g of LiF was added into 50 mL of a 9 M HCl solution and stirred for 30 min at 300 rpm. Then, 2 g of Ti3AlC2 was slowly added into the solution and stirred for 30 h at 35 °C with a stirring rate of 100 rpm. After that, the mixture was washed repeatedly with deionized water, and centrifuged (5000 rpm, 5 min) until the pH of the supernatant was greater than 6. The precipitate was then re-dispersed in 100 mL of deionized water and sonicated with an ultrasound water bath (kept below 4 °C) at 500 W for 1 h under N2 flow, followed by centrifugation at 3500 rpm for 1 h to obtain the Ti3C2Tx colloidal solution. After drying, 1 mL of the Ti3C2Tx colloid was weighed to calculate its concentration, yielding 1.2 g, then configured into a 1 mg mL−1 solution.
Preparation of NF@MXene
To prepare NF@MXene, the nickel foam (NF, 1 × 2 cm2) was ultrasonically cleaned with hydrochloric acid (6 M), acetone, ethanol and deionized water, and dried in a vacuum for 6 h at 60 °C. The NF was added into 2 mg mL−1 CTAB for 30 min and immersed into 1 mg mL−1 MXene for 30 min, then dried in a vacuum for 6 h at 60 °C.
Preparation of NF@MXene@CoNi-LDH
In a typical procedure, 1.20 mmol of Co(NO3)2·6H2O and 0.40 mmol of Ni(NO3)2·6H2O were added into 20 mL of deionized water and sonicated for 10 min. Then, NF@MXene was immersed into the solution and aged for 1 h and transferred into a 50 mL Teflon autoclave. 3.33 mmol of urea and 20 mL of deionized water were added into the mixture and it was kept at 120 °C for 4 h to obtain the NF@MXene@CoNi-LDH precursor. The precursor was washed with deionized water and dried in a vacuum at 60 °C.
Preparation of NF@MXene@Co3S4/Ni3S2
The NF@MXene@CoNi-LDH precursor was hydrothermally vulcanized to prepare the product. In detail, the precursor with 0.62 mmol of Na2S·9H2O was added to 40 mL of deionized water and transferred into a 50 mL Teflon autoclave, which was kept at 160 °C for 12 h, washed with deionized water and dried in a vacuum at 60 °C to gain the NF@MXene@Co3S4/Ni3S2 product.
Results and discussion
The heterostructure NF@MXene@Co3S4/Ni3S2 was obtained via a simple hydrothermal reaction (Fig. 1). Scanning electron microscopy (SEM) was initially used to observe the morphology of the as-synthesized NF@MXene@Co3S4/Ni3S2 and its two precursors. As shown in Fig. 2a, MXene is uniformly dispersed over the surface of the NF, then, fuzz-like CoNi-LDH grows on the MXene after the hydrothermal process (Fig. 2b). During the final vulcanization reaction under hydrothermal conditions, TMS-Co3S4/Ni3S2 converts the fuzz to feathers, leading to the much rougher surface shown in Fig. 2c. Without MXene, the material NF@Co3S4/Ni3S2 agglomerates and forms irregular clusters (Fig. S1†). Transmission electron microscopy (TEM) imaging shows the great transparency of the Co3S4/Ni3S2 feather, reflecting its ultrathin feature (Fig. 2d). The energy dispersive spectroscopy (EDS) spectra confirmed the existence of C, O, S, Ti, Co and Ni elements, and the mapping images suggest a uniform dispersion of the six elements in NF@MXene@Co3S4/Ni3S2 (Fig. 2e). The high-resolution TEM (HRTEM) image reveals the layered property of this heterostructure with MXene-supported Co3S4/Ni3S2 (Fig. 2f), and the lattice fringes indicated the good crystalline character of Co3S4, Ni3S2 and MXene with lattice spacings of 0.550 nm, 0.287 nm and 0.256 nm, indexed to the (111), (110) and (110) crystal planes of Co3S4, Ni3S2 and MXene, respectively (Fig. 2g–i).
