DOI:
10.1039/D2QI02217F
(Research Article)
Inorg. Chem. Front., 2023,
10, 93-107
Upconversion fluorescence-based PDT nanocomposites with self-oxygenation for malignant tumor therapy
Received
17th October 2022
, Accepted 14th November 2022
First published on 19th November 2022
Abstract
Photodynamic therapy (PDT) has made significant progress in tumor treatment, but it is still limited by poor light penetration and the imbalance between the hypoxic microenvironment of tumors and oxygen consumption during PDT, tremendously lowering the tumor removal efficiency. Herein, a high-efficiency PDT nano-system with high tissue penetration and oxygen-self-generation is presented for solving the hypoxic problem and enhancing the tumor therapeutic efficacy. By encapsulating chlorin e6 and upconversion nanoparticles (UCNPs) in an ultrathin silane (UCNPs-Ce6@Silane), an almost pure upconverted red light is obtained via NIR excitation and the red light further excites Ce6 molecules to exert PDT for effective tumor therapy. In this process, the NIR light could penetrate deeper into the tumor tissue, endowing this procedure with a better biological effect. In view of the tumor hypoxic microenvironment, a MnO2 nanozyme was further modified on the surface of UCNPs-Ce6@Silane NPs, which could catalyze the excessive hydrogen peroxide in the tumor and provide sufficient oxygen for PDT. From the experiments in the cellular level and animal models, it could be proved that the brilliant composite nanoplatform could exhibit remarkable self-oxygenation properties, sufficient ROS production and distinguished tissue penetration. The prominent results indicated that this design would be a hopeful solution in the tumor therapeutics.
1. Introduction
Malignant cancer remains the most devastating disease and affects the quality of life and survival of patients.1 Till date, extensive research has been made for the purpose of improving the efficacy and prognosis. In spite of the sustained progress, the clinical effects of these treatments are still limited by some factors such as tumor microenvironment.2 Therefore, it is meaningful to develop a highly effective and biosecurity therapeutic strategy, which could utilize a single method to ablate tumor tissues thoroughly.
Distinct from the conventional cancer treatment, photodynamic therapy (PDT) is an important and approved therapeutic strategy with the characteristic of non-invasiveness and low side effects.3 When endogenous or exogenous photosensitizers in biological tissues are illuminated by the corresponding wavelength, they absorb photon energy and change from the ground state to the excited state, which is very unstable, and they quickly release energy and produce reactive oxygen species (ROS).4 In addition, ROS could interact with a variety of biological macromolecules, damage the cell structure or affect cell functions, and lead to tumor cell death fatefully.5–7 Among many photosensitizer molecules, chlorin e6 is a very well-known photosensitizer that could be excited by a red light to achieve efficient PDT performance.8 Many research groups, including us, have developed a series of PDT strategies based on Ce6 photosensitizers. To improve the cyclic stability and release efficiency of Ce6 photosensitizers in the PDT process, Li et al. prepared novel hyperbranched polyphosphoester (hbPPE) containing numerous acetal bonds (S-hbPPE/Ce6), which was explored as a chlorin e6 (Ce6) nanocarrier for PDT,9 and this nanocarrier shows great potential to improve the therapeutic effect of PDT. Liu et al. coupled biotin with Ce6 and developed a new tumor-targeting photosensitizer Ce6–biotin,10 which showed improved tumor-targeting property. Zhang et al. loaded curcumin and Ce6 into PLGA NPs and then wrapped them with MCF-7 cell membranes (MCNPs).11 While increasing the uptake of nanocarriers by homologous cells, the combination of PDT and chemotherapy synergistically enhanced the inhibition of MCF-7 cell proliferation. In our prior research study, we designed a composite structure based on Fe3O4 and Ce6 to achieve PDT-killing tumor cells,12 and also applied this strategy to the treatment of inflammation either.13
For the Ce6 photosensitizers, red light as the excitation light source has a relatively large tissue penetration depth and plays an important role in biological application therapy.14 However, for the needs of clinical practical applications, the working depth of its light source needs to be further improved. Near-infrared (NIR) light is well known as a biological window with a large tissue penetration depth of almost 5–10 mm, which could enable treatment deeper into the tumor when irradiating the tumor site and avoid the invasiveness of optical fiber implantation under deep tissue penetration.15 Compared with pure red light alone, it has more advantages in the treatment of malignant tumors. How can the near-infrared light be utilized in PDT and achieve deeper penetration is the first key problem. Upconversion fluorescence is a luminescence mode that converts near-infrared light into visible light and even UV light, and the most famous ones with this function are rare earth upconversion nanoparticles (UCNPs).16,17 UCNPs could absorb two or more low-frequency photons and emit one high-frequency photon. Because the photon energy absorbed by it is lower than the photon energy emitted, it violates the classical Stokes law, so it is also called anti-Stokes law luminous material. Rare-earth doped NaYF4 upconversion phosphors have become a research hotspot in recent years due to their excellent chemical and luminescence properties including low phonon energy, narrow emission peak, long fluorescence lifetime, strong photostability, and low toxicity.18 Our previous work about UCNP@TiO2 composites could be an example of how the PDT function of TiO2 in the outer layer was triggered via NIR-to-UV upconversion luminescence by exciting inner UCNPs, achieving efficient PDT performance for antibacterial applications.19 On account of this research, we provide the thought to introduce the upconversion capability into the PDT system, convert the near-infrared light into red light and excite the excellent photosensitizer Ce6, in order to achieve the Ce6-based PDT therapy in deep tissues.
Oxygen content is an important factor in achieving efficient treatment during PDT since oxygen is the raw material to form radicals.20 In principle, the key internal factor of the photodynamic efficiency is oxygen, which is required as a raw material for PDT.21 PDT not only could kill the tumor cell without side effects, but could also induce the hypoxia problem. In the solid tumor environment, hypoxia is a key hallmark that is caused by the abnormal proliferation and dysfunctional angiogenesis of tumor cells.22 In addition, the oxygen consumption by PDT would further deteriorate the problem of its imbalance with the hypoxic environment. In this vicious circle, the imbalance of insufficient oxygen supply and aberrant oxygen consumption would inevitably affect the anti-tumor efficiency of PDT. Therefore, how to solve the key problem of hypoxia is of important in PDT applications.23,24
Hydrogen peroxide (H2O2) was admittedly regarded as the outstanding supplement source of molecular oxygen. Compared with other ROS (half-life < 1 μs), H2O2 (half-life about 1 ms) is relatively stable.25 In addition, H2O2, as an extracellular and intracellular signal molecule, mediates various effects in biological systems. It has been found that the H2O2 production rate of cancer cells was increased (up to 0.5 nmol per 104 cells per h) compared with normal cells,20 resulting in higher H2O2 levels in tumors than in normal tissues. Therefore, in addition to its important role in cell signaling, overproduced H2O2 has been developed as the main precursor of highly active ROS. Importantly, it has been found that under certain conditions, H2O2 accumulated in tumors can be decomposed into O2, which is used to supplement O2 required for PDT.25 Therefore, the introduction of enzymes that could catalyze the decomposition of hydrogen peroxide to generate oxygen can be an important strategy to improve the efficacy of PDT.26,27
In general, PDT efficacy and the tumor microenvironment are mutually related, and at the same time, mutually restrictive. On the one side, the hypoxia could be further attributed to the poor PDT efficacy, and on the other side, the local hypoxia microenvironment would deteriorate the tumor local immunity and ulteriorly reduce the curative effect of PDT. Based on the above-mentioned issues, it is important to develop a singlet component nanoplatform capable of generating oxygen and ROS for PDT simultaneously and efficiently. In this study, we designed a composite PDT nanoplatform integrating NIR light-triggered photosensitizers and nano enzymes for self-oxygenation function. In this nanoplatform, the photosensitizer Ce6 molecules were encapsulated within an ultrathin silane along with UCNPs to realize the nano-functional design, and MnO2 enzymes were further modified on the surface of UCNPs-Ce6@Silane. Under the 980 nm red light irradiation, the NaErF4:0.5%Tm@NaYF4:20%Yb core/shell UCNPs could play the role of converting NIR into 630 nm red light and excite the Ce6 molecules to produce a mass of ROS instantaneously. At the same time, the MnO2 enzyme could catalyze the production of oxygen from hydrogen peroxide and relieve the hypoxia microenvironment to form a positive cycle in the tumor therapeutics. This PDT-based nanoplatform provides valuable exploration for tumor treatment.
2. Experimental section
2.1. Materials
Erbium(III) acetate hydrate (99.9% trace metals basis), thulium(III) acetate hydrate (99.9% trace metals basis), yttrium(III) acetate hydrate (99.9% trace metals basis), ytterbium(III) acetate hydrate (99.9% trace metals basis), oleic acid (technical grade, 90%), octadecene (technical grade, 90%), sodium hydroxide, ammonium fluoride (99.9% trace metals basis), methanol, cyclohexane (anhydrous, 99.5%), trimethoxyoctadecylsilane, chlorin e6, tetrahydrofuran solvent, 1,3-diphenylisobenzofuran (BPDF), and Cell Counting Kit-8 (CCK-8) were obtained from Beijing Solarbio. Fetal bovine serum (FBS) was obtained from Tianjin kangyuan Biotechnology Co., Ltd. 2′,7′-Dichlorodihydrofluorescein diacetate (DCFH-DA) was purchased from Sigma. Calcein-AM/PI (BestBio) was used in our work without further purification. All chemicals were directly used without further purification.
