DOI:
10.1039/D2QI02118H
(Research Article)
Inorg. Chem. Front., 2023,
10, 280-287
A defect engineered p-block SnS2−x catalyst for efficient electrocatalytic NO reduction to NH3†
Received
2nd October 2022
, Accepted 10th November 2022
First published on 10th November 2022
Abstract
Electrocatalytic NO reduction to NH3 (NORR) has emerged as an attractive approach for simultaneously realizing NO abatement and ammonia generation. Herein, we demonstrate for the first time that p-block Sn-based materials are promising NORR catalysts. A defect engineering strategy is employed to develop a SnS2−x catalyst enriched with S-vacancy (VS) defects. SnS2−x delivers an exceptional NH3-faradaic efficiency of 90.3% with an NH3 yield rate of 78.6 μmol h−1 cm−2, outperforming most reported d-block NORR catalysts. Theoretical computations unveil that the high NORR performance of SnS2−x arises from the active Sn-VS sites which can selectively activate NO and reduce the energy barriers of the NORR pathway.
Ammonia (NH3) is an essential chemical and also a clean energy carrier.1–3 The Haber–Bosch technology for industrial NH3 production is performed at a high temperature and pressure, resulting in large amounts of energy consumption and CO2 emissions.4–6 The electrocatalytic nitrogen reduction reaction (NRR) has been widely deemed as a promising route to replace the Haber–Bosch process for sustainable NH3 synthesis.7–15 However, the effective NRR is heavily restricted by the low water solubility of N2, highly stable N
N bonds, and the competing hydrogen evolution reaction (HER),16–24 giving rise to extremely low NH3 yield rates and poor NH3-Faraday efficiency (FENH3). Alternatively, given the better water solubility of NO and its relatively low N
O bond energy, electrocatalytic NO reduction to NH3 (NORR) has recently emerged as a more energetically efficient route than the NRR for NH3 electrosynthesis.25–33 In the meantime, NO is a common atmospheric contaminant that causes serious environmental issues.34 Thus, the NORR represents an attractive approach for simultaneously realizing NO abatement and ammonia generation.35–37 Nevertheless, the practical application of the NORR is still hindered by its unsatisfactory reaction kinetics and energetics,38–40 and thus there is an urgent need to develop efficient catalysts to promote the NORR efficiency.
Until now, an increasing number of d-block metal-based phosphides,36 carbides,25 oxides41 and sulfides27 have been developed as efficient NORR catalysts, owing to the great advantage of unoccupied d-orbitals in activating NO molecules. Nonetheless, the unoccupied d-orbitals also easily bind with H atoms to favor the competing HER,42 leading to the compromised NORR selectivity. Recently, main-group p-block Bi-based materials have been confirmed as effective NORR catalysts with high FENH3,35,37 ascribed to the preferential binding of NO molecules rather than H atoms on Bi during the NORR process,35 highlighting the great potential of p-block metal-based materials as promising catalysts for the NORR. Given that p-block Sn possesses the robust ability to activate nitrogenous molecules and suppress the HER,43–45 while defect engineering can maximize the exposed active sites,46–48 we anticipate that defect engineered Sn-based catalysts might be appealing candidates toward the active and selective NORR.
Herein, we employed a defect engineering strategy to develop a p-block SnS2−x catalyst enriched with S-vacancy (VS) defects. SnS2−x delivers an exceptional FENH3 of 90.3% with an NH3 yield of 78.6 μmol h−1 cm−2, exceeding those of most reported d-block NORR catalysts. Theoretical computations are conducted to further provide atomic-level insight into the NORR mechanism of SnS2−x.
A hydrothermal approach is first used to prepare SnS2 nanosheet arrays grown on carbon cloth (CC), which is then subjected to plasma treatment to create VS defects on SnS2 and obtain SnS2−x.43,44,48 The X-ray diffraction (XRD, Fig. S1†) patterns of both SnS2/CC and SnS2−x/CC reveal the diffraction peaks matching well with the crystal planes of the SnS2 phase (JCPDS no. 23-677). The scanning electron microscopy (SEM, Fig. 1a and b) images of SnS2−x/CC show that abundant SnS2−x nanosheets are densely covered on CC, similar to the morphology of SnS2/CC (Fig. S2†), while the nanosheet morphology of SnS2−x can be further ascertained by the transmission electron microscopy (TEM, Fig. 1c) image. The high-resolution TEM (HRTEM, Fig. 1d) image of SnS2−x reveals two d-spacing values of 0.59 and 0.32 nm, corresponding to the (001) and (100) facets of SnS2. The inverse fast Fourier transform (IFFT, Fig. 1e and f) maps recorded from the marked e and f regions in Fig. 1d show many lattice distortions or dislocations,49–51 which can be more clearly reflected by their corresponding 3D surface plots (Fig. 1g and h),52,53 suggesting the existence of abundant lattice defects (i.e. VS) on SnS2−x.