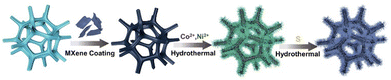 |
| Fig. 1 Schematic illustration of the preparation strategy of NF@MXene@Co3S4/Ni3S2. | |
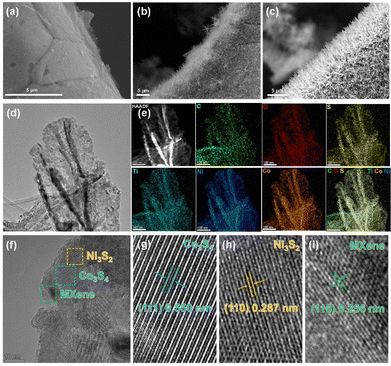 |
| Fig. 2 SEM images of (a) NF@MXene, (b) NF@MXene@CoNi-LDH and (c) NF@MXene@Co3S4/Ni3S2. (d) TEM image, (e) HAADF image and corresponding EDS elemental mapping of C, O, S, Ti, Ni, and Co and their overlay distribution of NF@MXene@Co3S4/Ni3S2. (f–i) HRTEM images of NF@MXene@Co3S4/Ni3S2. | |
The structure of NF@MXene@Co3S4/Ni3S2 was confirmed using powder X-ray diffraction (PXRD) with bare NF, MXene and NF@MXene as references. The weak peaks of MXene are covered by the strong signals of the NF pattern in the PXRD pattern of NF@MXene. New peaks were detected after the hydrothermal treatment of NF@MXene, located at 31.4°, 50.2°, and 55.1°, and 31.1°, 50.2°, 54.6°, and 55.2°, which respectively correspond to the Co3S4 (JCPDS No. 42-1448) and Ni3S2 (JCPDS No. 44-1418), evidencing the successful synthesis of the heterostructure NF@MXene@Co3S4/Ni3S2 (Fig. 3a). Moreover, the surface elemental composition and chemical state of the elements of NF@MXene@Co3S4/Ni3S2 were observed using X-ray photoelectron spectroscopy (XPS). The XPS survey spectrum indicates that NF@MXene@Co3S4/Ni3S2 consists of C, O, S, Ti, Co, and Ni elements (Fig. S2†), among which the elements C, O and Ti are derived from Ti3C2Tx MXene.47 In the Ti 2p XPS spectrum, four peaks centred at 464.0, 461.6, 458.3 and 455.6 eV can be observed, wherein the peaks at 455.6 and 461.6 eV are ascribed to the Ti–C bonds, and those at 458.3 and 464.0 eV can be assigned to the Ti–O bonds.(Fig. S3†)48–50 The XPS spectrum of S 2p contains spin–orbit doublet peaks at 162.1 eV (S 2p3/2) and 163.3 eV (S 2p1/2), and two shake-up satellite peaks at approximately 168.0 and 169.1 eV ascribed to the Sn2− and SOx species (Fig. 3b).44,51 The XPS spectrum of Co 2p consists of two pairs of spin–orbit doublets. The first doublet at 781.1 eV (Co 2p3/2) and 796.6 eV (Co 2p1/2) and the second doublet at 784.1 eV (Co 2p3/2) and 797.8 eV (Co 2p1/2) are attributed to Co2+ and Co3+ of Co3S4, respectively, while their accompanying satellite peaks are located at 791.6 and 802.6 eV (Fig. 3c).52–54 In the Ni 2p spectrum, two spin–orbit peaks locate at 856.1 eV (Ni2+ 2p3/2) and 873.6 eV (Ni2+ 2p1/2) along with two typical satellite peaks of Ni2+ at 861.4 and 879.4 eV, and the minor peak at 852.4 eV belongs to the metallic Ni of the NF substrate (Fig. 3d).37,55,56 The above analysis indicates that the composite NF@MXene@Co3S4/Ni3S2 was successfully manufactured.
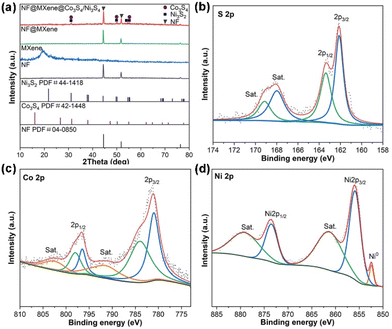 |
| Fig. 3 (a) PXRD patterns of NF, MXene, NF@MXene and NF@MXene@Co3S4/Ni3S2. High-resolution XPS spectra of (b) S 2p, (c) Co 2p and (d) Ni 2p regions of NF@MXene@Co3S4/Ni3S2. | |
In order to evaluate the effectiveness of the conductivity of the MXene nanosheets and the activity of the TMS-Co3S4/Ni3S2 feathers, the OER electrocatalytic performance of this heterostructure was appraised using a standard three-electrode cell in the potential range of 0.85–2.00 V versus the reversible hydrogen electrode (RHE) at a scan rate of 10 mV s−1 in 1.0 M KOH solution. In order to eliminate the influence of the nickel oxidation peak, the OER LSV polarization curves were obtained using the backward sweep, which can effectively evaluate the OER performance of as-synthesized catalysts (Fig. S4†).57 For comparison, a commercial RuO2 benchmark loaded on NF (denoted as NF@RuO2), the two precursors NF@MXene and NF@MXene@CoNi-LDH, and bare NF were also studied as control catalysts. Evidently, the featherlike NF@MXene@Co3S4/Ni3S2 catalyst exhibited superior electrocatalytic performance compared to the other electrodes. According to the linear sweep voltammetry (LSV) curves of the evaluated catalysts, NF@MXene@Co3S4/Ni3S2 can reach a current density of 10 mA cm−2 at a low overpotential (denoted as η10) of 186 mV (1.416 V vs. RHE), smaller than those of NF (429 mV), NF@MXene (260 mV), NF@MXene@CoNi-LDH (236 mV) and NF@RuO2 (399 mV) (Fig. 4a). Moreover, the samples exhibit the same trend for η100 as that of the LSV curves (Fig. 4b). Along with the MXene preventing Co3S4/Ni3S2 from agglomerating, the overpotential at a current density of 10 mA cm−2 of NF@MXene@Co3S4 (259 mV) with more active sites is smaller than that of NF@Co3S4/Ni3S2 (306 mV), but larger than that of NF@MXene@Ni3S2 (221 mV) with Ni3S2 instead of Co3S4 in NF@MXene@Co3S4 (Fig. S5†). The reaction kinetics of the electrocatalysts were evaluated using Tafel slopes with fitted linear regions. The Tafel slope of NF@MXene@Co3S4/Ni3S2 (97 mV dec−1) is smaller than those of NF (286 mV dec−1), NF@MXene (147 mV dec−1), NF@MXene@CoNi-LDH (122 mV dec−1) and NF@RuO2 (259 mV dec−1) (Fig. 4c), implying the fastest kinetics and the highest OER catalytic activity of NF@MXene@Co3S4/Ni3S2. The electrochemical active surface area (ECSA) of a catalyst is linearly proportional to the electrochemical double-layer capacitance (Cdl), which is estimated by measuring the non-faradaic potential range from the cyclic voltammetry (CV) curves at different scan rates (Fig. 4d and S6†). The calculated Cdl value of NF@MXene@Co3S4/Ni3S2 is 8.95 mF cm−2, much larger than those of NF (1.34 mF cm−2), NF@MXene (2.15 mF cm−2), NF@MXene@CoNi-LDH (3.30 mF cm−2) and NF@RuO2 (2.31 mF cm−2) (Fig. 4e), suggesting that the feather-like Co3S4/Ni3S2 fixed on the MXene nanosheets supported by nickel foam as a catalyst has a greater amount of catalytically active sites, contributing to better OER performance. Electrochemical impedance spectroscopy (EIS) tests were performed in a frequency range of 100 kHz-0.01 Hz under the open-circuit potential condition with an alternate current perturbation of 10 mV. A simplified equivalent circuit was used to fit the EIS data and to extract the series resistance (Rs), charge-transfer resistance (Rct) and constant phase elements (CPE), where Rs, Rct and CPE represent the series resistance, correspondingly accounting for the transport resistance, charge transfer resistance and electrode/electrolyte interface capacitance. The Nyquist plots further evidence the fastest electron transfer rate and accelerated faradaic process on the NF@MXene@Co3S4/Ni3S2 electrode, as shown in Fig. 4f.
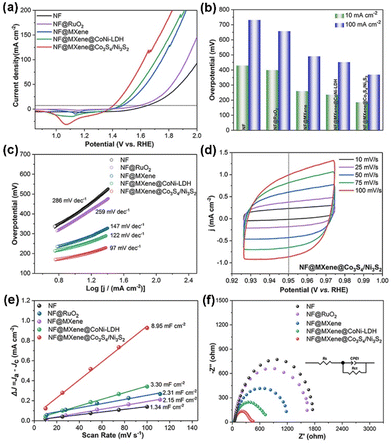 |
| Fig. 4 The OER performances of different catalysts in 1M KOH electrolyte. (a) LSV curves at 10 mV s−1, (b) overpotentials at 10 mA cm−2 and 100 mA cm−2, (c) Tafel slopes, (d) CV curves at non-faradaic potential, (e) Cdl values and (f) Nyquist plots. | |
Due to the Co3S4/Ni3S2 feathers fixed on the MXene nanosheets with varied active sites and good electrical conductivity, NF@MXene@Co3S4/Ni3S2 exhibits superior performance with a lower overpotential at 10 mA cm−2 compared with previously developed electrocatalysts (Fig. 5a, Table S1†). In addition, long-term stability is a vital parameter when investigating the electrochemical performance of an electrocatalyst. The long-term durability of NF@MXene@Co3S4/Ni3S2 was evaluated by continuous CV scanning and chronoamperometric tests. The OER curve almost completely overlaps the initial one after 1000 CV cycles (Fig. 5b) and the straight i-t curve proves that the current density remains stable with minimal decay for 24 h at a constant potential of 1.62 V, corroborating the electrocatalytic durability of this heterojunction structure (Fig. 5c). Further, the SEM image shows that NF@MXene@Co3S4/Ni3S2 still maintains its initial feather-like morphology, and the EDS elemental mapping confirmed the uniform distribution of C, O, S, Ti, Co and Ni after the OER stability test, the same as their initial one (Fig. 5d and e). In addition, the structure verified by PXRD and Brunauer–Emmett–Teller (BET) surface area of NF@MXene@Co3S4/Ni3S2 showed no obvious alteration after the stability test (Fig. S7 and S8, Table S2†), which again confirms the catalytic stability of this heterostructure for OER.