2.2. Synthesis of core NaErF4:0.5%Tm NPs
NaErF4:0.5%Tm NPs were synthesized by thermolysis of the respective lanthanide precursors.28,29 In a typical synthesis of 1 mmol NPs, erbium(III) acetate (0.995 mmol) and thulium(III) acetate (0.005 mmol) were mixed with 8 mL oleic acid and 14 mL octadecene in a 100 mL three-necked flask. The mixture was heated to 130 °C for 1 h under nitrogen protection with constant stirring to remove the moisture and form a clear lanthanide oleate complex solution and then cooled down to room temperature. After that, 5–6 mL of methanol solution containing NH4F (4 mmol) and NaOH (2.5 mmol) was added dropwise to the three-necked flask under stirring for another 30 min. Next, the mixture solution was heated to 80 °C for almost half an hour and then was increased to 125 °C and maintained at this temperature for a few minutes to remove methanol. The absence of bubbles from the reaction mixture was used as a marker for the complete removal of methanol. After methanol was evaporated, the solution was heated to 300 °C for 1.5 h with nitrogen to form the thermally stable beta phase (hexagonal structure of NaErF4) of nanoparticles (NPs). When the reaction was completed, the solution was cooled down naturally to room temperature. The particles were precipitated by the addition of an appropriate amount of ethanol, collected by centrifugation at 8000 rpm for 15 min, washed with absolute ethanol-cyclohexane, and finally redispersed in 10 mL of cyclohexane for further experiments.
2.3. Synthesis of core@active shell NaErF4:0.5%Tm@NaYF4:20%Yb NPs (core–shell UCNPs)
First, 20 at% Yb3+ ions in ytterbium(III) acetate were used along with 80 at% Y3+ ions in yttrium(III) acetate as metal precursors to make the reaction mixture.30 In a typical synthesis of 1 mmol NPs, 0.8 mmol yttrium(III) acetate and 0.2 mmol ytterbium(III) acetate were mixed with 8 mL oleic acid and 14 mL octadecene in a 100 mL three-necked flask. In this experiment, when the ratio of core to shell is 1 to 0.8, the contents of yttrium and ytterbium are relatively reduced. The contents were first flushed with nitrogen and then heated at 130 °C for 1 h. The mixture was then cooled down to room temperature. Subsequently, 5–6 mL methanol solution containing NH4F (4 mmol) and NaOH (2.5 mmol) was added into the three-necked flask dropwise and then heated to 125 °C for 30 min to remove the methanol. After methanol was evaporated, the solution was cooled down to room temperature. The reaction mixture was added to the already prepared core NPs dispersed in cyclohexane as seeds (10 mL). Cyclohexane was first evaporated at 90 °C and then heated to 130 °C to completely remove cyclohexane. After the cyclohexane was evaporated, the solution was heated to 300 °C for 1.5 h to form the beta phase of core@active shell NPs under nitrogen flow. When the reaction was completed, the solution was cooled down to room temperature. The resulting NPs were precipitated by the addition of ethanol, collected by centrifugation at 8000 rpm for 15 min.
2.4. Synthesis of NaErF4:0.5%Tm@NaYF4:20%Yb-Ce6@Silane (UCNPs-Ce6@Silane)
In this experiment, trimethoxyoctadecyl silane was used to cover the coating. NaErF4:0.5%Tm@NaYF4:20%Yb-Ce6@Silane composites were synthesized as follows: first, the silane was dissolved in tetrahydrofuran according to the mass ratio of silane/UCNPs of 1/2.15 After that, Ce6 molecules were added to the mixed solution of UCNPs and silane in a certain ratio, and mixed under ultrasonic conditions for at least half an hour. Then, the mixed solution was quickly injected into ammonia water with a pH of 9. After 2–4 hours of the hydrolysis reaction, it was transferred into a dialysis bag (a molecular weight cutoff of 8000–14
000) for dialysis. The resulting upconversion composite not only has the function of up-converting fluorescent labeling, but also has PDT function.
2.5. Synthesis of UCNPs-Ce6@Silane@MnO2 composites (UCNPs-Ce6@Silane@Mn)
First, multifunctional UCNPs-Ce6@Silane NPs were prepared according to our previous report. Subsequently, 60 μL of KMnO4 solution (0.1 M) was mixed with 5 mL UCNPs-Ce6@Silane solution followed by 15 min sonication.31 Afterwards, 60 μL of MnSO4·H2O (0.15 M) was added to the aforementioned solution with continuous sonication for 1 h. The obtained sample was dialyzed overnight to get the final product UCNPs-Ce6@Silane@Mn.
2.6. Measurement of singlet oxygen generation
The DPBF was selected as a chemical singlet oxygen probe.32 To confirm the enhanced ROS generation by UCNPs-Ce6@Silane@Mn composites, the H2O2, UCNPs-Ce6@Silane, UCNPs-Ce6@Silane@Mn, UCNPs-Ce6@Silane + H2O2 and UCNPs-Ce6@Silane@Mn + H2O2 aqueous solution (with the equivalent concentration of Ce6) was respectively mixed with DPBF. The mixed solutions were triggered by light irradiation (980 nm, 4 W cm−2) every 1 min, followed by recording the UV-vis absorption spectra at 425 nm.
2.7. Cell culture and cytotoxicity assay
The MCF-7 cells and B16 cells were cultured in a DMEM medium in an incubator at 37 °C under 5% CO2.33,34 The cells were supplemented with 1% penicillin–streptomycin (100 U mL−1 penicillin and 100 g mL−1 streptomycin) and 10% fetal bovine serum (FBS, Clark). Then, MCF-7 cells and B16 cells were seeded in 96-well plates at a density of 1 × 104 per well for 24 h and then incubated with different concentrations of UCNPs-Ce6@Silane or UCNPs-Ce6@Silane@Mn for another 24 h. The standard CCK8 assay was performed to determine the cytotoxicity using a microplate reader (Bio-Tek ELX800, USA).
2.8. Cell death and viability staining and singlet oxygen determination
Calcein-AM/PI stain experiment was applied to observe live and dead cells.35 The density of MCF-7/B16 cells was 2 × 105 per well seeded in 24-well plates for 24 h. Then, cells were incubated with a FBS-free culture medium and different NPs (UCNPs-Ce6@Silane, UCNPs-Ce6@Silane@Mn and Ce6). After 4 h, all culture dishes were treated with 980 nm laser (4 W cm−2) irradiation and further incubated for 2 h. Then, after removing the culture medium, Calcein-AM (0.3 μg mL−1, 1 mL) was added into culture dishes to incubate for another 20 min. After removing Calcein-AM, PI (10 ng mL−1, 1 mL) was added into culture dishes to incubate for 5 min. Then, all dishes were washed with PBS (pH 7.4) for three times and observed using a confocal laser scanning fluorescence microscope (CLSM; FV1200, Olympus Japan). Subsequently, we also evaluated the ROS generation by UCNPs-Ce6@Silane@Mn composites at a cellular level. MCF-7/B16 cells were incubated with different NPs (UCNPs-Ce6@Silane, UCNPs-Ce6@Silane@Mn and Ce6). After laser irradiation for 3 min, the intracellular ROS generation was measured by the ROS-sensitive fluorescence probe DCFH-DA using a small animal imaging system and finally imaged using a CLSM.
2.9. Characterization
The transmission electron microscopic (TEM) and high-resolution TEM (HR-TEM) images were recorded using a Hitachi H-8100IV high-resolution transmission electron microscope. The absorption wavelengths were tested using a Shimadzu UV-3600. The phase structure of UCNPs was measured using a RigakuTTR III X-ray power diffractometer (XRD). The cytotoxicity of the sample was analyzed using a microplate reader (Bio-Tek ELX800, USA).
2.10.
In vivo antitumor therapy
C57 mice (15–17 g, female) were commercially obtained and used in accordance with the protocols approved by the Committee for Animal Research of Jilin University. After observation for about 1 week, 0.1 mL of B16 cells (1 × 107 cells per mL) were subcutaneously injected into the right hind leg of C57 mice (18–20 g body weight).36 When the B16 tumor reached a size of 100 mm3, the B16 tumor-bearing mice were apportioned to different groups as follows: (1) control (laser only), (2) Ce6 + Laser, (3) UCNPs-Ce6@Silane + laser, and (4) UCNPs-Ce6@Silane@Mn + laser. After 2 h injection, the tumors of injected mice were exposed to a 980 nm laser at 4 W cm−2 for 5 min. The tumor size was monitored every day using a vernier caliper. The tumor volume (V) was calculated according to the following formula: V = L × W2/2, where L and W are the length and width of the tumor, respectively. The tumor tissues and internal organs of different groups of mice were analyzed by histological examination. All specimens were stained with hematoxylin and eosin (H&E) and then observed using an optical microscope. Tumor tissues of mice in different groups were examined and analyzed by immunohistochemistry, and all specimens were subjected to immunohistochemistry with HIF-1α and S100 antibodies and then observed using an optical microscope.