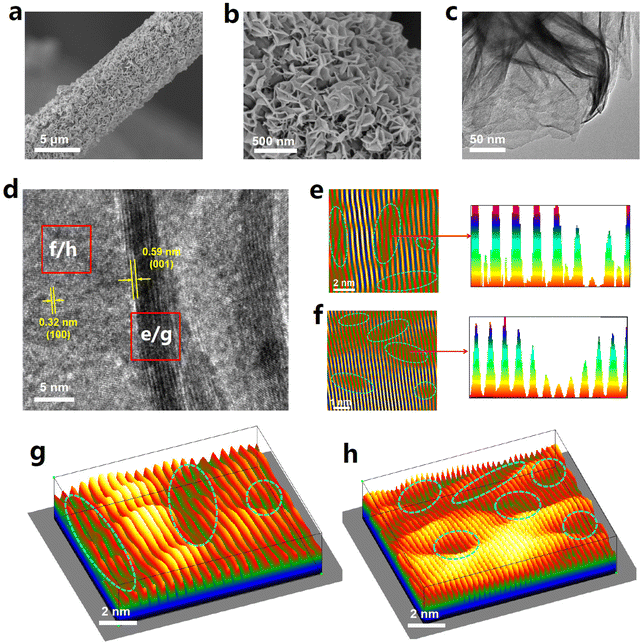 |
| Fig. 1 (a and b) SEM images of SnS2−x/CC. (c) TEM image of SnS2−x. (d) HRTEM image of SnS2−x. (e and f) IFFT maps and (g and h) 3D surface plots of the selected regions in (d) and the corresponding line intensity profiles. | |
X-ray photoelectron spectroscopy (XPS) and X-ray absorption spectroscopy (XAS) measurements are conducted to investigate the chemical states and coordination configurations of SnS2−x. The XPS Sn 3d spectra of SnS2−x (Fig. 2a) can be deconvoluted into Sn 3d5/2 (487.1 eV) and Sn 3d3/2 (495.6 eV), while the S 2p spectra (Fig. 2b) can be fitted into S 2p3/2 (161.3 eV) and S 2p1/2 (162.5 eV). It is apparent that the core-level Sn 3d5/2 and S 2p3/2 peaks of SnS2−x are both negatively shifted compared to those of SnS2, indicating the decreased Sn/S valence states of SnS2−x due to the introduction of electron-rich VS.52 This can also be proved by the Sn K-edge X-ray absorption near-edge structure (XANES, Fig. 2c) spectra, displaying the reduced edge energy of SnS2−x relative to SnS2. The Sn K-edge extended X-ray absorption fine structure (EXAFS, Fig. 2d) spectra of SnS2−x reveal two prominent peaks at 2.08 Å and 3.37 Å, which are assigned to Sn–S and Sn–Sn coordination bonds, respectively. Obviously, compared to SnS2, the Sn–S bond intensity of SnS2−x is markedly decreased, whereas its Sn–Sn bond intensity remains nearly unchanged, suggesting that the introduced vacancies in SnS2−x are S-vacancies rather than Sn-vacancies.52 Meanwhile, the wavelet transform (WT) contour maps show that in comparison with SnS2 (Fig. 2e), SnS2−x presents a noticeable change in the shape of WT profiles (Fig. 2f), which is attributed to the VS-induced distortion of the Sn–S bond structure. Furthermore, the electron paramagnetic resonance (EPR, Fig. 2g) spectra show that SnS2−x exhibits a much stronger g signal (2.001) than SnS2, further confirming that SnS2−x is enriched with VS.51
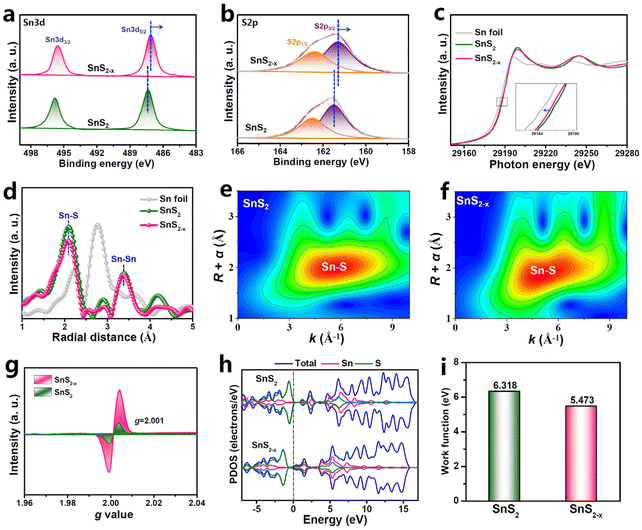 |
| Fig. 2 (a and b) XPS spectra of SnS2 and SnS2−x: (a) Sn 3d, (b) S 2p. (c) Sn K-edge XANES, (d) EXAFS spectra and (e and f) WT profiles of (e) SnS2 and (f) SnS2−x. (g) EPR spectra of SnS2 and SnS2−x. (h) PDOS profiles of SnS2 and SnS2−x. (i) Calculated work functions of SnS2 and SnS2−x. | |
Density functional theory (DFT) calculations are carried out to examine the electronic structures of SnS2 and SnS2−x. The projected density of state (PDOS, Fig. 2h) analyses show that, in contrast to semiconducting SnS2 with a noticeable band gap at the Fermi level, a new defect level appears at the conduction band minimum of SnS2−x, which reduces the band gap and enhances the conductivity,54–58 consistent with the electrochemical impedance spectroscopy measurements (Fig. S3†). The enhanced conductivity is favorable for the accelerated electron transfer efficiency and boosts the catalytic NORR kinetics.59–64 Additionally, the calculated average potential profiles (Fig. 2i, and Fig. S4†) show that SnS2−x exhibits a lower work function (5.473 eV) than SnS2 (6.316 eV), which implies the stronger capability of SnS2−x for the back-donation of electrons to the absorbed NO and reaction intermediates, promoting the NO activation and protonation.6 Moreover, ab initio molecular dynamics (AIMD) simulations (Fig. S5†) reveal the high thermodynamic stability of SnS2−x, in which the energy and temperature can keep the equilibrium states at 700 K, suggesting the stable VS structure in SnS2−x.3
