DOI:
10.1039/D2QI02080G
(Research Article)
Inorg. Chem. Front., 2023,
10, 67-77
Interlayer engineering of a V2O5 anode toward high-rate and durable dual-ion batteries†
Received
28th September 2022
, Accepted 8th November 2022
First published on 9th November 2022
Abstract
Dual-ion batteries (DIBs) have gained widespread attention thanks to their high operating voltage, low cost, and environmental friendliness. However, the development of DIBs is dramatically limited by the unsatisfactory rate capacity and cycling performance of anode materials. Herein, we adopted a water-incorporation approach to design a V2O5 anode with an extended interlayer gap (W-V2O5). The incorporated H2O not only offered ample space and abundant channels for efficient ion/electron intercalation/diffusion, but also improved structural stability during the repeated charge and discharge processes. Consequently, the W-V2O5 electrode delivered an enhanced specific capacity (293.2 vs. 264.4 mA h g−1 at 1C), rate property (48.2% vs. 24.6% from 1C to 70C), and cycling stability (96.8% vs. 74.6% over 1000 cycles). Furthermore, a DIBs device with fast kinetics was assembled with a graphite cathode and W-V2O5 NS anode, which could work normally in a wide range of current densities. This work opens a new avenue for designing layered materials for energy-storage devices with high rate and good durability.
Introduction
With the deepening of contradiction between environmental problems and energy demand, there is an urgent need to develop advanced rechargeable devices with cost-effectiveness, superior performance, and green environmental performance.1 Lithium-ion batteries (LIBs) have the advantages of large energy density, small self-discharge, long service life, memoryless effects, etc., and they have monopolized the electric vehicle and smart electronics markets.2 However, in practice, the shortage of lithium resources has made the manufacturing costs soar,3,4 while the preparation process of LIBs also causes pollution and damage to the environment;5,6 thus, there is an urgently need to develop novel substitutes that have high quality and are inexpensive.7,8 Alternatively, dual-ion batteries (DIBs, based on the working mechanism involving the storage of cations and anions separately in the anode and cathode during the charge/discharge process) represent a potential new energy-storage technology in the post-LIBs era, which brings the advantages of a high operating voltage, good safety, low cost, and environmental friendliness.9,10 Graphitic carbon is the preferred cathode for DIBs because of its large capacity, high reaction potential, good stability, low price, and environmental friendliness, which are conducive properties for constructing DIBs with large energy density, high power density, and good durability.10 Several new DIB devices have been reported, consisting of a graphite cathode and battery-type anode (including graphite,11,12 Co3O4,13 Ni3S2,14 MnO,15 KNi0.1Co0.9F3,16 Al metal,17 Sn metal,18 WS2,19 and MoS2
20). Unfortunately, the power density and durability of DIBs devices have thus far remained unsatisfactory. Therefore, it is a huge challenge to design an advanced anode that can be assembled with graphite cathodes into high-performance DIB devices.
Orthorhombic vanadium pentoxide (α-V2O5) with a layered structure was constructed by edge- and corner-sharing highly distorted [VO6] octahedrons and V2O5 sheets were held together via weak vanadium–oxygen interactions (van der Waals forces).21,22 Because of its natural abundance, good safety, multiple oxidation states, and environmental friendliness, α-V2O5 is a cation-intercalation anode material with great development potential for DIBs.23,24 The V5+/V4+ and V4+/V3+ redox couples are present in the Li+-intercalation reaction of α-V2O5, leading to a high theoretical capacity of 294 mA h g−1 for the insertion of two lithium ions.25 Moreover, the potential of α-V2O5 for intercalation is about 1.5–4.0 V vs. Li/Li+, which is smaller than that of graphite (>4.5 V vs. Li/Li+). This fully demonstrates that the α-V2O5 anode and graphite cathode can be assembled into a DIB device. Nevertheless, the further development and application of α-V2O5 suffer from its weak electrical conductivity (10−2 to 10−3 S cm−1) and low ionic diffusion coefficient (10−12 to 10−13 cm2 s−1), which can lead to an inferior rate capability and cycling stability performance.26–28 To overcome the above intrinsic drawbacks, many strategies have been applied, such as nanostructuring,29 cation/molecule pillaring,30 structural modification,26 and oxygen defects.31 Nevertheless, the reported α-V2O5 electrodes delivered unsatisfactory energy-storage performance due to limited kinetics and lifespan. Thus, it is incredibly challenging to exploit advanced α-V2O5 electrodes to achieve high stability and rate performance.
Herein, we introduce H2O molecules between the van der Waals layers of α-V2O5 nanosheets (NS) to extend the interlayer gap, and this sample was denoted as W-V2O5 NS. The introduced H2O molecules were able to occupy the sites of the lattice oxygen, and served as interlayer pillars to stabilize the structure. Furthermore, the ionic channel dimension was also enlarged as the a (lattice parameter) of W-V2O5 NS (13.12 Å) was larger than that of α-V2O5 NS (11.516 Å). This is favorable for the rapid diffusion of ions and the fast conduction of electrons. Therefore, the W-V2O5 NS electrode exhibited an improved high specific capacity (293.2 vs. 264.4 mA h g−1 at 1C), rate capability (8.2% vs. 24.6% from 1 to 70C), and cycling life (96.8% vs. 74.6% over 1000 cycles). Finally, a DIBs device was successfully assembled with the graphite cathode and W-V2O5 NS anode. The W-V2O5//graphite DIEs device could be operated well in the voltage range of 1.0–3.3 V with a short discharge time (0.1–2.5 h). Attributed to the fast kinetics and stable structure in the cycle process, the W-V2O5//graphite DIES device delivered a large energy density (481 W h kg−1) and decent power capability (3.08 kW kg−1) with good durability.
Experimental
Synthesis of α-V2O5 and W-V2O5 NS
In a typical procedure, vanadium oxytriisopropoxide (VOT, 1.0 mL) was first added into isopropanol (IPA, 40 mL) with mild stirring at room temperature. Then, commercial VO(acac)2 powders (2.65 g) was dissolved into the above mixed solution, which was kept stirring until a uniform solution was formed. The prepared reaction solution and a piece of clean carbon cloth (2 cm × 3 cm) were placed in a Teflon-lined stainless-steel autoclave (50 mL), which was heated to 180 °C and the temperature was kept constant for 10 h in an oven. The as-synthesized sample was then rinsed thoroughly with de-ionized water at room temperature. After annealing at 300 °C for 5 h in air, we eventually obtained the α-V2O5 NS. To gain the W-V2O5 NS, the α-V2O5 NS sample was wholly immersed in 0.8 M n-butyl lithium in hexane for 24 h in a glove box in an Ar atmosphere. Finally, the obtained sample was thoroughly rinsed with de-ionized water, and then left to dry naturally in the atmosphere.
Electrochemical characterization
A CR2032 coin cell was used to measure the electrochemical performances of all the half-cells. The free-standing α-V2O5 and W-V2O5 NS were grown on carbon cloth to directly serve as electrodes (1.3 mg cm−2). The graphite electrode was made from the active material, binder, and super P carbon black. First, the active material, binder, and super P carbon black were mixed uniformly in a weight ratio of 8
:
1
:
1. Second, the above mixture was evenly coated on an Al foil conductive substrate. Finally, the sample was put into a vacuum drying oven and heated to 80 °C for 12 h (1.7 mg cm−2). This coin half-cells system comprised a separator (Celgard 2400), counter and reference electrode (Li metal foil), and electrolyte (2 M LiPF6 dissolved in ethyl methyl carbonate).
W-V2O5//graphite DIES device tests were also conducted with CR2032 coin cells. In this DIES device, the graphite electrode acted as the cathode, and the W-V2O5 NS electrode was the anode. The mass ratio of graphite and W-V2O5 NS was about 2.5
:
1. The battery assembly was completed in a glove box filled with Ar. The concentration of H2O and O2 in the glove box was strictly controlled below 0.1 ppm.
A CHI 760E electrochemical workstation was used to collect the electrochemical performance data, including cyclic voltammetry (CV) curves, galvanostatic charge–discharge (GCD) profiles, Mott–Schottky plots, electrochemical impedance spectroscopy (EIS) spectra, and galvanostatic intermittent titration technique (GITT) curves.