3. Results and discussion
3.1. Synthesis and characterization of UCNPs-Ce6@Silane@Mn composites
The synthesis scheme of UCNPs-Ce6@Silane@Mn is shown in Fig. 1. First, core@active shell UCNPs (NaErF4:0.5%Tm@NaYF4:20%Yb NPs) were synthesized by an improved solvothermal method. The PDT absorption site of the Ce6 molecule is in the red region, so monochromatic strong red upconversion fluorescence is very important. In order to produce the single-band red color, we prepared monodisperse Tm3+-doped NaErF4 NPs (NaErF4:0.5%Tm), in which Tm3+ ions can be used as energy capture centers.30 Besides, the core–shell formation strategy was performed to achieve NaErF4:0.5%Tm@NaYF4:20%Yb nanocrystals, greatly improving the luminescence intensity. Then, we prepared the composite NPs combining Ce6 molecules and UCNPs by an amphiphilic silane modification strategy. Note that the amphiphilic silane modification technology involves the hydrophobic–hydrophobic interaction between the hydrophobic side chain of the silane and the hydrophobic group on the surface of UCNPs.37 In the UCNPs-Ce6@Silane composites, after hydrolysis, the silane forms a very thin hydrophilic coating, which means that the hydrophobic UCNPs were transformed into hydrophilic ones without increasing the size. In this process, the hydrophobic chain of the silane is hinged with the hydrophobic ligand on the surface of UCNPs, and the hydrophilic part faces outward to form a hydrophilic surface structure. Another advantage of silanes is forming a hydrophobic space that can be used to load Ce6 molecules. When UCNPs enter cells, they can be excited by 980 NIR light to emit strong red light, and Ce6 molecules in the hydrophobic layer can be excited by the upconversion red emission to realize PDT. The product NaErF4:0.5%Tm@NaYF4:20%Yb-Ce6@Silane is referred to as “UCNPs-Ce6@Silane”. The solution to the hypoxia problem is the feature of our work. In the hypoxic microenvironment, converting H2O2 into oxygen (O2) should be a very effective strategy, and it is reported that low toxic manganese dioxide (MnO2) can react with a low concentration of hydrogen peroxide, and produce oxygen to overcome tumor hypoxia.38–40 Therefore, on the basis of UCNPs-Ce6@Silane, we further introduced the MnO2 nanozyme to achieve self-oxygenation and improve the performance of PDT by coating a MnO2 nano layer onto the amphiphilic silane-modified multifunctional UCNPs.31 After the redox reaction between KMnO4 and MnSO4 in an aqueous solution, the final product NaErF4:0.5%Tm@NaYF4:20%Yb-Ce6@Silane@MnO2 was achieved and referred to as “UCNPs-Ce6@Silane@Mn”.
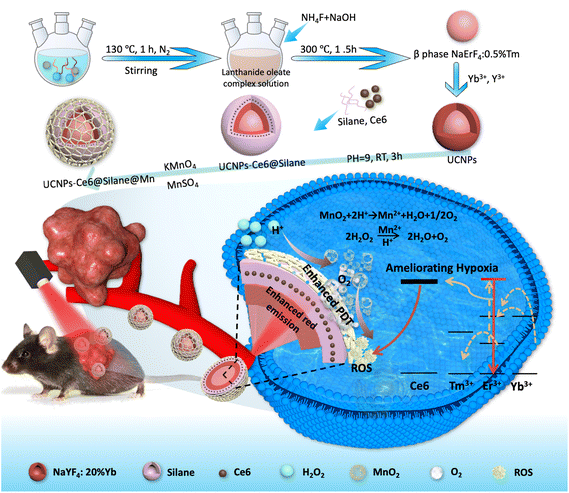 |
| Fig. 1 Illustration of the UCNPs-Ce6@Silane@Mn nanocomposite in the preparation and mechanism for tumor treatment. The core@active shell UCNPs (NaErF4:0.5%Tm@NaYF4:20%Yb NPs) were synthesized by an improved solvothermal method, and monodisperse Tm3+ was doped in order to produce the single-band red color. Then, Ce6 molecules were loaded by a amphiphilic silane modification strategy. On the basis of UCNPs-Ce6@Silane, we further coated a MnO2 nano layer onto the amphiphilic silane-modified multifunctional UCNPs. When UCNPs enter cells, they could be excited by 980 NIR light to emit strong red light, and Ce6 molecules in the hydrophobic layer could be excited by the upconversion red emission to realize PDT. Simultaneously, the MnO2 enzyme could catalyze the production of oxygen from hydrogen peroxide, improve the performance of PDT and relieve the hypoxia microenvironment, which could form a positive cycle in the tumor therapeutics. | |
The TEM images of core NaErF4:0.5%Tm, NaErF4:0.5%Tm@NaYF4:20%Yb, UCNPs-Ce6@Silane and UCNPs-Ce6@Silane@Mn are shown in Fig. 2(A–D). Fig. 2(E–H) are the particle size diagrams corresponding to Fig. 2(A–D) respectively. It can be seen that the particle size distribution of these four particles is very uniform. After the active shell NaYF4 was doped with 20% Yb ion layer modification, the size of NPs increased from 14.57 ± 0.98 nm to 17.58 ± 0.57 nm, while the shape of the NPs barely changed. After silane coating, a thin silane layer with a thickness of about 2–3 nm could be seen on the surface of the particles. As shown in Fig. 2G, the particle size of NPs UCNPs-Ce6@Silane is 20.38 ± 0.93 nm. The silane layer was successfully coated onto the core–shell UCNPs. Then, the MnO2 layer with a size of 4–5 nm was further modified on the silane layer. When a single nanoparticle is selected and characterized by HR-TEM, the thin layer of MnO2 on the surface can be clearly examined and the distinctive lattice fringes of MnO2 could be observed, as indicated by the yellow arrow in Fig. 2D. It can be clearly seen from the particle size diagram in Fig. 2 that the increase in particle size is due to different degrees of coating on the particle surface: core–shell coating, silane modification, and manganese dioxide growth, which preliminarily confirms the successful preparation of the materials.
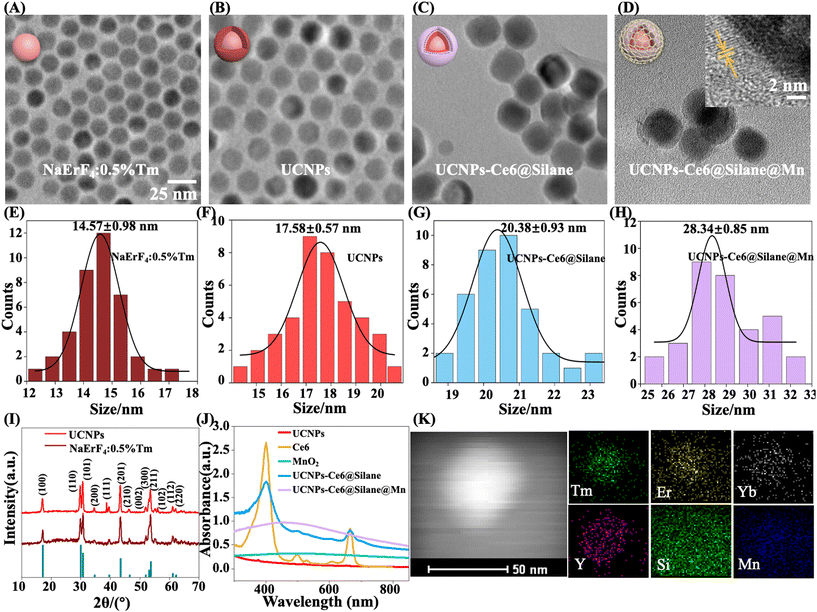 |
| Fig. 2 TEM images of (A) NaErF4:0.5%Tm, (B) NaErF4:0.5%Tm@NaYF4:20%Yb, (C) UCNPs-Ce6@Silane, and (D) UCNPs-Ce6@Silane@Mn and HR-TEM image of local area of single UCNPs-Ce6@Silane@Mn; the particle size diagrams corresponding to (A–D) respectively (E–H); (I) the XRD patterns of UCNPs-Ce6@Silane@Mn; (J) UV-vis absorption spectrum of Ce6, MnO2, NaErF4:0.5%Tm@NaYF4:20%Yb, UCNPs-Ce6@Silane and UCNPs-Ce6@Silane@Mn; (K) corresponding EDS elemental mappings (Tm, Er, Yb, Y, Si and Mn) of a single particle. | |
The XRD patterns of core β-NaErF4:0.5%Tm and core@active shell β-NaErF4:0.5%Tm@NaYF4:20%Yb NPs are shown in Fig. 2I. From the XRD patterns, it can be observed that the hexagonal phase structure of β-NaErF4:0.5%Tm matches well with the thermodynamically stable β-NaErF4 phase reported in JCPDS file no. 27-0689.9, suggesting high crystallinity.41Fig. 2J shows the absorption spectra of Ce6, MnO2, NaErF4:0.5%Tm@NaYF4:20%Yb, UCNPs-Ce6@Silane and UCNPs-Ce6@Silane@Mn. It can be seen from the data that the absorption value of UCNPs-Ce6@Silane almost completely overlaps with the absorption band of Ce6 molecules. After UCNPs-Ce6@Silane is combined with MnO2, a wide and long absorption peak appears in the 300–600 nm band, indicating that the absorption value of particles is greatly affected by MnO2. It is also possible that the absorption band of Ce6 is covered by MnO2 due to the low content of Ce6. Fig. 2K demonstrates the corresponding elemental mappings of Tm, Er, Yb, Y, Si, and Mn of a single particle, indicating the spatial distribution of elements, which further supports the successful preparation of UCNPs-Ce6@Silane@Mn according to the structure design.