Electrochemical NORR measurements are performed in NO-saturated 0.5 M Na2SO4 solution using a gas-tight two-compartment electrolytic cell.27 All potentials are calibrated to the reversible hydrogen electrode (RHE). The linear sweep voltammetry (LSV, Fig. 2a) curve of SnS2−x/CC in the NO-saturated electrolyte shows a noticeably higher current density than that in an Ar-saturated electrolyte, attesting that SnS2−x/CC is active for the NORR. To quantify the NORR performance of SnS2−x/CC, chronoamperometry tests are conducted at various potentials for 1 h and the products are analyzed by colorimetric methods (Fig. S6–S8†) and gas chromatography. As shown in Fig. 3b, SnS2−x/CC presents the maximum NH3 yield of 78.6 μmol h−1 cm−2 along with the highest FENH3 of 90.3% at −0.7 V, superior to those of most reported d-block NORR catalysts (Table S1†). The reduced NORR performance at potentials higher than −0.7 V is ascribed to the boosted HER (Fig. 3c). Meanwhile, the FEs and partial current densities of N2 and N2H4 by-products are rather low (Fig. 3c and Fig. S9†), suggesting the high NORR selectivity of SnS2−x/CC toward the NH3 synthesis. Besides the high NORR activity and selectivity, SnS2−x/CC also exhibits an excellent stability during the seven consecutive cycles (Fig. 3d) and the long-term chronopotentiometric test for 20 h (Fig. 3e).
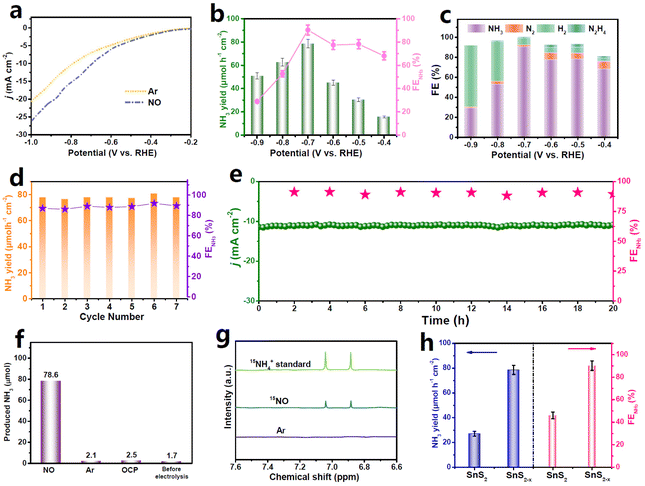 |
| Fig. 3 (a) LSV curves of SnS2−x/CC in Ar-/NO-saturated 0.5 M Na2SO4. (b) NH3 yields and FENH3 of SnS2−x/CC at various potentials. (c) FEs of different products at various potentials. (d) Cycling and (e) long-term tests of SnS2−x/CC at −0.7 V. (f) Amounts of produced NH3 over SnS2−x/CC under different conditions at −0.7 V. (g) 1H NMR spectra of the 15NH4+ standard sample and those fed with 15NO and Ar after NORR electrolysis on SnS2−x/CC at −0.7 V. (h) Comparison of the NORR performance between SnS2/CC and SnS2−x/CC at −0.7 V. | |
We performed control tests to verify the NH3 source.26 As shown in Fig. 3f, negligible NH3 can be detected in the electrolytes under Ar conditions and at the open circuit potential (OCP). The NO-Ar gas switching experiment (Fig. S10†) reveals that NH3 can only be prominently observed in NO-feeding cycles. In the isotopic labeling 1H nuclear magnetic resonance (NMR, Fig. 3g) spectra, feeding 15NO gas leads to a distinct 15NH4+ signal, whereas the 15NH4+ signal is absent under Ar conditions. These control experiments validate that the detected NH3 is derived from the NORR. In addition, NO3− is barely detected after electrolysis (Fig. S11†), suggesting that our gas-tight cell can effectively restrict NO oxidation during the NORR measurements.35
For comparison, the NORR performance of pristine SnS2/CC is also evaluated under identical electrolysis conditions (Fig. 3h). The NH3 yield and FENH3 of SnS2/CC are found to be 2.8 and 1.8 times poorer than those of SnS2−x/CC, respectively, highlighting the significance of the defect engineering strategy to substantially promote the NORR performance of SnS2−x. The origin of the enhanced NORR performance of SnS2−x relative to SnS2 is then unraveled by theoretical computations as described below.