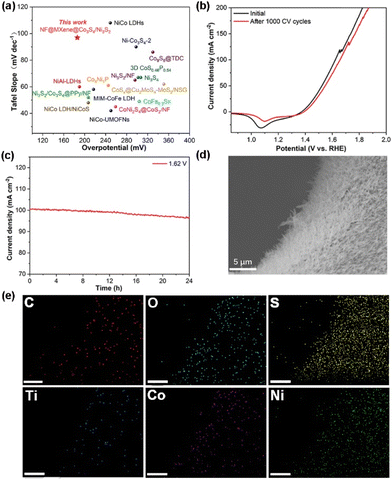 |
| Fig. 5 (a) Comparison of overpotentials at 10 mA cm−2 of different electrocatalysts in the literature. (b) The LSV curves of NF@MXene@Co3S4/Ni3S2 before and after 1000 CV cycles. (c) Chronoamperometry curve of the durability test of NF@MXene@Co3S4/Ni3S2. (d) SEM image and (e) EDS elemental mapping of NF@MXene@Co3S4/Ni3S2 after the stability test. | |
Density Functional Theory (DFT) calculation was performed to understand the mechanism of the OER activity of Co3S4 and Ni3S2 in the NF@MXene@Co3S4/Ni3S2 electrocatalyst to ascertain the actual active site in the heterostructure. The reaction coordinates during OER of Co3S4 and Ni3S2 are schematically depicted in Fig. 6a and b, respectively. The details of the optimized structures with adsorptive active intermediates are shown in Fig. S9–S12,† respectively. It was found that Co and Ni atoms behave as the main active sites for the adsorption of intermediates. According to the Gibbs free energy change of homologous active sites, we found that the conversion *O + OH− → *OOH + e− is the rate-determining step for both Co3S4 and Ni3S2 based catalysts synthesized in this work. The calculated free energy changes ΔG1, ΔG2, ΔG3 and ΔG4 for Co3S4 and Ni3S2 are shown in Table S3;† for the * + OH− → *OH + e− step, the Co3S4 group is more negative than the Ni3S2 group, which means that Co3S4 has a higher binding energy for OH. However, in the *OH + OH− → *O + H2O + e− step, Co3S4 needs an additional energy input of 0.16 eV (1.39–1.23 = 0.16 eV), while the value for Ni3S2 is 0 eV (1.23–1.23 = 0 eV), exactly equal to a standard hydrogen potential that does not require additional energy input. The next step, *O + OH− → *OOH + e−, further intensifies the additional energy input of Co3S4 (Fig. 6c and d). The highest energy input required for the overpotential of Co3S4 is 0.41 V, compared with 0.37 V for Ni3S2, which certifies that the nickel atom plays a pivotal role as the active site in this heterogeneous structure.
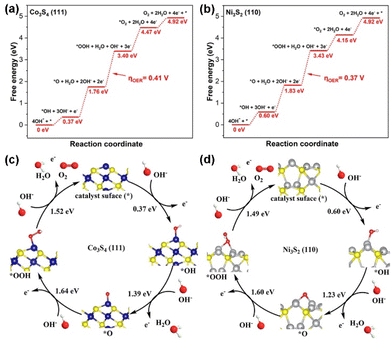 |
| Fig. 6 The optimized absorptive structures of (a) Co3S4 and (b) Ni3S2 at the four reaction coordinates during OER. Free energy diagram at 1.23 V for OER of (c) Co3S4 and (d) Ni3S2. | |
Conclusions
To sum up, a heterostructure, NF@MXene@Co3S4/Ni3S2, based on the homogeneous distribution of feathers supported by 2D nanosheets was successfully produced using a hydrothermal process. It shows excellent catalytic performance toward OER in an alkaline medium (1M KOH), manifesting a low overpotential of 186 mV at a current density of 10 mA cm−2 and a small Tafel slope of 97 mV dec−1 with good structural stability. Based on theoretical calculation, the nickel atom in NF@MXene@Co3S4/Ni3S2 performs as the key active site, giving a lower Gibbs free energy change from *O to *OOH. Consequently, the NF@MXene@Co3S4/Ni3S2 catalyst is a promising catalyst for OER and provides a new strategy for energy conversion.