Material characterization
Transmission electron microscopy (TEM, FEI Tecnai G2 F30) and field-emission scanning electron microscopy (FE-SEM, Hitachi S-4800) were used to analyze the microscopic topography. X-Ray diffraction (XRD, Bruker D8) was used to study the crystal structure. Raman spectroscopy, thermogravimetric analysis (TGA), and Fourier transform infrared spectroscopy (FTIR) were conducted on Renishaw inVia, Netzsch DSC-204 F1, and Thermo Nicolet 670 systems, respectively. The structural properties of the surrounding unpaired electrons were explored by electron paramagnetic resonance (EPR, A300-10–12 Bruker). An ASAP 2020 PLUS HD88 instrument was applied to obtain the N2 adsorption/desorption isotherms. The elemental composition and surface electronic state were investigated by X-ray photoelectron spectroscopy (XPS), synchrotron-based X-ray absorption near-edge spectroscopy (XANES), extended X-ray absorption fine structure (EXAFS) analysis, and ultraviolet photoemission spectroscopy (UPS), which were performed on the Photoemission Endstation (BL10B beamline).
Results and discussion
Fig. 1a illustrates the technology for extending the interlayer gaps of the α-V2O5 NS. First, the α-V2O5 NS was grown on carbon fiber cloth (Fig. S1†) through a hydrothermal method and annealing treatment. Then the sample was completely immersed in 0.8 M n-butyl lithium in hexane for 24 h, which could reduce the V species as a result of lithium-ion intercalation.31,32 Finally, the sample was transferred to water. In this step, the sample is oxidized caused by the Li+ extraction, and the hydrogen ions in the water are reduced to hydrogen gas simultaneously. Through such a redox process, H2O molecules can successfully occupy the positions of the lattice oxygen, thereby expanding the interlayer gaps in α-V2O5 NS. Compared to the original sample (Fig. S2a†), the W-V2O5 NS still retained the nanosheet array structure with a rougher surface that was caused by the mechanical strains from the expansion of the interlayer space (Fig. 1b). For more details about the microstructure, the W-V2O5 NS was characterized by TEM. The morphology of the W-V2O5 was a nanosheet with a rough surface, as noted in Fig. 1c. The surface of the nanosheets had many tiny pores, which increased the specific surface area of the W-V2O5 NS, as confirmed by the BET surface area measurements (Fig. S3†). The distinct diffraction rings in the selected area electron diffraction (SAED) pattern mean that the W-V2O5 NS is polycrystalline (insert in Fig. 1c). We could accurately identify these diffraction rings as the (200), (001), (101), and (111) planes of the orthorhombic V2O5 (JCPDS 41-1426). Specifically, interlayer gap belonging to the W-V2O5 NS was determined to be 0.656 nm (Fig. 1d), which was much larger than that of the original α-V2O5 NS (0.576 nm, Fig. S2c†), confirming that the incorporated H2O can expand the interlayer gaps of α-V2O5 NS. Additionally, the V, O, and C elements were uniformly distributed throughout the W-V2O5 NS, as confirmed by the energy dispersive spectroscopy (EDS) results in Fig. 1e–g. The crystal structure evolution along with the interlayer gap expansion could be probed through the XRD analyses. All the characteristic peaks of α-V2O5 NS in Fig. 2a matched well with the orthorhombic V2O5 (JCPDS 41-1426), and its lattice parameters a, b, and c were 11.516, 3.5656, and 4.3727 Å, respectively. No other impurity peaks were detected, indicating that the α-V2O5 NS was of very high purity. In the case of the W-V2O5 NS, similar diffraction peaks were maintained but shifted to lower angles. Specifically, the (200) peak shifted from 15.3° to 14.4°, indicating that the interlayer distance was expanded from 0.576 to 0.656 nm, which was in accordance with the HRTEM results. The interlayer distance of W-V2O5 NS was enlarged by 0.08 nm, much larger than that caused by oxygen defects,31 indicating the incorporated H2O had a better effect on expanding the interlayer gap compared to the oxygen defect. The widened interlayer distance would effectively promote Li+ insertion and extraction, which can be highly beneficial for boosting the lithium-ion storage performance.9 Moreover, the chemical bonding information of α-V2O5 and W-V2O5 NS could be found in the FTIR spectra (Fig. S4†). For the α-V2O5 NS, three characteristic peaks were observed, which were located at 1018 cm−1 (V
O stretching vibration), 835 cm−1 (V–O–V symmetric stretching), and 531 cm−1 (V–O–V asymmetric stretching).33,34 Obviously, the V–O–V bonds exhibited a blue-shift in W-V2O5 NS due to the stress variation and lattice distortion. Moreover, the presence of V
O at 990 cm−1 for the W-V2O5 NS indicated the reduction state (V4+) of vanadium. Additionally, two characteristic peaks attributed to O–H stretching vibration were observed at 3450 and 1622 cm−1, indicating the successful incorporation of H2O molecules between the van der Waals interlayers. As shown in Fig. S5,† all the Raman peaks of α-V2O5 NS were well consistent with the layered structure of orthorhombic V2O5
35,36 (Table S1†). Clearly, after incorporating H2O molecules, the Raman peaks became weaker and broader, and some of the peaks were shifted to lower wave numbers and even disappeared. These results confirmed that the incorporation of H2O could disturb the crystal arrangement of the [VO6] octahedrons and form oxygen defects in the W-V2O5 NS.
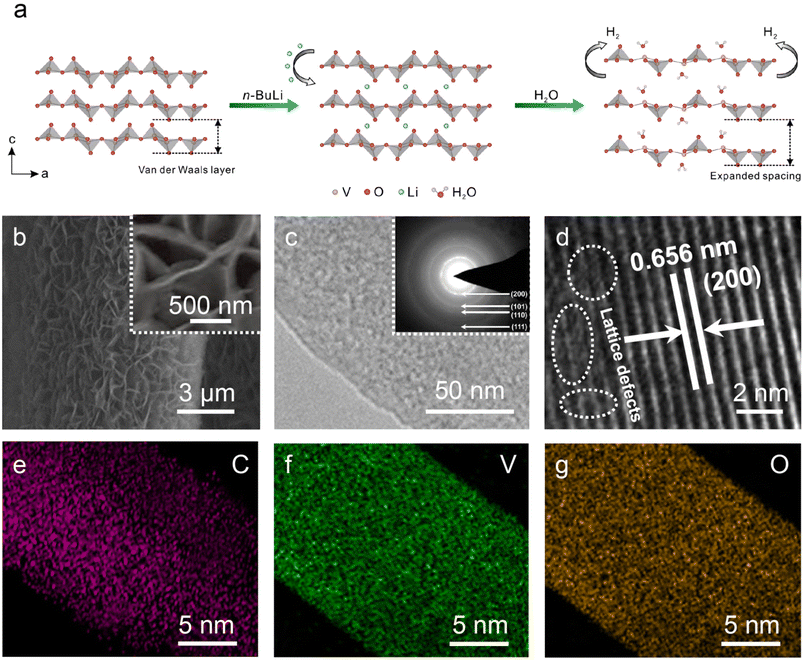 |
| Fig. 1 (a) Diagram of the process for extending the interlayer gap. (b) SEM image; inset is a magnification of the SEM image, (c) TEM image; inset is the SAED pattern, (d) high-resolution TEM image (HRTEM), and (e–g) C, V, and O elements mapping for W-V2O5 NS. | |
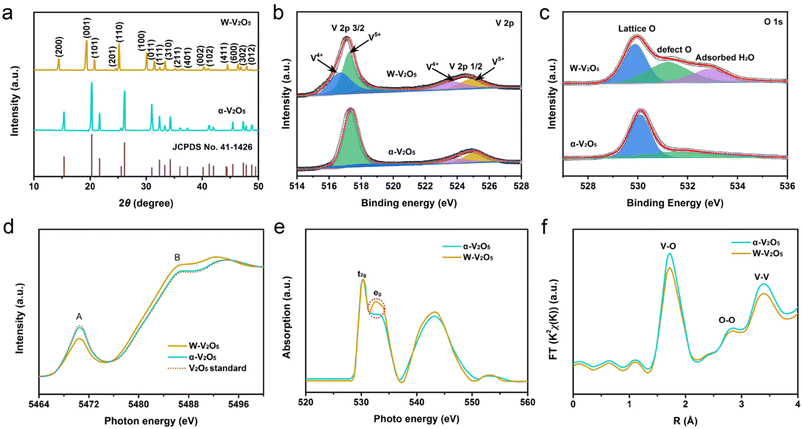 |