As shown in Fig. 4a, the NO molecule can hardly be absorbed on pristine SnS2, showing negligible N
O bond elongation and near-zero SnS2-to-NO electron transfer. In sharp contrast, NO can be favorably absorbed on SnS2−x where the N atom of NO is chemically bonded with the VS-induced unsaturated Sn site (Sn-VS).65 In this scenario, Sn-VS donates remarkable −0.6 |e| electrons to the anti-bonding orbital of NO and the N
O bond is largely elongated to 1.271 Å.66–68 Besides, in comparison with the pristine Sn site of SnS2, the absorbed NO on the Sn-VS of SnS2−x shows an enhanced *NO-p/Sn-p orbital hybridization at the Fermi level (Fig. S12†). Hence, Sn-VS plays a critical role in effectively activating NO and dissociating the N
O bond.
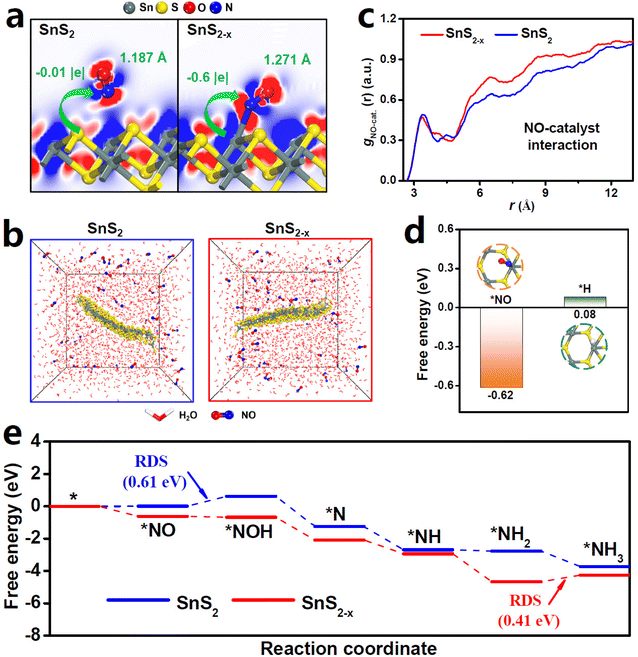 |
| Fig. 4 (a) Electron contour maps of adsorbed NO on SnS2 and SnS2−x. Blue: charge depletion. Red: charge accumulation. (b) Snapshots of the final states of the dynamic process of NO adsorption on SnS2−x and SnS2 after MD simulations, and the corresponding (c) RDF curves. (d) Free energies of *NO and *H on SnS2−x. (e) Free energy diagrams of the NORR process on SnS2−x and SnS2. | |
Molecular dynamics (MD) simulations are also performed to investigate the dynamic process of NO absorption. After simulations (Fig. 4b and Fig. S13†), it is obvious that SnS2−x shows more NO coverage than SnS2, and the corresponding radial distribution function (RDF, Fig. 4c) curves reveal a stronger NO-SnS2−x interaction than the NO-SnS2 interaction,6 confirming, once again, the robust ability of SnS2−x for NO adsorption and coverage. In addition, after examining the competitive adsorption between *NO and *H (Fig. 4d), we found that the Sn-VS site can selectively absorb *NO (−0.62 eV) rather than *H (0.16 eV), thereby resulting in the suppressed HER and thus the enhanced NORR selectivity of SnS2−x.
The free energy profiles are then constructed to investigate the entire NORR pathway on SnS2 and SnS2−x (Fig. 4e, Fig. S14, S15 and Table S2†).29 Impressively, owing to the strong NO activation enabled by Sn-VS, SnS2−x even undergoes an endergonic process for the first protonation step of *NO → *NOH, whereas SnS2 requires a high energy input of 0.61 eV to overcome *NO → *NOH which is the rate-determining step (RDS). The RDS of SnS2−x is *NH2 → *NH3 with only 0.41 eV uphill, which is lower than that of most previously reported catalysts.27,28,35 Additionally, the free energies of all the intermediates on SnS2−x are lower than those on SnS2, attesting the enhanced protonation energetics of SnS2−x to promote the NORR pathway.
In conclusion, SnS2−x has been verified to be an active, selective and durable NORR catalyst. Theoretical computations revealed the critical function of Sn-VS sites to selectively activate NO and reduce the energy barriers of the NORR pathway. This work highlights the great potential of p-block metal elements for developing high-performance NORR catalysts for NH3 electrosynthesis.
Conflicts of interest
There are no conflicts of interest to declare.
Acknowledgements
This work is supported by the Natural Science Foundation of Gansu Province (20JR10RA241).