3.2. Energy transfer mechanism of UCNPs-Ce6@Silane@Mn
The upconversion emission spectra of different NPs under 980 nm excitation are shown in Fig. 3A. As can be seen from the diagram, the brightness of the Tm-doped core structure increases compared with that of NaErF4 alone, and the intensity of the Tm-doped core–shell structure increases about 32 times after coating the shell with NaYF4:20%Yb. In addition, we chose Tm3+ ions doped at a concentration of 0.5%, according to the literature, since 0.5% is the best doping concentration to increase its red luminescence intensity.42,43 After loading the photosensitizer Ce6 and growing a layer of MnO2 on the surface of the composites, the luminescence intensity was significantly decreased compared with that of core–shell UCNPs, as shown in Fig. 3B.
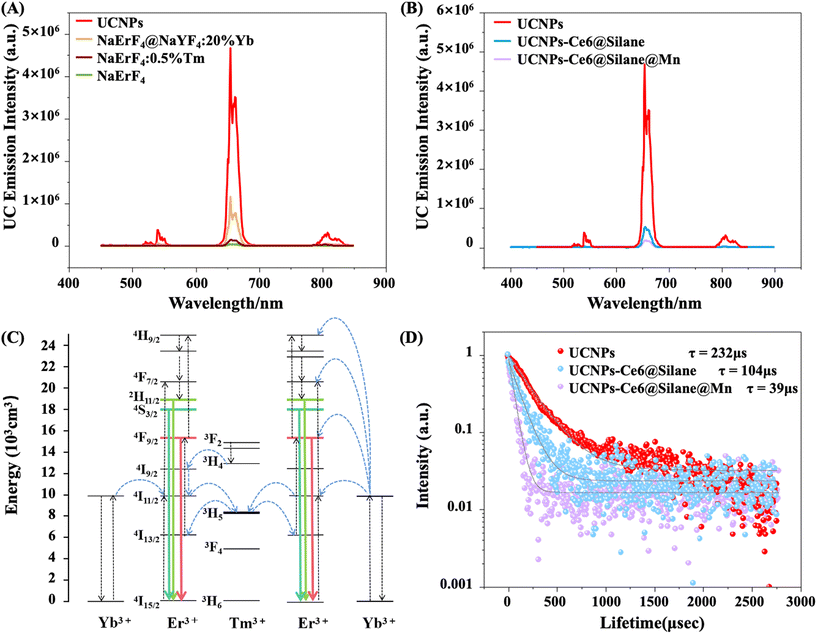 |
| Fig. 3 (A) Upconversion emission spectra of NaErF4, NaErF4:0.5%Tm, NaErF4@NaYF4:20%Yb, NaErF4:0.5%Tm@NaYF4:20%Yb. (B) Upconversion emission spectra of NaErF4:0.5%Tm@NaYF4:20%Yb, UCNPs-Ce6@Silane and UCNPS-Ce6@Silane@Mn. (C) Energy-level diagram depicting energy transfer processes. (D) Decay curves of luminescence lifetime of Er3+ at its 4F9/2 state in NaErF4:0.5%Tm@NaYF4:20%Yb, UCNPs-Ce6@Silane and UCNPs-Ce6@Silane@Mn monitored at 655 nm emission under 980 nm pulse laser excitation. | |
The energy transfer mechanism is shown in Fig. 3C. Note that the absorption cross-section of Er3+ ions is narrow, leading to weak emission intensity, and, in addition, the high cross-relaxation of Er3+ can also cause the luminescence quenching effect. When UCNPs were doped with a very small amount (0.5%) of Tm3+, the luminescence intensity of Er3+ ions is enhanced significantly, because Tm3+ ions can play the role of energy-trapping centers and restrict the excited photon energy of Er3+ to the core region through the reverse energy transfer (Er3+–Tm3+–Er3+) process.44 When the active NaYF4:20%Yb coating shell is involved, the 4I11/2 energy level of Er3+ is filled by the energy transfer from Yb3+ to Er3+ ions since Yb3+ ions have larger absorption cross sections than that of Er3+ ions at 980 nm. The NaErF4 host matrix facilitates the trapping of the populated photons to the 3H5 levels of Tm3+. Subsequently, the back-energy transfer occurs from the 3H5 level of Tm3+ into the 4I13/2 level of Er3+, and the 4I13/2 level of Er3+ can be further pumped to the 4F9/2 state with a NIR photon resulting in a strong red band emission at ∼655 nm during the involved upconversion process.
The involved energy transfer mechanism for core–shell UCNPs, UCNPs-Ce6@Silane and UCNPs-Ce6@Silane@Mn can be supported by lifetime decay analysis of the 4F9/2 level of Er3+ (Fig. 3D). The decay times of 4F9/2 levels at 655 nm are monitored for core–shell UCNPs, UCNPs-Ce6@Silane as well as UCNPs-Ce6@Silane@Mn. The lifetime values of core–shell UCNPs, UCNPs-Ce6@Silane and UCNPs-Ce6@Silane@Mn by mono-exponential fitting are 232, 104 and 39 μs, respectively. With the coating of silane and MnO2, the luminescence intensity of the samples decreases and the lifetime decreases in turn. These results indicate the occurrence of energy transfer from Er ions to Ce6 molecules.
3.3. Oxygen generation of UCNPs-Ce6@Silane@Mn composites
In addition to improving the luminous intensity of nano materials, solving the hypoxia problem of tumor microenvironment is also an important innovation in this work. In UCNPs-Ce6@Silane@Mn, the MnO2 layer has strong nano-enzyme catalytic ability for the decomposition of H2O2 to O2, which was desirable for tumor disease therapy. The sufficient O2 production can effectively improve the tumor hypoxic microenvironment and provide the oxygen source for enhancing the ROS production (Fig. 4A). To verify the upconversion PDT function of UCNPs-Ce6@Silane@Mn, ROS generation was tested using the singlet oxygen probe DPBF. Fig. 4B shows the absorption spectra of UCNPs-Ce6@Silane@Mn composite nanomaterials solution mixed with hydrogen peroxide under red light with an interval of 1 min. With the increase in irradiation time, the absorption value of DPBF at 425 nm is decreased, indicating the efficient generation of singlet oxygen. Fig. 4C describes the change in singlet oxygen generation by comparing the decrease in absorbance at 425 nm of different NPs in DPBF. Compared with pure H2O2 solution, the DPBF absorbances in UCNPs-Ce6@Silane and UCNPs-Ce6@Silane@Mn solution at 425 nm were decreased distinctly, indicating that both nanocomposites had a certain photosensitization effect, that is, they could produce ROS under laser irradiation. Compared with UCNPs-Ce6@Silane or UCNPs-Ce6@Silane@Mn solution alone, the DPBF absorbance in the UCNPs-Ce6@Silane solution showed no obvious change after the addition of H2O2. However, in the UCNPs-Ce6@Silane@Mn solution after addition of H2O2, the decrease in DPBF absorbance at 425 nm was accelerated, demonstrating that DPBF had been consumed in a short time, this is because MnO2 could catalyze the decomposition of H2O2 to produce oxygen, which greatly improves the yield of singlet oxygen.