| Fig. 2 (a) XRD patterns; (b) V 2p and (c) O 1s spectra; (d) V K-edge and (e) O K-edge XANES spectra; and (f) radial distribution functions for α-V2O5 and W-V2O5 NS. | |
XPS was further carried out to ascertain the chemical composition and valence state in α-V2O5 and W-V2O5 NS. The XPS survey spectra in Fig. S6a† reveal that V, O, and C elements coexisted in both samples. Importantly, the Li 1s signal could not be detected for W-V2O5 NS (Fig. S6b†), indicating there was no Li-containing compound in the W-V2O5 NS, and excluding the possibility for prelithiation. The V 2p spectra of the α-V2O5 and W-V2O5 NS are displayed in Fig. 2b. Two peaks appeared at 517 and 525 eV, corresponding to the spin–orbit of V 2p3/2 and V 2p1/2, respectively, which could be divided into two peaks of V5+ and V4+.35,37 After the incorporation of H2O molecules, the intensities of the V4+ peaks increased significantly, while the intensities of the V5+ peaks decreased obviously, which was ascribed to the partial reduction from V5+ to V4+ in the process of the H2O molecules incorporation. Meanwhile, the V5+ peak of W-V2O5 NS was shifted to a lower binding energy, which also proved the existence of oxygen vacancies.31 Based on the peak areas of W-V2O5 NS, the ratio between V4+ and V5+ was calculated to be 1
:
1.5, giving an average oxidation state of +4.6. The O 1s spectra of α-V2O5 and W-V2O5 NS are compared in Fig. 2c. For both samples, the peak of lattice oxygen was located at 529.9 eV, while the peak at 531.2 eV was assigned to the oxygen defect with a low oxygen coordination.38 Notably, the defect oxygen peak for W-V2O5 NS was much stronger compared with α-V2O5 NS, indicating that water molecules were successfully incorporated into the W-V2O5 NS. Additionally, the W-V2O5 NS manifested a peak for surface adsorbed water located at 532.8 eV, which facilitated improved wettability and reactivity.39 Moreover, the oxygen defects in W-V2O5 NS could be confirmed again by the EPR spectra in Fig. S7,† which displayed an intensive peak at g = 2.0 for the W-V2O5 NS. As demonstrated by the Mott–Schottky plots in Fig. S8,† the oxygen vacancies in the W-V2O5 NS could effectively enhance the electrical conductivity. Moreover, the incorporated H2O molecules in W-V2O5 NS could be further verified by the TGA curves (Fig. S9†). Based on a weight loss of 0.79 wt%, the formula of W-V2O5 was determined as V2O4.92·0.08H2O. Combined with the TGA and XPS analysis results, the molar amounts of incorporated H2O and oxygen defects were approximately equal. Consequently, the introduced H2O molecules could substitute for the lattice oxygen in W-V2O5 NS, serve as interlayer pillars to stabilize the structure, and affect the charge-shielding effect by reducing the interaction with the surrounding framework.40
Furthermore, XANES measurements were performed to evaluate the valence state and structural environment change of V in α-V2O5 and W-V2O5 NS. A small pre-edge peak (A) was detected in the V K-edge XANES spectra, representing the electronic dipole-forbidden transition from the 1s to 3d orbital (Fig. 2d). However, when the local symmetry of the vanadium–oxygen coordination is structurally distorted, resulting in the hybridization of p–d orbitals, the above-mentioned electron transfer will be permitted in this situation. Simultaneously, a main absorption edge peak (B) also appeared in Fig. 2d, which was associated with the dipole-allowed electron transition from the 1s to 4p orbital. It is worth noting that the change in chemical coordination had an important influence on the intensity of the pre-edge peak, whose position was closely related to the oxidation state of V. In comparison with α-V2O5 NS, the absorption edge of W-V2O5 NS moved to a lower photon energy, which suggested a higher electron density around the V sites.1,40 Additionally, the intensity of the pre-edge peak was much weaker than that of α-V2O5 NS. This proved that the local asymmetry around V was reduced, which was due to the interaction between the incorporated H2O and terminal O in [VO6].41 This result was also verified by the V L edges XANES spectra in Fig. S10.† The V LIII edges of the W-V2O5 NS were shifted to lower energy compared to the α-V2O5 NS, suggesting a higher electron density.42 The O K-edge XANES spectra were further obtained for the two samples and are shown in Fig. 2e. The energy region of 528–537 eV referred to the hybridization of O 2p with V 4d states, which was split into t2g and eg peaks. Evidently, the W-V2O5 NS exhibited a much stronger eg peak compared with α-V2O5 NS, which indicated a more electron-rich state at the V sites caused by oxygen vacancies.43 Besides, the UPS spectra of α-V2O5 and W-V2O5 NS are depicted in Fig. S11.† It is clear that the center of the d-band of W-V2O5 NS was closer to the Fermi level (EF). This indicated that the introduced H2O molecules had a forceful interaction with the terminal O of [VO6], which would have a great influence on the valence band structure.44 The R space curves obtained from k2χ(k) by FT for EXAFS are illustrated in Fig. 2f, and provide data for further exploring the bonding and coordination. Here, V–O, O–O, and V–V bonds in [VO6] were detected for the α-V2O5 and W-V2O5 NS, located at 1.72, 2.86, and 3.01 Å, respectively.45 Notice that the curve characteristics of both samples were highly similar, which confirmed that the H2O incorporation did not cause crystal phase transformation again. Additionally, the FT peaks of W-V2O5 NS were all weaker than those of α-V2O5 NS, which implied that W-V2O5 NS had an increased disorder (or decreased coordination number).46 As a consequence, the water incorporation would be able to enhance the electron density localization around the V atoms effectively.