References
- J. B. Howard and D. C. Rees, Structural basis of biological nitrogen fixation, Chem. Rev., 1996, 96, 2965–2982 CrossRef CAS.
- J. Liang, Q. Liu, A. A. Alshehri and X. Sun, Recent advances in nanostructured heterogeneous catalysts for N-cycle electrocatalysis, Nano Res. Energy, 2022, 1, e9120010 CrossRef.
- X. Li, P. Shen, Y. Luo, Y. Li, Y. Guo, H. Zhang and K. Chu, PdFe single-atom alloy metallene for N2 electroreduction, Angew. Chem., 2022, 134, e202205923 Search PubMed.
- X. Zhao, G. Hu, G. F. Chen, H. Zhang, S. Zhang and H. Wang, Comprehensive understanding of the thriving ambient electrochemical nitrogen reduction reaction, Adv. Mater., 2021, 33, 2007650 CrossRef CAS PubMed.
- K. Tanifuji and Y. Ohki, Metal–sulfur compounds in N2 reduction and nitrogenase-related chemistry, Chem. Rev., 2020, 120, 5194–5251 CrossRef CAS PubMed.
- K. Chu, Y. Luo, P. Shen, X. Li, Q. Li and Y. Guo, Unveiling the synergy of O-vacancy and heterostructure over MoO3−x/MXene for N2 electroreduction to NH3, Adv. Energy Mater., 2022, 12, 2103022 CrossRef CAS.
- H. Shen, C. Choi, J. Masa, X. Li, J. Qiu, Y. Jung and Z. Sun, Electrochemical ammonia synthesis: mechanistic understanding and catalyst design, Chem, 2021, 7, 1708–1754 CAS.
- Y. Ren, C. Yu, X. Tan, H. Huang, Q. Wei and J. Qiu, Strategies to suppress hydrogen evolution for highly selective electrocatalytic nitrogen reduction: challenges and perspectives, Energy Environ. Sci., 2021, 14, 1176–1193 RSC.
- Y. Li, H. Wang, C. Priest, S. Li, P. Xu and G. Wu, Advanced electrocatalysis for energy and environmental sustainability via water and nitrogen reactions, Adv. Mater., 2021, 33, 2000381 CrossRef CAS.
- X. Li, Y. Luo, Q. Li, Y. Guo and K. Chu, Constructing an electron-rich interface over an Sb/Nb2CTx-MXene heterojunction for enhanced electrocatalytic nitrogen reduction, J. Mater. Chem. A, 2021, 9, 15955–15962 RSC.
- Q. Li, J. Wang, Y. Cheng and K. Chu, Zn nanosheets: An earth-abundant metallic catalyst for efficient electrochemical ammonia synthesis, J. Energy Chem., 2021, 54, 318–322 CrossRef CAS.
- K. Chu, H. Nan, Q. Li, Y. Guo, Y. Tian and W. Liu, Amorphous MoS3 enriched with sulfur vacancies for efficient electrocatalytic nitrogen reduction, J. Energy Chem., 2021, 53, 132–138 CrossRef CAS.
- K. Chu, X. Li, Q. Li, Y. Guo and H. Zhang, Synergistic enhancement of electrocatalytic nitrogen reduction over boron nitride quantum dots decorated Nb2CTx-MXene, Small, 2021, 17, 2102363 CrossRef CAS PubMed.
- K. Chu, W. Gu, Q. Li, Y. Liu, Y. Tian and W. Liu, Amorphization activated FeB2 porous nanosheets enable efficient electrocatalytic N2 fixation, J. Energy Chem., 2021, 53, 82–89 CrossRef CAS.
- K. Chu, Y. Liu, J. Wang and H. Zhang, NiO nanodots on graphene for efficient electrochemical N2 reduction to NH3, ACS Appl. Energy Mater., 2019, 2, 2288–2295 CrossRef CAS.
- V. Kyriakou, I. Garagounis, E. Vasileiou, A. Vourros and M. Stoukides, Progress in the electrochemical synthesis of ammonia, Catal. Today, 2017, 286, 2–13 CrossRef.
- V. Kordali, G. Kyriacou and C. Lambrou, Electrochemical synthesis of ammonia at atmospheric pressure and low temperature in a solid polymer electrolyte cell, Chem. Commun., 2000, 1673–1674 RSC.
- T. Murakami, T. Nishikiori, T. Nohira and Y. Ito, Electrolytic synthesis of ammonia in molten salts under atmospheric pressure, J. Am. Chem. Soc., 2003, 125, 334–335 CrossRef CAS PubMed.
- G. Zhang, X. Li, P. Shen, Y. Luo, X. Li and K. Chu, PdNi nanosheets boost nitrate electroreduction to ammonia, J. Environ. Chem. Eng., 2022, 10, 108362 CrossRef CAS.
- K. Chu, Y. Cheng, Q. Li, Y. Liu and Y. Tian, Fe-doping induced morphological changes, oxygen vacancies and Ce3+–Ce3+ pairs in CeO2 for promoting electrocatalytic nitrogen fixation, J. Mater. Chem. A, 2020, 8, 5865–5873 RSC.