Author contributions
Yang Li: conceptualization, writing – original draft, visualization, and data curation. Qixuan Du: writing – original draft and visualization. Jian Cui: data curation and visualization. Xiao Chen: conceptualization, data curation, and visualization. Hongwei Yang: validation, supervision, and resources. Hua Qian: supervision, writing – review & editing, and resources.
Conflicts of interest
There are no conflicts to declare.
Acknowledgements
We gratefully acknowledge the National Natural Science Foundation of China (No.22075143) and Hongwei Yang thanks the Qing Lan Project for the grant.
References
- H. Jiang, J. Gu, X. Zheng, M. Liu, X. Qiu, L. Wang, W. Li, Z. Chen, X. Ji and J. Li, Defect-rich and ultrathin N doped carbon nanosheets as advanced trifunctional metal-free electrocatalysts for the ORR, OER and HER, Energy Environ. Sci., 2019, 12, 322–333 RSC
.
- H. Yan, Y. Xie, A. Wu, Z. Cai, L. Wang, C. Tian, X. Zhang and H. Fu, Anion-modulated HER and OER activities of 3D Ni-V-based interstitial compound heterojunctions for high-efficiency and stable overall water splitting, Adv. Mater., 2019, 31, 1901174 CrossRef PubMed
.
- H. Wang and H. Dai, Strongly coupled inorganic-nano-carbon hybrid materials for energy storage, Chem. Soc. Rev., 2013, 42, 3088–3113 RSC
.
- L. Cifuentes, V. H. Borja-Aburto, N. Gouveia, G. Thurston and D. L. Davis, Hidden health benefits of greenhouse gas mitigation, Science, 2001, 293, 1257–1259 CrossRef CAS
.
- T. H. Shen, L. Spillane, J. Peng, Y. Shao-Horn and V. Tileli, Switchable wetting of oxygen-evolving oxide catalysts, Nat. Catal., 2022, 5, 30–36 CrossRef CAS
.
- J. S. Kim, B. Kim, H. Kim and K. Kang, Recent progress on multimetal oxide catalysts for the oxygen evolution reaction, Adv. Energy Mater., 2018, 8, 1702774 CrossRef
.
- J. Chen, P. Cui, G. Zhao, K. Rui, M. Lao, Y. Chen, X. Zheng, Y. Jiang, H. Pan, S. X. Dou and W. Sun, Low-coordinate iridium oxide confined on graphitic carbon nitride for highly efficient oxygen evolution, Angew. Chem., Int. Ed., 2019, 58, 12540–12544 CrossRef CAS PubMed
.
- K. Zhu, F. Shi, X. Zhu and W. Yang, The roles of oxygen vacancies in electrocatalytic oxygen evolution reaction, Nano Energy, 2020, 73, 104761 CrossRef CAS
.
- L. Huang, G. Gao, H. Zhang, J. Chen, Y. Fang and S. Dong, Self-dissociation-assembly of ultrathin metal-organic framework nanosheet arrays for efficient oxygen evolution, Nano Energy, 2020, 68, 104296 CrossRef CAS
.
- J. Du, F. Li and L. Sun, Metal-organic frameworks and their derivatives as electrocatalysts for the oxygen evolution reaction, Chem. Soc. Rev., 2021, 50, 2663–2695 RSC
.
- B. You and Y. Sun, Innovative strategies for electrocatalytic water splitting, Acc. Chem. Res., 2018, 51, 1571–1580 CrossRef CAS PubMed
.
- V. Petrykin, K. Macounova, O. A. Shlyakhtin and P. Krtil, Tailoring the selectivity for electrocatalytic oxygen evolution on ruthenium oxides by zinc substitution, Angew. Chem., Int. Ed., 2010, 49, 4813–4815 CrossRef CAS PubMed
.
- Y. Lee, J. Suntivich, K. J. May, E. E. Perry and Y. Shao-Horn, Synthesis and activities of rutile IrO2 and RuO2 nanoparticles for oxygen evolution in acid and alkaline solutions, J. Phys. Chem. Lett., 2012, 3, 399–404 CrossRef CAS PubMed
.
- S. Laha, Y. Lee, F. Podjaski, D. Weber, V. Duppel, L. M. Schoop, F. Pielnhofer, C. Scheurer, K. Müller, U. Starke, K. Reuter and B. V. Lotsch, Ruthenium oxide nanosheets for enhanced oxygen evolution catalysis in acidic medium, Adv. Energy Mater., 2019, 9, 1803795 CrossRef
.
- Z. Chen, X. Duan, W. Wei, S. Wang and B.-J. Ni, Iridium-based nanomaterials for electrochemical water splitting, Nano Energy, 2020, 78, 105270 CrossRef CAS
.
- Z. P. Wu, X. F. Lu, S. Q. Zang and X. W. Lou, Non-noble-metal-based electrocatalysts toward the oxygen evolution reaction, Adv. Funct. Mater., 2020, 30, 1910274 CrossRef CAS
.