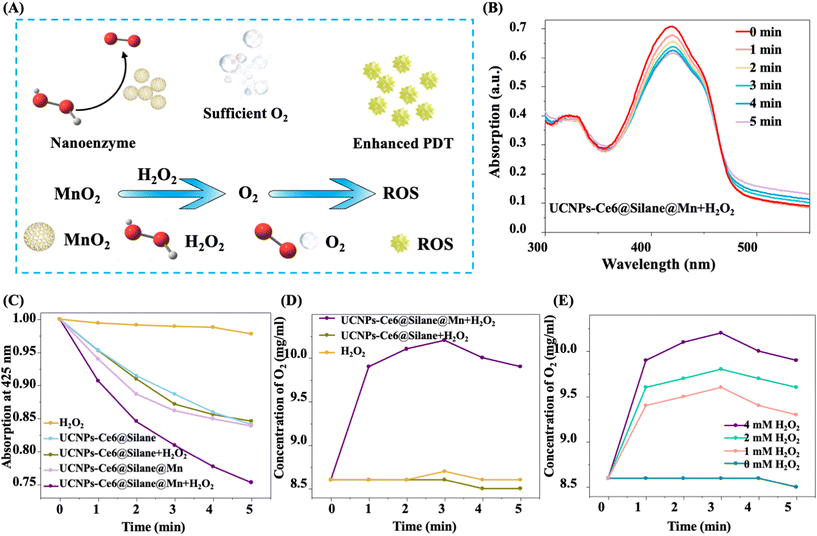 |
| Fig. 4 (A) In vitro mechanism of O2 and ROS generation. (B) Absorption spectra of DPBF in UCNPs-Ce6@Silane@Mn composite nanomaterial solutions mixed with H2O2 under red light with an interval of 1 min (C) Absorbance changes at 425 nm of DPBF solutions in the presence of H2O2, UCNPs-Ce6@Silane, UCNPs-Ce6@Silane@Mn, UCNPs-Ce6@Silane + H2O2, and UCNPs-Ce6@Silane@Mn + H2O2. (D) O2 generation from different NC solutions (UCNPs-Ce6@Silane, UCNPs-Ce6@Silane@Mn). (E) O2 generation with different concentrations of H2O2 (1–4 mM) after addition of UCNPs-Ce6@Silane@Mn. | |
In addition, in the mixed solution of UCNPs-Ce6@Silane@Mn and H2O2, the rapid and continuous generation of dissolved O2 could be detected over time (Fig. 4D). This result supports the significant catalytic activity of UCNPs-Ce6@Silane@Mn for providing O2 in the presence of H2O2, which speeds up the production of ROS and improves the efficacy of PDT, while UCNPs-Ce6@Silane obviously does not have this catalytic activity. At present, studies have shown that H2O2 plays an important role in mediating cell growth and apoptosis.45 In the process of cell proliferation and apoptosis, its concentration ranges from 10−8 M to 10−4 M, spanning four orders of magnitude. A large amount of H2O2 will be produced in the process of normal cell carcinogenesis. It has been concluded that H2O2 is a very important intracellular indicator signal molecule in the occurrence and development of cancer.46 As shown in Fig. 4E, we further measured in situ oxygen generation with different concentrations of H2O2, showing that when the concentration of UCNPs-Ce6@Silane@Mn remains unchanged, a large amount of O2 is still produced in the presence of very low concentration H2O2. Therefore, the construction of the nano platform provides the possibility to effectively ameliorate the tumor hypoxic microenvironment and improve the efficacy of PDT.
3.4. PDT effect of different NPs at cellular level
The PDT effect was first examined at the cellular level.47 In this work, we further investigated the PDT functions in MCF-7 cell and B16 cell line, respectively. MCF-7 cells and B16 cells were incubated with different NPs (100 μg mL−1) for 4 h, and then 980 nm irradiation at 4 W cm−2 (3 min) was conducted. First, we detected the production of singlet oxygen in cell systems with different NPs by the ROS-sensitive fluorescence probe DCFH-DA. DCFH-DA probes have no fluorescence, and they can freely pass through the cell membrane and be hydrolyzed into DCFH by esterase in the cell. However, DCFH cannot penetrate the cell membrane, so the probe can be easily loaded into the cell. Reactive oxygen species in cells can oxidize non-fluorescent DCFH to produce fluorescent DCF.48 Therefore, the level of reactive oxygen species in cells can be known by detecting the fluorescence of DCF. As shown in Fig. 5A and D, under the same experimental condition, different groups defined as only laser, Ce6, UCNPs-Ce6@Silane, and UCNPs-Ce6@Silane@Mn produced different levels of singlet oxygen. In both cell lines (MCF-7 and B16), both groups with UCNPs-Ce6@Silane and UCNPs-Ce6@Silane@Mn could induce ROS production based on the DCFH-DA probe. Obviously, the fluorescence intensity of the group with UCNPs-Ce6@Silane@Mn is much higher than that of the group with UCNPs-Ce6@Silane. However, the group of laser only and the Ce6 group show no fluorescence, indicating that the production of singlet oxygen could not be induced without any photosensitizer or irradiation light. After statistical analysis of the relative fluorescence intensity of each group, this result can be more intuitively proved (Fig. 5A and D).
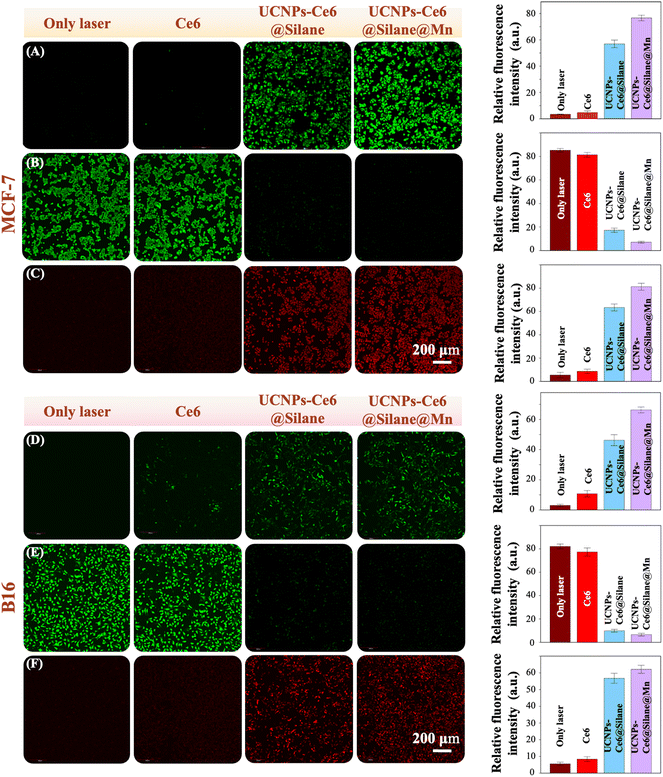 |
| Fig. 5 PDT experiments at the cell level with different NPs. Confocal microscopic images of MCF-7 and B16 cells incubated with different NPs (100 μg mL−1, 1 mL) for 4 h, after 5 min irradiation (980 nm, 4 W cm−2). (A) and (D) Stain by DCFH-DA detection for singlet oxygen production and statistical analysis of relative fluorescence intensity of each group. (B) and (E) MCF-7 and B16 cells stained by Calcein-AM for living cells and statistical analysis of relative fluorescence intensity of each group. (C) and (F) MCF-7 and B16 cells stained by PI for dead cells and statistical analysis of relative fluorescence intensity of each group. | |
The efficacy of PDT mainly depends on the production of singlet oxygen. The cellular treatment effect was detected by Calcein-AM/PI stain experiment. Calcein-AM can easily penetrate the living cell membrane and be hydrolyzed into Calcein by esterase after entering the cytoplasm, emitting strong green fluorescence, while PI passes through the disordered area of the dead cell membrane to the cell nucleus and displays intense red fluorescence due to the high cell permeability of dead cells.49 As can be seen from Fig. 5B and E, the cells in the laser only group and Ce6 group are basically alive and show intense green fluorescence, indicating that 980 nm irradiation for 3 min (4 W cm−2) or Ce6 cannot cause damage to cells without UCNPs. It is obvious that group UCNPs-Ce6@Silane and UCNPs-Ce6@Silane@Mn show a similar therapeutic effect based on Calcein-AM/PI stain results that a large number of dead cells can be seen after the same irradiation time and emit intense red fluorescence (Fig. 5C and F). In addition, it can still be seen that the therapeutic effect of the group UCNPs-Ce6@Silane@Mn is better than that of the group UCNPs-Ce6@Silane. Based on this, we still analyzed the relative fluorescence intensity of each group, and the statistical results also confirmed this. Therefore, in this experiment, the therapeutic effect in cells corresponds to the level of singlet oxygen induced by the NPs. According to the above-mentioned experimental results, we have reason to believe that multifunctional UCNPs-Ce6@Silane@Mn composite nanomaterials may become potential candidates for clinical cancer treatment.
3.5. Tumor therapy in vitro and in vivo
UCNPs-Ce6@Silane@Mn NPs were further applied in B16 tumor-bearing mice. Before that, the cytotoxicity of UCNPs-Ce6@Silane and UCNPs-Ce6@Silane@Mn was determined on MCF-7 cells and B16 cells by a standard Cell Counting Kit-8 (CCK-8) colorimetric assay. Under the condition of ensuring the consistency of Ce6 concentration in the two NPs, after 24 h incubation with the concentration varying from 50 to 200 μg mL−1, both groups exhibited satisfactory biocompatibility. It is worth mentioning that 980 nm laser is the only irradiation light. Under the 980 nm laser exposure with a power density of 4 W cm−2, the cytotoxicity of both NPs showed concentration dependence for the two kinds of cell lines.