- K. Chu, Y. Liu, Y. Chen and Q. Li, Synergistic boron-dopants and boron-induce oxygen vacancies in MnO2 nanosheets to promote electrocatalytic nitrogen reduction, J. Mater. Chem. A, 2020, 8, 5200–5208 RSC.
- P. Shen, G. Wang, K. Chen, J. Kang, D. Ma and K. Chu, Selenium-vacancy-rich WSe2 for nitrate electroreduction to ammonia, J. Colloid Interface Sci., 2023, 629, 563–570 CrossRef CAS PubMed.
- K. Chu, Y. Liu, Y. Li, H. Zhang and Y. Tian, Efficient electrocatalytic N2 reduction on CoO quantum dots, J. Mater. Chem. A, 2019, 7, 4389–4394 RSC.
- Y. Luo, Q. Li, Y. Tian, Y. Liu and K. Chu, Amorphization engineered VSe2−x nanosheets with abundant Se-vacancies for enhanced N2 electroreduction, J. Mater. Chem. A, 2022, 10, 1742–1749 RSC.
- D. Qi, F. Lv, T. Wei, M. Jin, G. Meng, S. Zhang, Q. Liu, W. Liu, D. Ma, M. S. Hamdy, L. Jun and L. Xijun, High-efficiency electrocatalytic NO reduction to NH3 by nanoporous VN, Nano Res. Energy, 2022, 1, e9120022 CrossRef.
- J. Liang, P. Liu, Q. Li, T. Li, L. Yue, Y. Luo, Q. Liu, N. Li, B. Tang, A. A. Alshehri, I. Shakir, P. O. Agboola, C. Sun and X. Sun, Amorphous boron carbide on titanium dioxide nanobelt arrays for high-efficiency electrocatalytic NO reduction to NH3, Angew. Chem., Int. Ed., 2022, 61, e202202087 CAS.
- L. Zhang, J. Liang, Y. Wang, T. Mou, Y. Lin, L. Yue, T. Li, Q. Liu, Y. Luo, N. Li, B. Tang, Y. Liu, S. Gao, A. A. Alshehri, X. Guo, D. Ma and X. Sun, High-performance electrochemical NO reduction into NH3 by MoS2 nanosheet, Angew. Chem., Int. Ed., 2021, 60, 25263–25268 CrossRef CAS.
- P. Liu, J. Liang, J. Wang, L. Zhang, J. Li, L. Yue, Y. Ren, T. Li, Y. Luo, N. Li, B. Tang, Q. Liu, A. M. Asiri, Q. Kong and X. Sun, High-performance NH3 production via NO electroreduction over a NiO nanosheet array, Chem. Commun., 2021, 57, 13562–13565 RSC.
- B. He, P. Lv, D. Wu, X. Li, R. Zhu, K. Chu, D. Ma and Y. Jia, Confinement catalysis of a single atomic vacancy assisted by aliovalent ion doping enabled efficient NO electroreduction to NH3, J. Mater. Chem. A, 2022, 10, 18690–18700 RSC.
- P. Lv, D. Wu, B. He, X. Li, R. Zhu, G. Tang, Z. Lu, D. Ma and Y. Jia, An efficient screening strategy towards multifunctional catalysts for the simultaneous electroreduction of NO3−, NO2− and NO to NH3, J. Mater. Chem. A, 2022, 10, 9707–9716 RSC.
- G. Meng, T. Wei, W. Liu, W. Li, S. Zhang, W. Liu, Q. Liu, H. Bao, J. Luo and X. Liu, NiFe layered double hydroxide nanosheet array for high-efficiency electrocatalytic reduction of nitric oxide to ammonia, Chem. Commun., 2022, 58, 8097–8100 RSC.
- X. Peng, Y. Mi, H. Bao, Y. Liu, D. Qi, Y. Qiu, L. Zhuo, S. Zhao, J. Sun, X. Tang, J. Luo and X. Liu, Ambient electrosynthesis of ammonia with efficient denitration, Nano Energy, 2020, 78, 105321 CrossRef CAS.
- Y. Xiong, Y. Li, S. Wan, Y. Yu, S. Zhang and Q. Zhong, Ferrous-based electrolyte for simultaneous NO absorption and electroreduction to NH3 using Au/rGO electrode, J. Hazard. Mater., 2022, 430, 128451 CrossRef CAS.
- Y. Li, C. Cheng, S. Han, Y. Huang, X. Du, B. Zhang and Y. Yu, Electrocatalytic reduction of low-concentration nitric oxide into ammonia over Ru nanosheets, ACS Energy Lett., 2022, 7, 1187–1194 CrossRef CAS.
- Y. Lin, J. Liang, H. Li, L. Zhang, T. Mou, T. Li, L. Yue, Y. Ji, Q. Liu, Y. Luo, N. Li, B. Tang, Q. Wu, M. S. Hamdy, D. Ma and X. Sun, Bi nanodendrites for highly efficient electrocatalytic NO reduction to NH3 at ambient conditions, Mater. Today Phys., 2022, 22, 100611 CrossRef CAS.