- J. Zhang, Q. Zhang and X. Feng, Support and interface effects in water-splitting electrocatalysts, Adv. Mater., 2019, 31, 1808167 CrossRef
.
- F. Shi, K. Huang, Y. Wang, W. Zhang, L. Li, X. Wang and S. Feng, Black phosphorus-modified Co3O4 through tuning the electronic structure for enhanced oxygen evolution reaction, ACS Appl. Mater. Interfaces, 2019, 11, 17459–17466 CrossRef CAS PubMed
.
- T. Zhou, C. Wang, Y. Shi, Y. Liang, Y. Yu and B. Zhang, Temperature-regulated reversible transformation of spinel-to-oxyhydroxide active species for electrocatalytic water oxidation, J. Mater. Chem. A, 2020, 8, 1631–1635 RSC
.
- L. Song, X. Zhang, S. Zhu, Y. Xu and Y. Wang, Hydrogen spillover effect enhanced by carbon quantum dots activated MoS2, Carbon, 2022, 199, 63–69 CrossRef CAS
.
- S. Deng, Y. Shen, D. Xie, Y. Lu, X. Yu, L. Yang, X. Wang, X. Xia and J. Tu, Directional construction of Cu2S branch arrays for advanced oxygen evolution reaction, J. Energy Chem., 2019, 39, 61–67 CrossRef
.
- Y. Wang, Y. Zhou, M. Han, Y. Xi, H. You, X. Hao, Z. Li, J. Zhou, D. Song, D. Wang and F. Gao, Environmentally-friendly exfoliate and active site self-assembly: Thin 2D/2D heterostructure amorphous nickel-iron alloy on 2D materials for efficient oxygen evolution reaction, Small, 2019, 15, 1805435 CrossRef
.
- K. Harish, J. Balamurugan, T. T. Nguyen, N. H. Kim and J. H. Lee, Advanced interfacial engineering of oxygen-enriched FexSn1−xOSe nanostructures for efficient overall water splitting and flexible zinc-air batteries, Appl. Catal., B, 2022, 305, 120924 CrossRef CAS
.
- I. S. Kwon, I. H. Kwak, G. M. Zewdie, S. J. Lee, J. Y. Kim, S. J. Yoo, J. G. Kim, J. Park and H. S. Kang, WSe2−VSe2 alloyed nanosheets to enhance the catalytic performance of hydrogen evolution reaction, ACS Nano, 2022, 16, 12569–12579 CrossRef CAS PubMed
.
- Y. Shi, W. Du, W. Zhou, C. Wang, S. Lu, S. Lu and B. Zhang, Unveiling the promotion of surface-adsorbed chalcogenate on the electrocatalytic oxygen evolution reaction, Angew. Chem., Int. Ed., 2020, 59, 22470–22474 CrossRef CAS
.
- S. Zhao, M. Li, M. Han, D. Xu, J. Yang, Y. Lin, N.-E. Shi, Y. Lu, R. Yang, B. Liu, Z. Dai and J. Bao, Defect-rich Ni3FeN nanocrystals anchored on N-doped graphene for enhanced electrocatalytic oxygen evolution, Adv. Funct. Mater., 2018, 28, 1706018 CrossRef
.
- J. Huang, Y. Sun, X. Du, Y. Zhang, C. Wu, C. Yan, Y. Yan, G. Zou, W. Wu, R. Lu, Y. Li and J. Xiong, Cytomembrane-structure-inspired active Ni-N-O interface for enhanced oxygen evolution reaction, Adv. Mater., 2018, 30, 1803367 CrossRef PubMed
.
- C. Walter, P. W. Menezes, S. Orthmann, J. Schuch, P. Connor, B. Kaiser, M. Lerch and M. Driess, A molecular approach to manganese nitride acting as a high performance electrocatalyst in the oxygen evolution reaction, Angew. Chem., Int. Ed., 2018, 57, 698–702 CrossRef CAS PubMed
.
- J. Park, H. Yoon, D.-Y. Lee, S. G. Ji, W. Yang, S. D. Tilley, M.-C. Sung, I. J. Park, J. Tan, H. Lee, J. Y. Kim, D.-W. Kim and J. Moon, Photovoltaic powered solar hydrogen production coupled with waste SO2 valorization enabled by MoP electrocatalysts, Appl. Catal., B, 2022, 305, 121045 CrossRef CAS
.
- X. Ding, J. Yu, W. Huang, D. Chen, W. Lin and Z. Xie, Modulation of the interfacial charge density on Fe2P-CoP by coupling CeO2 for accelerating alkaline electrocatalytic hydrogen evolution reaction and overall water splitting, Chem. Eng. J., 2023, 451, 138550 CrossRef CAS
.