To evaluate the advantages of the expanded interlayer gap, the α-V2O5 and W-V2O5 NS were directly used as cathodes for half-cell electrochemical performance testing. When the battery is deeply discharged, an irreversible ω-phase LixV2O5 (2 < x < 3) will be formed, which would be detrimental for cycle life. Thus, in order to prevent the above situation, we selected an electrochemical working voltage between 2.0 and 4.0 V (vs. Li/Li+). The CV curves of α-V2O5 and W-V2O5 NS are shown in Fig. 3a, which were collected at 0.2 mV s−1 for the 3rd cycle. Compared with the α-V2O5 NS, the CV curve of W-V2O5 NS exhibited a greater current density and area, suggesting that W-V2O5 NS had better kinetics and capacity.47 Three pairs of redox peaks were observed in both electrodes, representing a three-step intercalation and intercalation process. During the cathodic process, the sample underwent the following transformations: α-V2O5 → ε-Li0.5V2O5 (3.35 V), ε-Li0.5V2O5 → δ-LiV2O5 (3.35 V), and δ-LiV2O5 → γ-Li2V2O5 (3.35 V).48 Conversely, the sample went through a reversible conversion in the anodic process: γ-LiV2O5 → δ-Li2V2O5 (2.60 V), δ-Li2V2O5 → ε-Li0.5V2O5 (3.27 V), and ε-Li0.5V2O5 → α-V2O5 (3.46 V).26 Moreover, compared with the α-V2O5 NS, the first five CV curves of the W-V2O5 NS electrode overlapped much more (Fig. S12†), signifying the good reversibility and durability. The electrochemical reactions for both electrodes during charging and discharging can be represented as follows:26
| V2O5 + 0.5Li+ + 0.5e− ⇌ ε-Li0.5V2O5 | (1) |
| ε-Li0.5V2O5 + 0.5Li+ + 0.5e− ⇌ δ-LiV2O5 | (2) |
| δ-LiV2O5 + Li+ + e− ⇌ γ-Li2V2O5 | (3) |
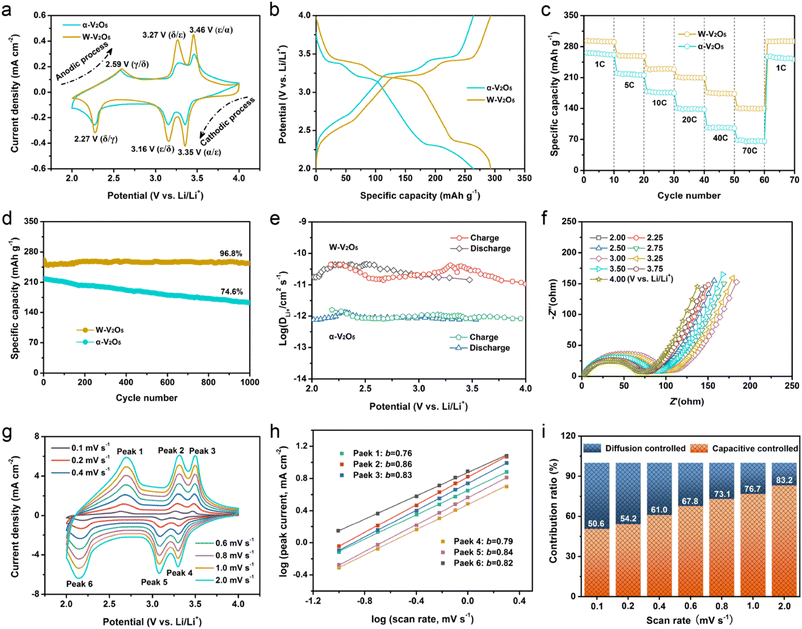 |
| Fig. 3 (a) CV curves, (b) GCD curves, (c) rate performance, (d) cycling performance, and (e) Li+ diffusion coefficient of the α-V2O5 and W-V2O5 NS electrodes. (f) Nyquist plots at different potentials, (g) CV curves, (h) log(i) vs. log(v) plots, and (i) capacitive contribution ratio for the W-V2O5 NS electrode. | |
Furthermore, the improved capacity for the W-V2O5 NS electrode could be further demonstrated with the GCD curves, which were measured at 1C (294 mA g−1) and are depicted in Fig. 3b. Each charge or discharge curve had three voltage platforms, and each voltage platform corresponded to an electrochemical reaction, which agreed with the results of the CV curves. Compared with the α-V2O5-NS electrode, the W-V2O5 NS electrode not only manifested a higher discharge voltage plateau, but also a lower charge voltage plateau. Additionally, the W-V2O5 NS electrode released a large capacity of 293 mA h g−1, which was very near to the theoretical capacity of 294 mA h g−1. Such a value was better than for the α-V2O5 NS electrode (268 mA h g−1) and a recently reported cathode material (Table S2†).31,49–52Fig. 3c shows the high-rate capabilities for α-V2O5 and W-V2O5 NS electrodes, which are important for developing high-performance LIBs, while the representative charge and discharge curves are displayed in Fig. S13.† When the current density was changed from 1C to 70C, a capacity retention of up to 48.2% was achieved by the W-V2O5 NS electrode. This value was superior to the α-V2O5 NS electrode (24.6% capacity retention) and the-state-of-art V2O5-based electrode presented in Table S2.† In addition, the W-V2O5 NS electrode displayed better cycle durability than the α-V2O5 NS electrode (Fig. 3d), whereby the W-V2O5 NS electrode could still maintain a large capacity of up to 253.7 mA h g−1 (96.8% capacity retention) over 1000 cycles at 5C. By comparison, the α-V2O5 NS electrode showed an inferior specific capacity of 163.3 mA h g−1 (74.6% capacity retention) over 1000 cycles under the same test conditions. Furthermore, the W-V2O5 NS electrode was further cycled at 40C to study the advanced long-term durability (Fig. S14†). A capacity retention of up to 85.7% was achieved by the W-V2O5 NS electrode after 2000 cycles, and such a value was better than the α-V2O5 NS electrode (57.1% capacity retention) and other V2O5-based electrodes (Table S2†).
The influence of the introduced H2O molecules on the reaction kinetics was further assessed by GITT and EIS. The lithium-ion diffusion coefficient (DLi+) of the α-V2O5 and W-V2O5 NS electrode were obtained through GITT (Fig. 3e), and their GITT charge–discharge curves are displayed in Fig. S15.† The DLi+ value in the W-V2O5 NS electrode was 3.4 × 10−11 cm2 s−1, greatly surpassing the α-V2O5 NS electrode (1.1 × 10−12 cm2 s−1). The enlarged ion diffusion channel is one important reason for the greatly improved DLi+ in the W-V2O5 NS electrode. Another important reason is the shielding effect of the introduced H2O molecules, which can eliminate the electrostatic interaction between the V2O5 lattice and lithium ions.53,54 At the same time, EIS was performed for α-V2O5 and W-V2O5 NS electrodes at different voltages in the cathodic process (Fig. 3f and S16†). As the potential increased from 2.0 to 4.0 V vs. Li/Li+, the Ohmic resistance (Rs) was very stable, while the charge-transfer resistance (Rct) increased first and then decreased (Fig. S17†). The W-V2O5 NS electrode revealed an obviously smaller Rs and Rct compared with the α-V2O5 NS electrode, suggesting a faster electron-transfer speed. Additionally, kinetics analysis was conducted to further explore the capacitive behavior of α-V2O5 and W-V2O5 NS electrodes. In the scan rate range of 0.1–2.0 mV s−1, the W-V2O5 NS electrode could maintain the shape of the CV curve without significant changes (Fig. 3g), revealing a quick responsiveness to high-speed scanning for LIBs. The formula i = avb can be used to assess the capacitive effect,55 which considers the current density (i), scan rate (v), and two parameters (a, b), whereby when b is equal to 0.5 and 1.0, the capacitive effect is a diffusion-controlled process and surface-controlled process, respectively. The calculated b values from peak 1 to peak 6 were 0.76, 0.86, 0.83, 0.79, 0.84, and 0.82, respectively (Fig. 3h). These values were all between 0.5 and 1.0, indicating that the capacitive effect of the W-V2O5 NS electrode was a mixture of diffusion-controlled and surface-controlled processes. Besides, the capacitance contribution could be further quantitatively differentiated into capacitive-controlled effects and diffusion-controlled effects by applying the formula i (V) = k1v + k2v1/2.56 As the scan rate ranged from 0.1 to 2.0 mV s−1, the capacitive contributions in the W-V2O5 NS electrode were 50.6%, 54.2%, 61.0%, 67.8%, 73.1%, 76.7%, and 83.2% (Fig. 3i). This shows that the diffusion-controlled process played a dominant role in the capacitance behavior. It can be seen from Fig. S18† that the capacitive contribution in the W-V2O5 NS electrode was larger than in the α-V2O5 NS electrode, revealing enhanced rapid electron and ion migration, which were beneficial for delivering excellent rate performance.
Subsequently, a W-V2O5//graphite DIES device was fabricated by applying the W-V2O5 NS anode and graphite cathode (Fig. 4a). In the process of charging, the anion PF6− migrated from the electrolyte to the cathode, and intercalated with the graphite cathode to generate the graphite intercalation compound Cx(PF6). Simultaneously, the cation Li+ migrated from the electrolyte to the anode, and intercalated with the V2O5 anode to form the compound LixV2O5. Conversely, the PF6− and Li+ were deintercalated from the graphite and V2O5, respectively, and returned to the electrolyte during discharge. The electrochemical performances of the graphite cathode are displayed in Fig. S19,† which manifested its large specific capacity (124 mA h g−1), high discharge potential (4.6 V), favorable rate capability (111 mA h g−1 at 1 A g−1), and superior lifespan (91.3% capacity retention over 1500 cycle). Therefore, the designed W-V2O5//graphite DIES is expected to have potential as an energy-storage device with high performance.