- J. Liang, Q. Zhou, T. Mou, H. Chen, L. Yue, Y. Luo, Q. Liu, M. S. Hamdy, A. A. Alshehri, F. Gong and X. Sun, FeP nanorod array: A high-efficiency catalyst for electroreduction of NO to NH3 under ambient conditions, Nano Res., 2022, 15, 4008–4013 CrossRef CAS.
- Q. Liu, Y. Lin, L. Yue, J. Liang, L. Zhang, T. Li, Y. Luo, M. Liu, J. You, A. A. Alshehri, Q. Kong and X. Sun, Bi nanoparticles/carbon nanosheet composite: A high-efficiency electrocatalyst for NO reduction to NH3, Nano Res., 2022, 15, 5032–5037 CrossRef CAS.
- J. Liang, W.-F. Hu, B. Song, T. Mou, L. Zhang, Y. Luo, Q. Liu, A. A. Alshehri, M. S. Hamdy, L.-M. Yang and X. Sun, Efficient nitric oxide electroreduction toward ambient ammonia synthesis catalyzed by a CoP nanoarray, Inorg. Chem. Front., 2022, 9, 1366–1372 RSC.
- L. Zhang, Q. Zhou, J. Liang, L. Yue, T. Li, Y. Luo, Q. Liu, N. Li, B. Tang, F. Gong, X. Guo and X. Sun, Enhancing electrocatalytic NO reduction to NH3 by the CoS nanosheet with sulfur vacancies, Inorg. Chem. Front., 2022, 61, 8096–8102 CrossRef CAS.
- L. Ouyang, Q. Zhou, J. Liang, L. Zhang, L. Yue, Z. Li, J. Li, Y. Luo, Q. Liu, N. Li, B. Tang, A. Ali Alshehri, F. Gong and X. Sun, High-efficiency NO electroreduction to NH3 over honeycomb carbon nanofiber at ambient conditions, J. Colloid Interface Sci., 2022, 616, 261–267 CrossRef CAS PubMed.
- Z. Li, Z. Ma, J. Liang, Y. Ren, T. Li, S. Xu, Q. Liu, N. Li, B. Tang, Y. Liu, S. Gao, A. A. Alshehri, D. Ma, Y. Luo, Q. Wu and X. Sun, MnO2 nanoarray with oxygen vacancies: An efficient catalyst for NO electroreduction to NH3 at ambient conditions, Mater. Today Phys., 2022, 22, 100586 CrossRef CAS.
- C. Ling, X. Niu, Q. Li, A. Du and J. Wang, Metal-free single atom catalyst for N2 fixation driven by visible light, J. Am. Chem. Soc., 2018, 140, 14161–14168 CrossRef CAS.
- K. Chen, Y. Luo, P. Shen, X. Liu, X. Li, X. Li and K. Chu, Boosted nitrate electroreduction to ammonia on Fe-doped SnS2 nanosheet arrays with rich S-vacancies, Dalton Trans., 2022, 51, 10343–10350 RSC.
- K. Chu, J. Wang, Y. Liu, Q. Li and Y. Guo, Mo-doped SnS2 with rich S-vacancies for highly efficient electrocatalytic N2 reduction: the critical role of Mo-Sn-Sn trimer, J. Mater. Chem. A, 2020, 8, 7117–7124 RSC.
- K. Chu, Y. Liu, Y. Li, J. Wang and H. Zhang, Electronically coupled SnO2 quantum dots and graphene for efficient nitrogen reduction reaction, ACS Appl. Mater. Interfaces, 2019, 11, 31806–31815 CrossRef CAS PubMed.
- D. Yan, H. Li, C. Chen, Y. Zou and S. Wang, Defect engineering strategies for nitrogen reduction reactions under ambient conditions, Small Methods, 2018, 1800331 Search PubMed.
- G. Wang, P. Shen, Y. Luo, X. Li, X. Li and K. Chu, A vacancy engineered MnO2−x electrocatalyst promotes electroreduction of nitrate to ammonia, Dalton Trans., 2022, 51, 9206–9212 RSC.
- Y. Li, Y. Liu, J. Wang, Y. Guo and K. Chu, Plasma-engineered NiO nanosheets with enriched oxygen vacancies for enhanced electrocatalytic nitrogen fixation, Inorg. Chem. Front., 2020, 7, 455–463 RSC.
- Y. Cheng, X. Li, P. Shen, Y. Guo and K. Chu, MXene quantum dots/copper heterostructure for synergistically enhanced N2 electroreduction, Energy Environ. Mater., 2022 DOI:10.1002/eem2.12268.
- Q. Li, Y. Guo, Y. Tian, W. Liu and K. Chu, Activating VS2 basal planes for enhanced NRR electrocatalysis: the synergistic role of S-vacancies and B dopants, J. Mater. Chem. A, 2020, 8, 16195–16202 RSC.