- Z. Li, S. Xin, Y. Zhang, Z. Zhang, C. Li, C. Li, R. Bao, J. Yi, M. Xu and J. Wang, Boosting elementary steps kinetics towards energetic alkaline hydrogen evolution via dual sites on phase-separated Ni-Cu-Mn/hydroxide, Chem. Eng. J., 2023, 451, 138540 CrossRef CAS
.
- D. Y. Chung, P. P. Lopes, P. F. B. D. Martins, H. He, T. Kawaguchi, P. Zapol, H. You, D. Tripkovic, D. Strmcnik, Y. Zhu, S. Seifert, S. Lee, V. R. Stamenkovic and N. M. Markovic, Dynamic stability of active sites in hydr(oxy) oxides for the oxygen evolution reaction, Nat. Energy, 2020, 5, 222–230 CrossRef
.
- H. Sun, C. W. Tung, Y. Qiu, W. Zhang, Q. Wang, Z. Li, J. Tang, H. C. Chen, C. Wang and H. M. Chen, Atomic metal-support interaction enables reconstruction-free dual-site electrocatalyst, J. Am. Chem. Soc., 2022, 144, 1174–1186 CrossRef CAS PubMed
.
- T. Song, H. Xue, J. Sun, N. Guo, J. Sun, Y. R. Hao and Q. Wang, Bimetallic doping engineering of Ni3S2 nanosheets originating from NiFe layered double hydroxide for efficient overall water splitting, Chem. Commun., 2022, 58, 9874–9877 RSC
.
- Z. Li, W. Xu, X. Yu, S. Yang, Y. Zhou, K. Zhou, Q. Wu, S. Ning, M. Luo, D. Zhao and N. Wang, Synergistic effect between 1D Co3S4/MoS2 hetero-structures to boost the performance for alkaline overall water splitting, Inorg. Chem. Front., 2022, 9, 2139–2149 RSC
.
- J. Liu, Y. Wang, Y. Liao, C. Wu, Y. Yan, H. Xie and Y. Chen, Heterostructured Ni3S2-Ni3P/NF as a bifunctional catalyst for overall urea-water electrolysis for hydrogen generation, ACS Appl. Mater. Interfaces, 2021, 13, 26948–26959 CrossRef CAS
.
- T. Ai, H. Wang, W. Bao, L. Feng, X. Zou, X. Wei, L. Ding, Z. Deng and B. Rao, Fe-V synergistic doping effect of hierarchical Ni3S2 oblate-nanorod arrays for efficient electrocatalytic oxygen evolution reaction, Chem. Eng. J., 2022, 450, 138358 CrossRef CAS
.
- G.-L. Li, S. Cao, Z.-F. Lu, X. Wang, Y. Yan and C. Hao, FePc nanoclusters modified NiCo layered double hydroxides in parallel with Ti3C2 MXene as a highly efficient and durable bifunctional oxygen electrocatalyst for zinc-air batteries, Appl. Surf. Sci., 2022, 591, 153142 CrossRef CAS
.
- H. Liao, X. Guo, P. Wan and G. Yu, Conductive MXene nanocomposite organohydrogel for flexible, healable, low-temperature tolerant strain sensors, Adv. Funct. Mater., 2019, 29, 1904507 CrossRef
.
- Y. Z. Zhang, J. K. El-Demellawi, Q. Jiang, G. Ge, H. Liang, K. Lee, X. Dong and H. N. Alshareef, MXene hydrogels: fundamentals and applications, Chem. Soc. Rev., 2020, 49, 7229–7251 RSC
.
- A. VahidMohammadi, J. Rosen and Y. Gogotsi, The world of two-dimensional carbides and nitrides (MXenes), Science, 2021, 372, 1165 CrossRef
.
- C. Hao, Y. Wu, Y. An, B. Cui, J. Lin, X. Li, D. Wang, M. Jiang, Z. Cheng and S. Hu, Interface-coupling of CoFe-LDH on MXene as high-performance oxygen evolution catalyst, Mater. Today Energy, 2019, 12, 453–462 CrossRef
.
- M. Yu, S. Zhou, Z. Wang, J. Zhao and J. Qiu, Boosting electrocatalytic oxygen evolution by synergistically coupling layered double hydroxide with MXene, Nano Energy, 2018, 44, 181–190 CrossRef CAS
.
- J. Fu, L. Li, J. M. Yun, D. Lee, B. K. Ryu and K. H. Kim, Two-dimensional titanium carbide (MXene)-wrapped sisal-like NiCo2S4 as positive electrode for high-performance hybrid pouch-type asymmetric supercapacitor, Chem. Eng. J., 2019, 375, 121939 CrossRef CAS
.