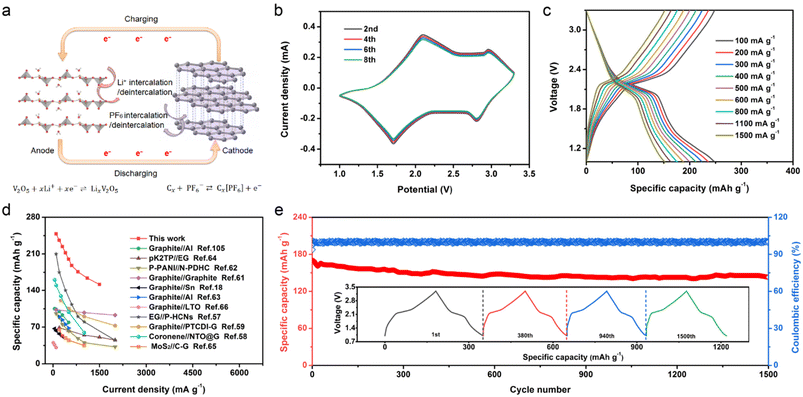 |
| Fig. 4 Electrochemical performances of W-V2O5//graphite DIES device. (a) Schematic illustration, (b) CV curves, (c) charge and discharge curves, (d) rate capability compared with recent works,18,57–66 and (e) cycling performance; inset is the selected charge and discharge curves. | |
The CV curves were obtained from the 2nd, 4th, 6th, and 8th cycles of the W-V2O5//graphite DIES device, and the scan rate was set at 0.5 mV s−1 (Fig. 4b). These CV curves revealed two anode and two cathode peaks, which signified a multistep anion and cation intercalation/deintercalation process. A high coincidence of these CV curves could be identified, indicating a good reversibility and stability during anion and cation intercalation/deintercalation. As expected, the elaborated W-V2O5//graphite DIES device delivered high capacities of 248, 236, 224, 212, 200, 188, 176, 164, and 152 mA h g−1 at 0.1, 0.2, 0.3, 0.4, 0.5, 0.6, 0.8, 1.1, and 1.5 A g−1, respectively (Fig. 4c). The W-V2O5//graphite DIES device demonstrated a high specific capacity and superior rate capability (Fig. 4d), which substantially outweighed most other reported DIES devices at comparable current densities, such as EG//P-HCNs (210 mA h g−1 at 0.1 A g−1),57 coronene//NTO@G (161 mA h g−1 at 0.05 A g−1),58 graphite//PTCDI-G (121 mA h g−1 at 0.25 A g−1),59 graphite//Al (105 mA h g−1 at 0.05 A g−1),60 graphite//graphite (103 mA h g−1 at 0.1 A g−1),61 P-PANI//N-PDHC (96 mA h g−1 at 0.1 A g−1),62 graphite//Al (90 mA h g−1 at 0.2 A g−1),63 pK2TP//EG (69 mA h g−1 at 0.2 A g−1),64 graphite//Sn (67 mA h g−1 at 0.05 A g−1),18 MoS2//C-G (65 mA h g−1 at 0.2 A g−1),65 and graphite//LTO (40 mA h g−1 at 0.01 A g−1).66 Furthermore, when the current density varied from 0.1 to 1.5 A g−1, the capacity retention of the W-V2O5//graphite device was as high as 70.4%, suggesting good charge-transfer kinetics and high reversibility. Moreover, the W-V2O5//graphite DIES device exhibited excellent cycling durability (84% retention) and released a capacity of up to 143 mA h g−1 over 1500 cycles at 1.0 A g−1 (Fig. 4e), which surpasses most reported DIES devices, for instance, EG//P-HCNs (121 mA h g−1 at 0.5 A g−1 over 1500 cycles),57 graphite//PTCDI-G (116.3 mA h g−1 at 0.25 A g−1 over 300 cycles),59 graphite//Al (91.52 mA h g−1 at 0.2 A g−1 over 200 cycles),60 graphite//Al (86.93 mA h g−1 at 0.2 A g−1 over 500 cycles),63 P-PANI//N-PDHC (75 mA h g−1 at 0.1 A g−1 over 100 cycles),62 and pK2TP//EG (63 mA h g−1 at 0.5 A g−1 over 200 cycles).64 As depicted in the inset of Fig. 4, the GCD curves of the 1st, 380th, 940th, and 1500th cycles displayed little capacity attenuation, revealing a good durability property for the W-V2O5//graphite DIES device again. Additionally, the W-V2O5//graphite DIES device possessed an admirable coulombic efficiency, which behaved very smoothly and infinitely approached 100% throughout the whole electrochemical process.
The combination of fast kinetics with superb capacity led to the W-V2O5//graphite DIES device obtaining a peak energy density of 481.1 W h kg−1 at 0.19 kW kg−1, as shown in Fig. 5 (based on the total mass of active materials). Such a value surpasses the recently reported DIES devices, such as PANI//PDI-EDA (29.5 W h kg−1),67 LMO//N-NS/nS (30 W h kg−1),68 coronene//NTO@G (78 W h kg−1),58 graphite//Al (113 W h kg−1),60 graphite//LTO (150 W h kg−1),66 graphite//Al (178 W h kg−1),63 EG//P-HCNs (180 W h kg−1),57 graphite//graphite (190 W h kg−1),61 MCMB//Al (210 W h kg−1),69 graphite//PTCDI-G (230 W h kg−1),59m-PTPA//Zn (235 W h kg−1),70 and P-PANI//N-PDHC (295 W h kg−1).62 Beyond that, the W-V2O5//graphite DIES device also provided a top power density of up to 3.08 kW kg−1, which is comparable to that of supercapacitors.71–73 Additionally, we also manufactured a lithium-ion full-cell with a commercial Li3VO4 positive electrode and W-V2O5 negative electrode for comparison (Fig. S20†). Obviously, the W-V2O5//graphite DIES device was superior to the W-V2O5//Li3VO4 LIB device in terms of both energy density and power density. Encouragingly, the W-V2O5//graphite DIES device could be employed to power light-emitting diode (LED) indicators (inset in Fig. 5), which confirmed the potential application of the fabricated W-V2O5//graphite DIES in portable electronics devices.
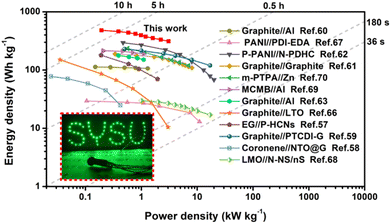 |
| Fig. 5 Ragone plots of the W-V2O5//graphite DIES device compared with other recently reported DIES devices.57–63,66–73 Inset is the image of an LED indicator lit up by the W-V2O5//graphite DIES device. | |
Conclusions
To sum up, we reported a simple and effective H2O-incorporation approach to design a V2O5 anode with an extended interlayer gap. This strategy could strongly stimulate the lithium-storage performance of the W-V2O5 NS electrode, greatly boosting its rate capability and cycle life. The introduced H2O molecules served as interlayer pillars to expand the interlayer gaps and stabilize the structure, which could offer large space and abundant channels for ion diffusion and electron transport, thus promoting the rapid electrochemical kinetics. Moreover, the introduced H2O molecules could eliminate the electrostatic interaction between the V2O5 lattice and lithium ions. This could effectively buffer the volume variation of the W-V2O5 NS electrode in the charge–discharge cycle process, thereby validly enhancing its cycling durability. Additionally, because the lattice oxygen in W-V2O5 NS was replaced by the equivalent mole of H2O molecules, the enhanced rate performance and durability were not a trade-off for the capacity. More importantly, a DIES device was assembled by coupling the anion-intercalation graphite cathode and cation-intercalation W-V2O5 NS anode, which could combine fast kinetics with intercalation-type charge storage. Delightfully, an admirable energy density and power density were achieved by the W-V2O5//graphite DIES device, which were as high as 481.1 W h kg−1 and 3.08 kW kg−1, respectively. This work can provide a reference and ideas about the interlayer engineering strategy for designing fast-kinetics and long-life layered electrode materials in DIBs and other energy-storage systems.
Conflicts of interest
There are no conflicts to declare.
Acknowledgements
We acknowledge the Basic and Applied Basic Research Project of Guangdong Province (2019A1515110827), Science and Technology Planning Project of Guangzhou (202102080169), Education Commission of Guangdong Province (2022ZDZX3048), Advanced Functional Materials Scientific Research and Technical Service Team (X20190197), Undergraduate research projects of Guangdong Industry Polytechnic College (XSKYL202208), and Exquisite Education Project of Guangdong Industry Polytechnic College (JZYR202120).