- Y. Luo, P. Shen, X. Li, Y. Guo and K. Chu, Sulfur-deficient Bi2S3−x synergistically coupling Ti3C2Tx-MXene for boosting electrocatalytic N2 reduction, Nano Res., 2022, 15, 3991–3999 CrossRef CAS.
- P. Shen, X. Li, Y. Luo, Y. Guo, X. Zhao and K. Chu, High-efficiency N2 electroreduction enabled by Se-vacancy-rich WSe2−x in water-in-salt electrolytes, ACS Nano, 2022, 16, 7915–7925 CrossRef CAS.
- P. Shen, X. Li, Y. Luo, N. Zhang, X. Zhao and K. Chu, Ultra-efficient N2 electroreduction achieved over a rhodium single-atom catalyst (Rh1/MnO2) in water-in-salt electrolyte, Appl. Catal., B, 2022, 316, 121651 CrossRef CAS.
- Q. Li, P. Shen, Y. Tian, X. Li and K. Chu, Metal-free BN quantum dots/graphitic C3N4 heterostructure for nitrogen reduction reaction, J. Colloid Interface Sci., 2022, 606, 204–212 CrossRef CAS.
- Y. Luo, K. Chen, P. Shen, X. Li, X. Li, Y. Li and K. Chu, B-doped MoS2 for nitrate electroreduction to ammonia, J. Colloid Interface Sci., 2023, 629, 950–957 CrossRef CAS.
- K. Chu, Q. Li, Y. Cheng and Y. Liu, Efficient electrocatalytic nitrogen fixation on FeMoO4 nanorods, ACS Appl. Mater. Interfaces, 2020, 12, 11789–11796 CrossRef CAS.
- D. Ma, Z. Zeng, L. Liu and Y. Jia, Theoretical screening of the transition metal heteronuclear dimer anchored graphdiyne for electrocatalytic nitrogen reduction, J. Energy Chem., 2021, 54, 501–509 CrossRef CAS.
- D. Wu, B. He, Y. Wang, P. Lv, D. Ma and Y. Jia, Double-atom catalysts for energy-related electrocatalysis applications: a theoretical perspective, J. Phys. D: Appl. Phys., 2022, 55, 203001 CrossRef.
- K. Chu, X. Li, Y. Tian, Q. Li and Y. Guo, Boron nitride quantum dots/Ti3C2Tx-MXene heterostructure for efficient electrocatalytic nitrogen fixation, Energy Environ. Mater., 2022, 5, 1303–1309 CrossRef CAS.
- W. Gu, Y. Guo, Q. Li, Y. Tian and K. Chu, Lithium iron oxide (LiFeO2) for electroreduction of dinitrogen to ammonia, ACS Appl. Mater. Interfaces, 2020, 12, 37258–37264 CrossRef CAS PubMed.
- K. Chu, Y. Liu, Y. Li, Y. Guo and Y. Tian, Two-dimensional (2D)/2D interface engineering of MoS2/C3N4 heterostructure for promoted electrocatalytic nitrogen fixation, ACS Appl. Mater. Interfaces, 2020, 12, 7081–7090 CrossRef CAS.
- S. Javad and W. Guoxiu, Progress and prospects of two-dimensional materials for membrane-based osmotic power generation, Nano Res. Energy, 2022, 1, e9120008 CrossRef.
- L. Lulu, H. Israr Masood ul Farwa, H. Ruinan, P. Luwei, X. Nengneng, N. Nabeel Khan, Z. Jia-Nan and Q. Jinli, Copper as a single metal atom based photo-, electro-, and photoelectrochemical catalyst decorated on carbon nitride surface for efficient CO2 reduction: A review, Nano Res. Energy, 2022, 1, e9120015 Search PubMed.
- A. Touqeer, L. Shuang, S. Muhammad, L. Ke, A. Mohsin, L. Liang and C. Wei, Electrochemical CO2 reduction to C2+ products using Cu-based electrocatalysts: A review, Nano Res. Energy, 2022, 1, e9120021 CrossRef.
- Y. Guo, Y. Cheng, Q. Li and K. Chu, FeTe2 as an earth-abundant metal telluride catalyst for electrocatalytic nitrogen fixation, J. Energy Chem., 2021, 56, 259–263 CrossRef CAS.
- Y. Wang, M. Wang, Z. Lu, D. Ma and Y. Jia, Enabling multifunctional electrocatalysts by modifying the basal plane of unifunctional 1T-MoS2 with anchored transition metal single atoms, Nanoscale, 2021, 13, 13390–13400 RSC.
- Y. Wang, D. Wu, P. Lv, B. He, X. Li, D. Ma and Y. Jia, Theoretical insights into the electroreduction of nitrate to ammonia on graphene-based single-atom catalysts, Nanoscale, 2022, 14, 10862–10872 RSC.
- D. Ma, Y. Wang, L. Liu and Y. Jia, Electrocatalytic nitrogen reduction on the transition-metal dimer anchored N-doped graphene: performance prediction and synergetic effect, Phys. Chem. Chem. Phys., 2021, 23, 4018–4029 RSC.
|
This journal is © the Partner Organisations 2023 |
Click here to see how this site uses Cookies. View our privacy policy here.