The results indicated that the MCF-7 cells were 80% viability and B16 cells were 85% viability after incubation with UCNPs-Ce6@Silane@Mn with a concentration of 200 μg mL−1 without laser exposure (Fig. 6A and B). After comparing the cytotoxicity with several different concentrations of NPs, 100 μg mL−1 was selected finally as the in vitro experimental concentration. At this concentration, the MCF-7 cells and B16 cells keep more than 90% viability after treatment with two kinds of NPs. However, when laser irradiation was involved, the MCF-7 cell viability declines significantly to 33% with UCNPs-Ce6@Silane@Mn (100 μg mL−1). Due to the lack of oxygen-self-generation properties in the composite UCNPs-Ce6@Silane, the cell viability remained at 60% (Fig. 6A). As shown in Fig. 6B, in the B16 cell line, the cell viability decreased to 39% under the irradiation as treated with UCNPs-Ce6@Silane@Mn, while the viability treated with UCNPs-Ce6@Silane under 980 nm irradiation was 64%. The independent sample t-test results are marked in Fig. 6A and B. Different cell survival rates further confirmed the difference in the therapeutic effects of the two groups. The data showed that the treatment effect of UCNPs-Ce6@Silane@Mn was more significant. After incubation with cells for the same time, conduction of laser irradiation for 5 minutes was performed. During treatment, original oxygen in the cells was transformed into ROS by photosensitizers in the two groups. When all oxygen is consumed, UCNPs-Ce6@Silane loses its effectiveness, while UCNPs-Ce6@Silane@Mn maintains the function of catalyzing the decomposition of H2O2 in the cells to generate oxygen, continuously providing in situ oxygen, so as to generate ROS to kill the tumor cells. Therefore, the therapeutic effect of UCNPs-Ce6@Silane@Mn is better than that of UCNPs-Ce6@Silane. In the PDT process, the amount of hydrogen peroxide is an important factor, and until the amount of hydrogen peroxide is reduced, the therapeutic effect of PDT will be reduced, because the catalytic ability of MnO2 is sufficient.
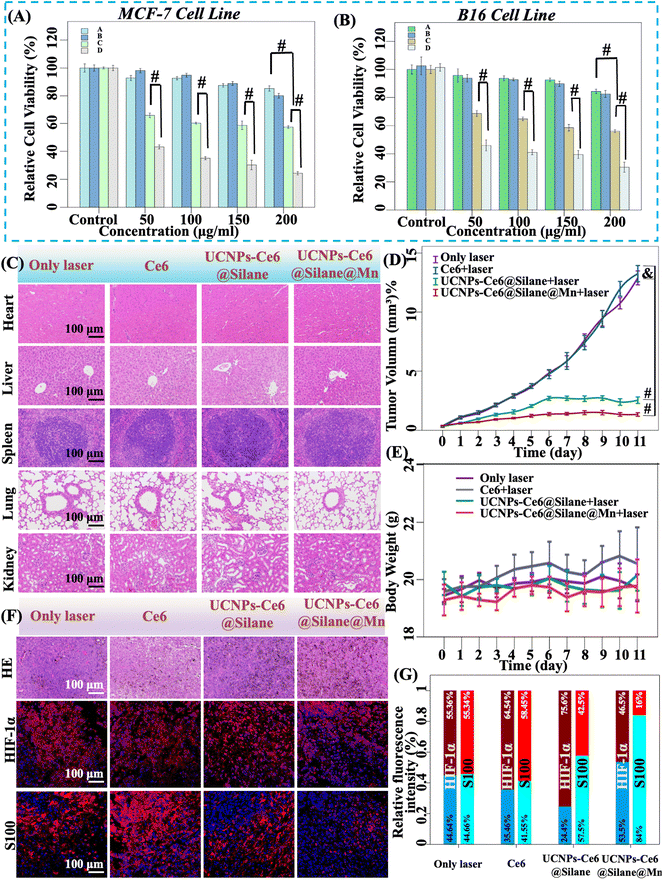 |
| Fig. 6 (A) and (B) Cell viability of the MCF-7 cell line and B16 cell line after 24 h incubation with different kinds of NPs and with (980 nm, 5 min, 4 W cm−2) or without irradiation. (A) UCNPs-Ce6@Silane, (B) UCNPs-Ce6@Silane@Mn, (C) UCNPs-Ce6@Silane with irradiation, (D) UCNPs-Ce6@Silane@Mn with irradiation. (C) H&E staining of major organ slices collected from mice 24 h posttreatment. (D) Tumor volume change curve of B16 tumor-bearing mice after treatment in different groups as indicated (980 nm, 5 min, 4 W cm−2). (E) Average body weights of mice collected within 11 days after treatment in different groups (two tailed t-test, & >0.05, # <0.001). (F) H&E staining and HIF-1α and S100 immunofluorescence staining images. (G) HIF-1α and S100 immunohistochemical experiment fluorescence statistical chart. | |
After in vivo treatment, we took out the tumor mass and major tissues and organs of mice in each group, including heart, liver, spleen, lung, and kidney, and stained them with hematoxylin and eosin (H&E staining) (Fig. 6C). Compared with the control group, no tissue damage was observed in the major organs of each group. Hence, it can be concluded that tumor treatment in vivo will not have adverse effects on the main organs and tissues of mice.
Considering the practical application of tumor treatment, we conducted animal-level experiments. The B16 tumor-bearing C57 mice were randomly divided into four groups with ten mice in each group: (1) laser only (control); (2) Ce6 and 980 nm laser; (3) UCNPs-Ce6@Silane and 980 nm laser; and (4) UCNPs-Ce6@Silane@Mn and 980 nm laser. Nanoparticles in different groups were irradiated with 980 nm laser (4 W cm−2 for 5 min) after injection for 2 hours (100 μL, ∼5 mg mL−1). The tumor volume and body weights of mice were measured every day after treatment. Through daily observation, we found that the tumor volume growth trend of different groups of mice was consistent with the estimated results (photographs of animals used for experiments are not allowed in animal laboratories). The tumor volume in the legs of mice in the control group (laser only) and the group with Ce6 injection increased rapidly. With the extension of time, we observed that the surface of tumor tissue in the legs of one mouse in the group with Ce6 injection showed a necrotic trend, and the necrotic tissue expanded with the continuous growth of tumor volume until the tumor tissue collapsed and the mice died on the 10th day after radiation. The tumor volume of the UCNPs-Ce6@Silane and 980 nm laser group increased slowly, and the tumor volume of UCNPs-Ce6@Silane@Mn and 980 nm laser group basically did not increase. Until the 9th day after treatment, the tumor volume of UCNPs-Ce6@Silane@Mn and 980 nm laser group decreased compared with the previous days, and the leg tumors of one mouse could not even be observed, leaving only a small black spot on the 11th day after treatment. The recorded data were plotted in the diagram, as shown in Fig. 6D, the volume growth curves of the control group and the group with Ce6 injection basically coincided, and there was no inhibitory effect, while the obvious tumor inhibition can be observed from the curves of the UCNPs-Ce6@Silane and 980 nm laser group and UCNPs-Ce6@Silane@Mn and 980 nm laser group. Compared with the tumor growth inhibition (TGI) rate of 78.13% in the UCNPs-Ce6@Silane and 980 nm laser group, the inhibition effect of tumor volume growth in the UCNPs-Ce6@Silane@Mn and 980 nm laser group is more significant, and the TGI is as high as 87.17%. No abnormal changes in body weight were observed in all groups (Fig. 6E). Therefore, it can be concluded that UCNPs-Ce6@Silane@Mn has the function of relieving the tumor hypoxic microenvironment by catalyzing the decomposition of H2O2 in the tumor microenvironment to produce in situ O2, which greatly improves the efficacy of PDT.
In Fig. 6F, H&E staining of the tumor showed that different degrees of damage can be seen in UCNPs-Ce6@Silane and 980 nm laser group and UCNPs-Ce6@Silane@Mn and 980 nm laser group in comparison with the control group, in which no necrosis or other damage were observed in the tumor structure with laser irradiation and Ce6 injection. In the group treated by UCNPs-Ce6@Silane, some areas of tissue necrosis were found, while large necrotic areas were observed in the UCNPs-Ce6@Silane@Mn treated group. Combined with the cellular level and in vitro experiment, which is in accordance with the expected results. The H&E staining suggests that the therapeutic effect of UCNPs-Ce6@Silane@Mn is superior to that of UCNPs-Ce6@Silane, and it had the potential possibility of clinical application. In addition, we also took tumor tissue blocks from different groups of mice for immunohistochemical experiments. We selected two antibodies, HIF-1α and S100. Under the microscope, the positive signals were reddish brown and red respectively. The results of immunohistochemical experiments and fluorescence statistical analysis are shown in Fig. 6F and G. The results indicated that in the control group (laser only) and Ce6 injection group, the positive expression rates of HIF-1α were 55.36% and 64.54%, respectively. In the UCNPs-Ce6@Silane group, the positive expression rate of HIF-1α was as high as 75.6%, and in the UCNPs-Ce6@Silane@Mn group, the positive expression rate of HIF-1α was only 46.5%.