References
- H. Z. Zhang, W. X. Wu, Q. Y. Liu, F. Yang, X. Shi, X. Q. Liu, M. H. Yu and X. H. Lu, Interlayer Engineering of α–MoO3 Modulates Selective Hydronium Intercalation in Neutral Aqueous Electrolyte, Angew. Chem., Int. Ed., 2021, 60, 896–903 CrossRef CAS PubMed.
- M. Yu, R. Dong and X. Feng, Two-dimensional carbon-rich conjugated frameworks for electrochemical energy applications, J. Am. Chem. Soc., 2020, 142, 12903–12915 CrossRef CAS.
- F. Wang, O. Borodin, T. Gao, X. L. Fan, W. Sun, F. D. Han, A. Faraone, J. A. Dura, K. Xu and C. S. Wang, Highly reversible zinc metal anode for aqueous batteries, Nat. Mater., 2018, 17, 543–549 CrossRef CAS PubMed.
- J. X. Zheng, Q. Zhao, T. Tang, J. F. Yin, C. D. Quilty, G. D. Renderos, X. T. Liu, Y. Deng, L. Wang, D. C. Bock, C. Jaye, D. H. Zhang, E. S. Takeuchi, K. J. Takeuchi, A. C. Marschilok and L. A. Archer, Reversible epitaxial electrodeposition of metals in battery anodes, Science, 2019, 366, 645–648 CrossRef CAS PubMed.
- C. F. Liu, Z. Neale, J. Q. Zheng, X. X. Jia, J. J. Huang, M. Y. Yan, M. Tian, M. S. Wang, J. H. Yang and G. Z. Cao, Expanded hydrated vanadate for high-performance aqueous zinc-ion batteries, Energy Environ. Sci., 2019, 12, 2273–2285 RSC.
- L. T. Ma, S. M. Chen, C. B. Long, X. L. Li, Y. W. Zhao, Z. X. Liu, Z. D. Huang, B. B. Dong, J. A. Zapien and C. Y. Zhi, Achieving high-voltage and high–capacity aqueous rechargeable zinc ion battery by incorporating two–species redox reaction, Adv. Energy Mater., 2019, 9, 1902446 CrossRef CAS.
- F. Wan and Z. Q. Niu, Design strategies for vanadium–based aqueous zinc-ion batteries, Angew. Chem., 2019, 131, 16508–16517 CrossRef.
- L. Li, Z. Hu, Y. Lu, C. C. Wang, Q. Zhang, S. Zhao, J. Peng, K. Zhang, S. L. Chou and J. Chen, Angew. Chem., Int. Ed., 2021, 60, 13050–13056 CrossRef CAS PubMed.
- M. H. Yu, H. Shao, G. Wang, F. Yang, C. L. Liang, P. Rozier, C.-Z. Wang, X. H. Lu, P. Simon and X. L. Feng, Interlayer gap widened α-phase molybdenum trioxide as high-rate anodes for dual-ion-intercalation energy storage devices, Nat. Commun., 2020, 11, 1–9 CrossRef.
- Y. M. Sui, C. F. Liu, R. C. Masse, Z. G. Neale, M. Atif, M. AlSalhi and G. Z. Cao, Dual-ion batteries: The emerging alternative rechargeable batteries, Energy Storage Mater., 2020, 25, 1–32 CrossRef.
- X. Li, X. W. Ou and Y. B. Tang, 6.0 V high-voltage and concentrated electrolyte toward high energy density K-based dual-graphite battery, Adv. Energy Mater., 2020, 10, 2002567 CrossRef CAS.
- K. M. Song, J. F. Liu, H. L. Dai, Y. Zhao, S. H. Sun, J. Y. Zhang, C. D. Qin, P. F. Yan, F. Q. Guo, C. Y. Wang, Y. L. Cao, S. F. Li and W. H. Chen, Atomically dispersed Ni induced by ultrahigh N-doped carbon enables stable sodium storage, Chem, 2021, 7, 2684–2694 CAS.
- L. Sui, X. Y. Shi, T. Deng, H. Yang, H. Y. Liu, H. Chen, W. Zhang and W. T. Zheng, Integrated Co3O4/carbon fiber paper for high-performance anode of dual-ion battery, J. Energy Chem., 2019, 37, 7–12 CrossRef.
- S. Wang, J. G. Tu, J. S. Xiao, J. Zhu and S. Q. Jiao, 3D skeleton nanostructured Ni3S2/Ni foam@RGO composite anode for high-performance dual-ion battery, J. Energy Chem., 2019, 28, 144–150 CrossRef.
- L. N. Wu, S. Y. Shen, Y. H. Hong, C. H. Shen, F. M. Han, F. Fu, X. D. Zhou, L. Huang, J. T. Li and S. G. Sun, Novel MnO–Graphite Dual-Ion Battery and New Insights into Its Reaction Mechanism during Initial Cycle by Operando Techniques, ACS Appl. Mater. Interfaces, 2019, 11, 12570–12577 CrossRef CAS PubMed.
- Q. L. Xu, R. Ding, W. Shi, D. F. Ying, Y. F. Huang, T. Yan, P. Gao, X. J. Sun and E. H. Liu, Perovskite KNi0.1Co0.9F3 as a pseudocapacitive conversion anode for high-performance nonaqueous Li-ion capacitors and dual-ion batteries, J. Mater. Chem. A, 2019, 7, 8315–8326 RSC.
- X. F. Tong, F. Zhang, B. F. Ji, M. H. Sheng and Y. B. Tang, Carbon-coated porous aluminum foil anode for high-rate, long-term cycling stability, and high energy density dual-ion batteries, Adv. Mater., 2016, 28, 9979–9985 CrossRef CAS PubMed.
- B. F. Ji, F. Zhang, X. H. Song and Y. B. Tang, A novel potassium-ion-based dual-ion battery, Adv. Mater., 2017, 29, 1700519 CrossRef.
- S. Bellani, F. X. Wang, G. Longoni, L. Najafi, R. Oropesa-Nunez, A. E. Del Rio Castillo, M. Prato, X. D. Zhuang, V. Pellegrini, X. L. Feng and F. Bonaccorso, WS2–graphite dual-ion batteries, Nano Lett., 2018, 18, 7155–7164 CrossRef CAS PubMed.
- C. Y. Cui, Z. X. Wei, J. T. Xu, Y. Q. Zhang, S. H. Liu, H. K. Liu, M. L. Mao, S. Y. Wang, J. M. Ma and S. Dou, Three-dimensional carbon frameworks enabling MoS2 as anode for dual ion batteries with superior sodium storage properties, Energy Storage Mater., 2018, 15, 22–30 CrossRef.
- R. Enjalbert and J. Galy, A refinement of the structure of V2O5, Acta Crystallogr., 1986, 42, 1467–1469 Search PubMed.
- X. Zhao, L. Mao, Q. H. Cheng, F. F. Liao, G. Y. Yang, X. H. Lu and L. Y. Chen, Interlayer engineering of preintercalated layered oxides as cathode for emerging multivalent metal-ion batteries: zinc and beyond, Energy Storage Mater., 2021, 38, 397–437 CrossRef.
- H. B. Geng, M. Cheng, B. Wang, Y. Yang, Y. F. Zhang and C. C. Li, Electronic structure regulation of layered vanadium oxide via interlayer doping strategy toward superior high-rate and low-temperature zinc-ion batteries, Adv. Funct. Mater., 2020, 30, 1907684 CrossRef CAS.
- Y. J. Zhu, M. Yang, Q. Y. Huang, D. R. Wang, R. B. Yu, J. Y. Wang, Z. J. Zheng and D. Wang, V2O5 textile cathodes with high capacity and stability for flexible lithium-ion batteries, Adv. Mater., 2020, 32, 1906205 CrossRef CAS PubMed.
- C. R. Wang, L. Zhang, M. Al-Mamun, Y. H. Dou, P. Liu, D. W. Su, G. X. Wang, S. Q. Zhang, D. Wang and H. J. Zhao, A Hollow-Shell Structured V2O5 Electrode-Based Symmetric Full Li-Ion Battery with Highest Capacity, Adv. Energy Mater., 2019, 9, 1900909 CrossRef.
- D. L. Chao, X. H. Xia, J. L. Liu, Z. X. Fan, C. F. Ng, J. Y. Lin, H. Zhang, Z. X. Shen and H. J. Fan, A V2O5/conductive-polymer core/shell nanobelt array on three-dimensional graphite foam: a high-rate, ultrastable, and freestanding cathode for lithium-ion batteries, Adv. Mater., 2014, 26, 5794–5800 CrossRef CAS.