- Q. Jiang, N. Kurra, M. Alhabeb, Y. Gogotsi and H. N. Alshareef, All pseudocapacitive MXene-RuO2 asymmetric supercapacitors, Adv. Energy Mater., 2018, 8, 1703043 CrossRef
.
- H. Shin, W. Eom, K. H. Lee, W. Jeong, D. J. Kang and T. H. Han, Highly electroconductive and mechanically strong Ti3C2Tx MXene fibers using a deformable MXene gel, ACS Nano, 2021, 15, 3320–3329 CrossRef CAS
.
- S. Han, Y. Chen, Y. Hao, Y. Xie, D. Xie, Y. Chen, Y. Xiong, Z. He, F. Hu, L. Li, J. Zhu and S. Peng, Multi-dimensional hierarchical CoS2@MXene as trifunctional electrocatalysts for zinc-air batteries and overall water splitting, Sci. China Mater., 2020, 64, 1127–1138 CrossRef
.
- D. D. Khumujam, T. Kshetri, T. I. Singh, N. H. Kim and J. H. Lee, Fibrous asymmetric supercapacitor based on wet spun MXene/PAN fiber-derived multichannel porous MXene/CF negatrode and NiCo2S4 electrodeposited MXene/CF positrode, Chem. Eng. J., 2022, 449, 137732 CrossRef CAS
.
- C. F. Du, X. Sun, H. Yu, Q. Liang, K. N. Dinh, Y. Zheng, Y. Luo, Z. Wang and Q. Yan, Synergy of Nb doping and surface alloy enhanced on water-alkali electrocatalytic hydrogen generation performance in Ti-based MXene, Adv. Sci., 2019, 6, 1900116 CrossRef
.
- B. Ji, S. Fan, X. Ma, K. Hu, L. Wang, C. Luan, J. Deng, L. Cheng and L. Zhang, Electromagnetic shielding behavior of heat-treated Ti3C2TX MXene accompanied by structural and phase changes, Carbon, 2020, 165, 150–162 CrossRef CAS
.
- Y. Gao, J. Li, H. Gong, C. Zhang, H. Fan, X. Xie, X. Huang, H. Xue, T. Wang and J. He, The identified intrinsic active sites for efficient and.stable bi-functional catalyst N-MoS2.Ni3S2/NiS: the Mo-N structure and Ni-S structure on the heterogeneous interface synergistically enhance water splitting, J. Mater. Chem. A, 2022, 10, 11755–11765 RSC
.
- C. Liang, X. Zhang, P. Feng, H. Chai and Y. Huang, ZIF-67 derived hollow cobalt sulfide as superior adsorbent for effective adsorption removal of ciprofloxacin antibiotics, Chem. Eng. J., 2018, 344, 95–104 CrossRef CAS
.
- Z. Li, W. Lv, G. Wu, X. Li, X. Wang and W. Zhang, Multi-type cubic ComXn (X = O, S, Se) induced by zeolitic imidazolate framework (ZIF) as cathode materials for aluminum battery, Chem. Eng. J., 2022, 430, 133135 CrossRef CAS
.
- H. Wang, J. Xu, Q. Zhang, S. Hu, W. Zhou, H. Liu and X. Wang, Super-hybrid transition metal sulfide nanoarrays of Co3S4 nanosheet/P-doped WS2 nanosheet/Co9S8 nanoparticle with Pt-like activities for robust all-pH hydrogen evolution, Adv. Funct. Mater., 2022, 32, 211362 Search PubMed
.
- Z. Wang, S. Shen, Z. Lin, W. Tao, Q. Zhang, F. Meng, L. Gu and W. Zhong, Regulating the local spin state and band structure in Ni3S2 nanosheet for improved oxygen evolution activity, Adv. Funct. Mater., 2022, 32, 2112832 CrossRef CAS
.
- Z. Wu, Y. Feng, Z. Qin, X. Han, X. Zheng, Y. Deng and W. Hu, Bimetallic multi-level layered Co-NiOOH/Ni3S2@NF nanosheet for hydrogen evolution reaction in alkaline medium, Small, 2022, 2106904 CrossRef CAS
.
- J. Wang, M. Zhang, G. Yang, W. Song, W. Zhong, X. Wang, M. Wang, T. Sun and Y. Tang, Heterogeneous Bimetallic Mo-NiPx/NiSy as a Highly Efficient Electrocatalyst for Robust Overall Water Splitting, Adv. Funct. Mater., 2021, 31, 2101532 CrossRef CAS
.
Footnote |
† Electronic supplementary information (ESI) available: Additional electrochemistry, characterization and computation data, Fig. S1–S12 and Tables S1–S3. See DOI: https://doi.org/10.1039/d2qi01830f |
|
This journal is © the Partner Organisations 2023 |
Click here to see how this site uses Cookies. View our privacy policy here.