Hypoxia is a common feature of malignant tumors. HIF-1α is a transcription factor that exists in mammals and humans under hypoxic conditions. At present, many studies have confirmed that HIF-1α is highly expressed in many malignant tumor tissues and plays an important role in tumor growth and invasion.50,51 As a central regulator of cell balance and hypoxia-induced gene expression, HIF-1α could regulate the transcription of a series of target genes (such as VEGF and GLUT1), and play an important role in tumor proliferation, metastasis, genesis and development. It is not only overexpressed in tumor cells and their metastatic cells, but also could induce the expression of abnormal genes in tumor tissues, and is closely related to tumor cell growth and tumor neovascularization.52 For example, in the hypoxic microenvironment of tumors, HIF-1α could induce increased expression of erythropoietin receptor, promote angiogenesis via up-regulation of VEGF expression, and simultaneously up-regulate the expression of glucose metabolism and glycolysis-related enzymes. These changes make cells adapt to hypoxia and enhance the adaptability of tumor cells synchronously. In addition, HIF-1α, as a result of a combination of intracellular hypoxia and genetic variation, could be a biomarker for highly aggressive cancers. In some malignant diseases, if HIF-1α is positive and highly expressed, the patients will still face high treatment inefficiency and mortality even if the pathological grade of the tumor is low. Therefore, reducing the expression of HIF-1α is of great significance for the treatment of malignant tumors.53 At the same time, photodynamic therapy is a process of oxygen consumption, which makes the oxygen in tumor tissue decrease sharply, thus promoting the expression of HIF-1α. Therefore, in the UCNPs-Ce6@Silane group, the positive expression rate of HIF-1α was the highest. However, UCNPs-Ce6@Silane@Mn has the function of self-oxygenation, so it will not cause an increase in oxygen consumption in tumor tissues during treatment. Therefore, the positive expression rate of HIF-1α was the lowest, indicating that the degree of hypoxia is also low, which proves the effectiveness of the drug.
S100 has been clearly recognized as a diagnostic marker for melanoma.54 The S100 protein family is the largest subfamily of Ca2+-binding proteins. Many studies have pointed out that S100 protein is closely related to tumor. First of all, most S100 genes are clustered on chromosome 1q21, which is prone to chromosome recombination and promote tumor occurrence. Second, the expression of S100 protein is increased in many malignant tumors, suggesting that its abnormal expression may be related to tumor development. Finally, some S100 proteins can interact with tumor-related proteins and regulate tumor factors such as NF-κB, P53 and beta-linked proteins to accelerate tumor development. It plays an important role in tumor genesis and metastasis, tumor microenvironment regulation, and tumor prognosis, and it has potential as a biomarker and prognostic factor. Since the 1980s, S100B expression in primary tumors has been used as a diagnostic marker for malignant melanoma in human and veterinary medicine, and signaling mediated by extracellular S100B may also contribute to melanoma progression. The interaction of stromal cell-derived S100A4 with RAGE on tumor cells also activated the expression of NF-κB dependent genes. Tumor cells subsequently release pro-inflammatory cytokines and paracrine factors that respectively stimulate endothelial cells and monocytes to promote angiogenesis and tumor immune response. At present, studies have also shown that S100A4 expression is associated with hepatocyte growth factor receptor (HGFR; also known as MET) or osteoporosis hormone in combination, which becomes a predictor of metastatic disease and poor survival. Intracellular and extracellular S100A4 and S100A7 as well as extracellular S100A8 and S100A9 could mediate breast tumor progression and metastasis. In cells that do not express estrogen receptor-α (ERα), intracellular S100A7 could improve cell survival and invasion by up-regulation of NF-κB and epidermal growth factor receptor (EGFR) signaling. Tumor cell-derived extracellular S100A7 binds to a receptor for advanced glycation end products (RAGE) on macrophages to mediate their recruitment into the tumor microenvironment. In addition, extracellular S100A7 binds to RAGE on endothelial cells to promote angiogenesis. The expression of S100A4 in tumor cells enhances cell migration and invasion via interactions with cytoskeletal effector factors such as myosin IIA and Rhotekin (RTKN).55 From Fig. 6F, it could be observed that the positive expression rate of S100 in the control group (laser only) and the Ce6 injection group (i.e., the red area) was significantly higher than that in the UCNPs-Ce6@Silane group and UCNPs-Ce6@Silane@Mn group, and the fluorescence statistical analysis data (Fig. 6G) showed that the positive rates of S100 were 55.34%, 58.45%, 42.5%, and 16%, respectively. Therefore, it can be concluded that the therapeutic effect of UCNPs-Ce6@Silane@Mn was significantly better than that of UCNPs-Ce6@Silane, and after the treatment of UCNPs-Ce6@Silane@Mn, the malignant degree of melanoma was significantly reduced. It is consistent with the expected experimental results. Therefore, we believe that UCNPs-Ce6@Silane@Mn does have the potential for clinical applications.
4. Conclusions
In summary, we have designed and prepared a smart nanoplatform integrating upconversion-based PDT and self-oxygenation functions with a single red emission band. The NaErF4:0.5%Tm@NaYF4:20%Yb UCNPs, Ce6 and MnO2 nanozyme could successfully be incorporated by an amphiphilic silane modification strategy, which could not only enhance the water solubility, but also form a hydrophobic space that can be used to load Ce6 molecules. The NaErF4:0.5%Tm@NaYF4:20%Yb core/shell UCNPs could convert NIR into 630 nm red light and excite the Ce6 molecules to produce a mass of ROS instantaneously. This property endowed a deeper penetration depth to PDT therapy for malignant tumors. Simultaneously, the nano-enzymatic activity of the MnO2 nanolayer plays an important role in catalyzing endogenous H2O2 to produce O2, greatly enhancing PDT performance and remodeling the tumor microenvironment. In order to verify the feasibility of the materials, we carried out experiments at the cellular level and animal level respectively. Remarkable therapeutic effects have been achieved in both levels due to the NIR light excitation as well as the self-supplement oxygenation design. The PDT-enhanced synergic anti-tumor capacity of UCNPs-Ce6@Silane@Mn NPs has been systematically confirmed both in vitro and in vivo. Importantly, the UCNPs-Ce6@Silane@Mn NPs with excellent anti-tumor properties also possessed great biocompatibility and effectively suppressive effects on the regulation of tumor microenvironment by the key factors of HIF-1α and S100 protein in vivo. In conclusion, as an effective strategy, UCNPs-Ce6@Silane@Mn NPs have provided beneficial strategies for in situ malignant tumor vanishment and microenvironment regulation, which shows great application prospects in the field of tumor therapy.
Author contributions
The manuscript was written through contributions of all authors. All authors have given approval to the final version of the manuscript.
Conflicts of interest
There are no conflicts to declare.
Acknowledgements
This work was supported by the National Natural Science Foundation of China (52250077, 52272080, 82201102), General Program of Natural Science Foundation of Jilin Province (20210401059YY, 20220402005GH, YDZJ202201ZYTS017, 20190201199JC, 20220204116YY), Jilin Health Science and Technology Capability Improvement Project (2021JC037), Science and Technology Project of Jilin Province Financial Department (jcsz2021893-2i), Science and Technology Project of Jilin Province Education Department (JJKH20221098KJ).
References
- N. Harbeck, F. Penault-Llorca, J. Cortes, M. Gnant, N. Houssami, P. Poortmans, K. Ruddy, J. Tsang and F. Cardoso, Breast cancer, Nat. Rev. Dis. Primers, 2019, 5, 66 CrossRef.
- K. L. Britt, J. Cuzick and K. A. Phillips, Key steps for effective breast cancer prevention, Nat. Rev. Cancer, 2020, 20, 417–436 CrossRef CAS PubMed.
- X. Li, J. F. Lovell, J. Yoon and X. Chen, Clinical development and potential of photothermal and photodynamic therapies for cancer, Nat. Rev. Clin. Oncol., 2020, 17, 657–674 CrossRef PubMed.
- H. Zhu, Y. Fang, Q. Miao, X. Qi, D. Ding, P. Chen and K. Pu, Regulating near-infrared photodynamic properties of semiconducting polymer nanotheranostics for optimized cancer therapy, ACS Nano, 2017, 11, 8998–9009 CrossRef CAS PubMed.
- R. Li, Z. Jia and M. A. Trush, Defining ROS in biology and medicine, React. Oxygen Species, 2016, 1, 9–21 Search PubMed.
- Q. Chen, X. Shao, M. Hao, H. Fang, R. Guan, Z. Tian, M. Li, C. Wang, L. Ji, H. Chao, J. Guan and J. Diao, Quantitative analysis of interactive behavior of mitochondria and lysosomes using stuctured illumination microscopy, Biomaterials, 2020, 250, 120059 CrossRef CAS.
- K. M. Holmström and T. Finkel, Cellular mechanisms and physiological consequences of redox-dependent signalling, Nat. Rev. Mol. Cell Biol., 2014, 15, 411–421 CrossRef.