- J. H. Lee, J. M. Kim, J. H. Kim, Y. R. Jang, J. A. Kim, S. H. Yeon and S. Y. Lee, Toward Ultrahigh-Capacity V2O5 Lithium-Ion Battery Cathodes via One-Pot Synthetic Route from Precursors to Electrode Sheets, Adv. Mater. Interfaces, 2016, 3, 1600173 CrossRef.
- Q. Liu, Z. F. Li, Y. D. Liu, H. Y. Zhang, Y. Ren, C. J. Sun, W. Q. Lu, Y. Zhou, L. Stanciu, E. A. Stach and J. Xie, Graphene-modified nanostructured vanadium pentoxide hybrids with extraordinary electrochemical performance for Li-ion batteries, Nat. Commun., 2015, 6, 1–10 Search PubMed.
- P. G. Bruce, B. Scrosati and J. M. Tarascon, Nanomaterials for rechargeable lithium batteries, Angew. Chem., Int. Ed., 2008, 47, 2930–2946 CrossRef CAS PubMed.
- B. Ju, H. J. Song, H. Yoon and D. W. Kim, Amorphous hydrated vanadium oxide with enlarged interlayer spacing for aqueous zinc-ion batteries, Chem. Eng. J., 2021, 420, 130528 CrossRef CAS.
- X. Peng, X. M. Zhang, L. Wang, L. S. Hu, S. H. S. Cheng, C. Huang, B. Gao, F. Ma, K. F. Huo and P. K. Chu, Hydrogenated V2O5 nanosheets for superior lithium storage properties, Adv. Funct. Mater., 2016, 26, 784–791 CrossRef CAS.
- J. H. Zhang, H. X. Zhang, M. M. Liu, Q. C. Xu, H. Jiang and C. Z. Li, Cobalt-stabilized oxygen vacancy of V2O5 nanosheet arrays with delocalized valence electron for alkaline water splitting, Chem. Eng. Sci., 2020, 227, 115915 CrossRef CAS.
- D. Chen, X. H. Rui, Q. Zhang, H. B. Geng, L. Y. Gan, W. Zhang, C. C. Li, S. M. Huang and Y. Yu, Persistent zinc-ion storage in mass-produced V2O5 architectures, Nano Energy, 2019, 60, 171–178 CrossRef CAS.
- Z. H. Wang, P. Liang, R. G. Zhang, Z. M. Liu, W. Y. Li, Z. G. Pan, H. Yang, X. D. Shen and J. Wang, Oxygen-defective V2O5 nanosheets boosting 3D diffusion and reversible storage of zinc ion for aqueous zinc-ion batteries, Appl. Surf. Sci., 2021, 562, 150196 CrossRef CAS.
- K. Chen, G. D. Zhang, L. P. Xiao, P. W. Li, W. L. Li, Q. C. Xu and J. Xu, Polyaniline Encapsulated Amorphous V2O5 Nanowire-Modified Multi-Functional Separators for Lithium-Sulfur Batteries, Small Methods, 2021, 5, 2001056 CrossRef CAS PubMed.
- J. Liu, H. Xia, D. F. Xue and L. Lu, Double-shelled nanocapsules of V2O5-based composites as high-performance anode and cathode materials for Li ion batteries, J. Am. Chem. Soc., 2009, 131, 12086–12087 CrossRef CAS.
- C. Z. Liu, J. H. Yao, Z. G. Zou, Y. W. Li and G. Z. Cao, Boosting the cycling stability of hydrated vanadium pentoxide by Y3+ pillaring for sodium-ion batteries, Mater. Today Energy, 2019, 11, 218–227 CrossRef CAS.
- H. D. Chen, J. J. Huang, S. H. Tian, L. Liu, T. F. Qin, L. Song, Y. P. Liu, Y. N. Zhang, X. G. Wu, S. L. Lei and S. L. Peng, Interlayer Modification of Pseudocapacitive Vanadium Oxide and Zn(H2O)n2+ Migration Regulation for Ultrahigh Rate and Durable Aqueous Zinc-Ion Batteries, Adv. Sci., 2021, 8, 2004924 CrossRef CAS.
- W. D. Qiu, Y. L. Tian, Z. C. Lin, S. T. Lin, Z. Q. Geng, K. T. Huang, A. H. Lei, F. C. Huang, H. J. Feng, F. Z. Ding and X. H. Lu, High rate and ultralong life flexible all-solid-state zinc ion battery based on electron density modulated NiCo2O4 nanosheets, J. Energy Chem., 2022, 70, 283–291 CrossRef CAS.
- N. N. Liu, X. Wu, L. S. Fan, S. Gong, Z. K. Guo, A. S. Chen, C. Y. Zhao, Y. C. Mao, N. Q. Zhang and K. N. Sun, Intercalation Pseudocapacitive Zn2+ Storage with Hydrated Vanadium Dioxide toward Ultrahigh Rate Performance, Adv. Mater., 2020, 32, 1908420 CrossRef CAS PubMed.
- E. B. Deeva, A. Kurlov, P. M. Abdala, D. Lebedev, S. M. Kim, C. P. Gordon, A. Tsoukalou, A. Fedorov and C. R. Müller, In situ XANES/XRD study of the structural stability of two-dimensional molybdenum carbide Mo2CTx: Implications for the catalytic activity in the water–gas shift reaction, Chem. Mater., 2019, 31, 4505–4513 CrossRef CAS.
- S. J. Deng, Y. Zhang, D. Xie, L. Yang, G. Z. Wang, X. S. Zheng, J. F. Zhu, X. L. Wang, Y. Yu, G. X. Pan, X. H. Xia and J. P. Tu, Oxygen vacancy modulated Ti2Nb10O29−x embedded onto porous bacterial cellulose carbon for highly efficient lithium ion storage, Nano Energy, 2019, 58, 355–364 CrossRef CAS.
- Y. Gogotsi and P. Simon, True performance metrics in electrochemical energy storage, Science, 2011, 334, 917–918 CrossRef CAS PubMed.
- Y. Nakayama, K. Morii, Y. Suzuki, H. Machida, S. Kera, N. Ueno, H. Kitagawa, Y. Noguchi and H. Ishii, Origins of improved hole-injection efficiency by the deposition of MoO3 on the polymeric semiconductor poly (dioctylfluorene-alt-benzothiadiazole), Adv. Funct. Mater., 2009, 19, 3746–3752 CrossRef CAS.
- H. Morikawa, M. Miyake, S. I. Iwai, K. Furukawa and A. Revcolevschi, Structural analysis of molten V2O5, J. Chem. Soc., Faraday Trans. 1, 1981, 77, 361–367 RSC.
- Y. X. Zeng, Z. Z. Lai, Y. Han, H. Z. Zhang, S. L. Xie and X. H. Lu, Oxygen-vacancy and surface modulation of ultrathin nickel cobaltite nanosheets as a high-energy cathode for advanced Zn-ion batteries, Adv. Mater., 2018, 30, 1802396 CrossRef PubMed.
- L. Q. Mai, Q. Y. An, Q. L. Wei, J. Y. Fei, P. F. Zhang, X. Xu, Y. L. Zhao, M. Y. Yan, W. Wen and L. Xu, Nanoflakes-Assembled Three-Dimensional Hollow-Porous V2O5 as Lithium Storage Cathodes with High-Rate Capacity, Small, 2014, 10, 3032 CrossRef CAS PubMed.
- A. Q. Pan, H. B. Wu, L. Yu and X. W. Lou, Emplate-free synthesis of VO2 hollow microspheres with various interiors and their conversion into V2O5 for lithium-ion batteries, Angew. Chem., 2013, 125, 2282–2286 CrossRef.
- S. F. Xu, H. C. Dai, S. L. Zhu, Y. C. Wu, M. X. Sun, Y. Chen, K. Fan, C. Y. Zhang, C. L. Wang and W. P. Hu, A branched dihydrophenazine-based polymer as a cathode material to achieve dual-ion batteries with high energy and power density, eScience, 2021, 1, 60–68 CrossRef.
- J. M. Son, S. Oh, S. H. Bae, S. Nam and I. K. Oh, A Pair of NiCo2O4 and V2O5 Nanowires Directly Grown on Carbon Fabric for Highly Bendable Lithium-Ion Batteries, Adv. Energy Mater., 2019, 9, 1900477 CrossRef.
- X. B. Yu, G. Y. Zhao, C. Liu, C. L. Wu, H. H. Huang, J. J. He and N. Q. Zhang, A MoS2 and Graphene Alternately Stacking van der Waals Heterostructure for Li+/Mg2+ Co-Intercalation, Adv. Funct. Mater., 2021, 31, 2103214 CrossRef CAS.
- Y. L. Shan, L. Xu, Y. J. Hu, H. Jiang and C. Z. Li, Internal-diffusion controlled synthesis of V2O5 hollow microspheres for superior lithium-ion full batteries, Chem. Eng. Sci., 2019, 200, 38–45 CrossRef CAS.
- H. J. Lee, J. Shin and J. W. Choi, Intercalated water and organic molecules for electrode materials of rechargeable batteries, Adv. Mater., 2018, 30, 1705851 CrossRef.
- D. Kundu, B. D. Adams, V. Duffort, S. H. Vajargah and L. F. Nazar, A high-capacity and long-life aqueous rechargeable zinc battery using a metal oxide intercalation cathode, Nat. Energy, 2016, 1, 1–8 Search PubMed.
- V. Augustyn, J. Come, M. A. Lowe, J. W. Kim, P.-L. Taberna, S. H. Tolbert, H. D. Abruña, P. Simon and B. Dunn, High-rate electrochemical energy storage through Li+ intercalation pseudocapacitance, Nat. Mater., 2013, 12, 518–522 CrossRef CAS PubMed.
- J. L. Liu, J. Wang, C. H. Xu, H. Jiang, C. Z. Li, L. L. Zhang, J. Y. Lin and Z. X. Shen, Advanced energy storage devices: basic principles, analytical methods, and rational materials design, Adv. Sci., 2018, 5, 1700322 CrossRef PubMed.
- X. Y. Wang, S. F. Wang, K. X. Shen, S. G. He, X. H. Hou and F. M. Chen, Phosphorus-doped porous hollow carbon nanorods for high-performance sodium-based dual-ion batteries, J. Mater. Chem. A, 2020, 8, 4007–4016 RSC.
- S. Y. Dong, Z. F. Li, I. A. Rodríguez-Pérez, H. Jiang, J. Lu, X. G. Zhang and X. L. Ji, A novel coronene//Na2Ti3O7 dual-ion battery, Nano Energy, 2017, 40, 233–239 CrossRef CAS.
- Z. D. Huang, Y. Hou, T. R. Wang, Y. W. Zhao, G. J. Liang, X. L. Li, Y. Guo, Q. Yang, Z. Chen, Q. Li, L. T. Ma, J. Fan and C. Y. Zhi, Manipulating anion intercalation enables a high-voltage aqueous dual ion battery, Nat. Commun., 2021, 12, 1–11 CrossRef PubMed.
- X. L. Zhang, Y. B. Tang, F. Zhang and C. S. Lee, A novel aluminium-graphite dual-ion battery, Adv. Energy Mater., 2016, 6, 1502588 CrossRef.
- Y. Wang, Y. J. Zhang, S. Wang, S. Y. Dong, C. Q. Dang, W. C. Hu and D. Y. Yu, Ultrafast Charging and Stable Cycling Dual-Ion Batteries Enabled via an Artificial Cathode-Electrolyte Interface, Adv. Funct. Mater., 2021, 31, 2102360 CrossRef CAS.
- Z. Q. Sun, K. J. Zhu, P. Liu, H. X. Li and L. F. Jiao, Optimized Cathode for High-Energy Sodium-Ion Based Dual-Ion Full Battery with Fast Kinetics, Adv. Funct. Mater., 2021, 31, 2107830 CrossRef CAS.
- L. Xiang, X. W. Ou, X. Y. Wang, Z. M. Zhou, X. Li and Y. B. Tang, Highly concentrated electrolyte towards enhanced energy density and cycling life of dual-ion battery, Angew. Chem., Int. Ed., 2020, 59, 17924–17930 CrossRef CAS PubMed.
- A. Yu, Q. G. Pan, M. Zhang, D. H. Xie and Y. B. Tang, Fast rate and long life potassium-ion based dual-ion battery through 3D porous organic negative electrode, Adv. Funct. Mater., 2020, 30, 2001440 CrossRef CAS.
- H. L. Zhu, F. Zhang, J. R. Li and Y. B. Tang, Penne-like MoS2/carbon nanocomposite as anode for sodium-ion-based dual-ion battery, Small, 2018, 14, 1703951 CrossRef PubMed.
- X. Y. Shi, S. S. Yu, T. Deng, W. Zhang and W. Zheng, Unlock the potential of Li4Ti5O12 for high-voltage/long-cycling-life and high-safety batteries: Dual-ion architecture superior to lithium-ion storage, J. Energy Chem., 2020, 44, 13–18 CrossRef.
- Y. P. Zhu, J. Yin, A. H. Emwas, O. F. Mohammed and H. N. Alshareef, An Aqueous Mg2+-Based Dual-Ion Battery with High Power Density, Adv. Funct. Mater., 2021, 31, 2107523 CrossRef CAS.
- J. M. Park, M. Jana, P. Nakhanivej, B.-K. Kim and H. S. Park, Facile multivalent redox chemistries in water-in-bisalt hydrogel electrolytes for hybrid energy storage full cells, ACS Energy Lett., 2020, 5, 1054–1061 CrossRef CAS.
- F. Zhang, B. F. Ji, X. F. Tong, M. H. Sheng, X. L. Zhang, C. S. Lee and Y. B. Tang, A dual-ion battery constructed with aluminum foil anode and mesocarbon microbead cathode via an alloying/intercalation process in an ionic liquid electrolyte, Adv. Mater. Interfaces, 2016, 3, 1600605 CrossRef.
- H. Z. Zhang, L. F. Zhong, J. H. Xie, F. Yang, X. Q. Liu and X. H. Lu, A COF-like N-rich conjugated microporous polytriphenylamine cathode with pseudocapacitive anion storage behavior for high-energy aqueous zinc dual-ion batteries, Adv. Mater., 2021, 33, 2101857 CrossRef CAS PubMed.
- S. M. Chen, L. T. Ma, K. Zhang, M. Kamruzzaman, C. Y. Zhi and J. A. Zapien, A flexible solid-state zinc ion hybrid supercapacitor based on co-polymer derived hollow carbon spheres, J. Mater. Chem. A, 2019, 7, 7784–7790 RSC.
- Y. Z. Jiao, H. T. Zhang, H. L. Zhang, A. Liu, Y. X. Liu and S. J. Zhang, Highly bonded T-Nb2O5/rGO nanohybrids for 4 V quasi-solid state asymmetric supercapacitors with improved electrochemical performance, Nano Res., 2018, 11, 4673–4685 CrossRef CAS.
- C. Zhou, Y. W. Zhang, Y. Y. Li and J. P. Liu, Construction of high-capacitance 3D CoO@polypyrrole nanowire array electrode for aqueous asymmetric supercapacitor, Nano Lett., 2013, 13, 2078–2085 CrossRef CAS PubMed.
Footnotes |
† Electronic supplementary information (ESI) available: SEM image, TEM image, N2 adsorption–desorption isotherm, FTIR spectra, Raman spectra, Raman peak assignment, survey and Li 1s XPS spectra, EPR spectra, Mott–Schottky plots, TGA curves, V L edges XANES spectra, UPS spectra, CV curves, comparison of electrochemical performance, charge and discharge profiles, cycling performance, GITT charge discharge curves, Nyquist plots, Rs and (b) Rct as a function of potential, CV curves, log(i) vs. log(v) plots, capacitive contribution for α-V2O5 and W-V2O5 sample. Electrochemical performance of the graphite cathode and W-V2O5//Li3VO4 device. See DOI: https://doi.org/10.1039/d2qi02080g |
‡ These authors contributed equally to this work. |
|
This journal is © the Partner Organisations 2023 |
Click here to see how this site uses Cookies. View our privacy policy here.