- X. Qin, M. Zhang, X. Hu, Q. Du, Z. Zhao, Y. Jiang and Y. Luan, Nanoengineering of a newly designed chlorin e6 derivative for amplified photodynamic therapy via regulating lactate metabolism, Nanoscale, 2021, 13, 11953–11962 RSC.
- F. Li, C. Chen, X. Yang, X. He, Z. Zhao, J. Li, Y. Yu, X. Yang and J. Wang, Acetal-linked hyperbranched polyphosphoester nanocarriers loaded with chlorin e6 for ph-activatable photodynamic therapy, ACS Appl. Mater. Interfaces, 2018, 10, 21198–21205 CrossRef CAS.
- W. Liu, X. Ma, Y. Jin, J. Zhang, Y. Li, Y. Tang, Y. Song and S. Wang, Chlorin e6-biotin conjugates for tumor-targeting photodynamic therapy, Molecules, 2021, 26, 7342 CrossRef CAS PubMed.
- Y. Zhang, Z. He, Y. Li, Q. Xia, Z. Li, X. Hou and N. Feng, Tumor cell membrane-derived nano-Trojan horses encapsulating phototherapy and chemotherapy are accepted by homologous tumor cells, Mater. Sci. Eng., C, 2021, 120, 111670 CrossRef CAS PubMed.
- X. K. Sun, B. Dong, H. W. Xu, S. H. Xu, X. R. Zhang, Y. X. Lin, L. Xu, X. Bai, S. Zhang and H. W. Song, Amphiphilic silane modified multifunctional nanoparticles for magnetically targeted photodynamic therapy, ACS Appl. Mater. Interfaces, 2017, 9, 11451–11460 CrossRef CAS.
- Y. Sun, X. Sun, X. Li, W. Li and B. Dong, A versatile nanocomposite based on nanoceria for antibacterial enhancement and protection from aPDT-aggravated inflammation via modulation of macrophage polarization, Biomaterials, 2021, 268, 120614 CrossRef CAS.
- F. Li, Y. Du, J. Liu, H. Sun, J. Wang, R. Li, D. Kim, T. Hyeon and D. Ling, Responsive assembly of upconversion nanoparticles for ph-activated and near-infrared-triggered photodynamic therapy of deep tumors, Adv. Mater., 2018, 30, 1802808 CrossRef.
- T. Zhang, D. Ying, M. Qi, X. Li and Y. Zhou, Anti-Biofilm property of bioactive upconversion nanocomposites containing chlorin e6 against periodontal pathogens, Molecules, 2019, 24, 2692 CrossRef CAS.
- Y.-C. Chan, M.-H. Chan, C.-W. Chen, R.-S. Liu, M. Hsiao and D. P. Tsai, Near-infrared-activated fluorescence resonance energy transfer-based nanocomposite to sense mmp2-overexpressing oral cancer cells, ACS Omega, 2018, 3, 1627–1634 CrossRef CAS PubMed.
- L. Yang, S. Zhu, Z. He, X. Li, J. Chen, S. Bi and J. Zhu, Aqueous-phase synthesis of upconversion metal-organic frameworks for ATP-responsive in situ imaging and targeted combinational cancer therapy, Chin. Chem. Lett., 2022, 33, 314–319 CrossRef CAS.
- X. Guo, W. Wu, Y. Li, J. Zhang, L. Wang and H. Ågren, Recent research progress for upconversion assisted dye-sensitized solar cells, Chin. Chem. Lett., 2021, 32, 1834–1846 CrossRef CAS.
- M. Qi, X. Li, X. Sun, C. Li, F. R. Tay, M. Weir, B. Dong, Y. Zhou, L. Wang and H. Xu, Novel nanotechnology and near-infrared photodynamic therapy to kill periodontitis-related biofilm pathogens and protect the periodontium, Dent. Mater., 2019, 35, 1665–1681 CrossRef CAS.
- M. Wang, J. Zhao, L. Zhang, F. Wei, Y. Lian, Y. Wu, Z. Gong, S. Zhang, J. Zhou and C. Ke, Role of tumor microenvironment in tumorigenesis, J. Cancer, 2017, 8, 761–773 CrossRef CAS PubMed.
- S. S. Lucky, K. C. Soo and Y. Zhang, Nanoparticles in photodynamic therapy, Chem. Rev., 2015, 115, 1990–2042 CrossRef CAS.
- X. Jing, F. Yang, C. Shao, K. Wei, M. Xie, H. Shen and Y. Shu, Role of hypoxia in cancer therapy by regulating the tumor microenvironment, Mol. Cancer, 2019, 18, 157 CrossRef PubMed.
- X. Li, N. Kwon, T. Guo, Z. Liu and J. Yoon, Innovative strategies for hypoxic-tumor photodynamic therapy, Angew. Chem., Int. Ed., 2018, 57, 11522–11531 CrossRef CAS.
- J. Majidpoor and K. Mortezaee, Angiogenesis as a hallmark of solid tumors - clinical perspectives, Cell. Oncol., 2021, 44, 715–737 CrossRef CAS.
- N. Yang, W. Y. Xiao, X. J. Song, W. J. Wang and X. C. Dong, Recent Advances in Tumor Microenvironment hydrogen peroxide-responsive materials for cancer photodynamic therapy, Nano-Micro Lett., 2020, 12, 212–238 Search PubMed.
- Q. Chen, L. Feng, J. Liu, W. Zhu, Z. Dong, Y. Wu and Z. Liu, Intelligent albumin–MnO2 nanoparticles as pH-/H2O2-responsive dissociable nanocarriers to modulate tumor hypoxia for effective combination therapy, Adv. Mater., 2016, 28, 7129–7136 CrossRef CAS PubMed.
- J. Liu, Q. Chen, W. Zhu, X. Yi, Y. Yang, Z. Dong and Z. Liu, Nanoscale-coordination-polymer-shelled manganese dioxide composite nanoparticles: a multistage redox/pH/H2O2-responsive cancer theranostic nanoplatform, Adv. Funct. Mater., 2017, 27, 1605926 CrossRef.
- Z. Yin, H. Li, W. Xu, S. Cui, D. Zhou, X. Chen, Y. Zhu, G. Qin and H. Song, Local field modulation induced three–order upconversion enhancement: combining surface plasmon effect and photonic crystal effect, Adv. Mater., 2016, 28, 2518–2525 CrossRef CAS PubMed.
- X. Chen, Y. S. Zhu, D. L. Zhou, W. Xu, J. Y. Zhu, G. C. Pan, Z. Yin, H. Wang, S. B. Cui and H. W. Song, Size-dependent downconversion near-infrared emission of NaYF4:Yb3+,Er3+ nanoparticles, J. Mater. Chem. C, 2017, 5, 2451–2458 RSC.
- R. Joshi, R. S. Perala, S. B. Shelar, A. Ballal, B. P. Singh and R. S. Ningthoujam, Super Bright red upconversion in NaErF4:0.5%Tm@NaYF4:20%Yb nanoparticles for anti-counterfeit and bioimaging applications, ACS Appl. Mater. Interfaces, 2021, 13, 3481–3490 CrossRef CAS PubMed.
- X. L. Sun, J. Sun, Y. Sun, C. Y. Li, J. Fang, T. S. Zhang, Y. Wan, L. Xu, Y. M. Zhou, L. Wang and B. Dong, Oxygen self-sufficient nanoplatform for enhanced and selective antibacterial photodynamic therapy against anaerobe-induced periodontal disease, Adv. Funct. Mater., 2021, 31, 2101040 CrossRef CAS.
- Jt. Ping, Hs. Peng, J. Qin, F. You, Y. Wang, G. Chen and M. Song, A fluorescent nanoprobe for real-time monitoring of intracellular singlet oxygen during photodynamic therapy, Microchim. Acta, 2018, 185, 269 CrossRef PubMed.
- M. Amiri, A. Pardakhti, M. Ahmadi-Zeidabadi, A. Akbari and M. Salavati-Niasari, Magnetic nickel ferrite nanoparticles: Green synthesis by Urtica and therapeutic effect of frequency magnetic field on creating cytotoxic response in neural cell lines, Colloids Surf., B, 2018, 172, 244–253 CrossRef CAS PubMed.
- J. Meng, J. M. Xing, Y. Z. Wang, J. Lu, Y. L. Zhao, X. Y. Gao, P. C. Wang, L. Jia and X. J. Liang, Epigenetic modulation of human breast cancer by metallofullerenol nanoparticles: in vivo treatment and in vitro analysis, Nanoscale, 2011, 3, 4713–4719 RSC.
- W. Zeng, X. Wu, T. Chen, S. Sun, Z. Shi, J. Liu, X. Ji, X. Zeng, J. Guan, L. Mei and M. Wu, Renal-clearable ultrasmall polypyrrole nanoparticles with size-regulated property for second near-infrared light-mediated photothermal therapy, Adv. Funct. Mater., 2021, 31, 2008362 CrossRef CAS.
- V. Bobek, K. Kolostova, D. Pinterova, G. Kacprzak, J. Adamiak, J. Kolodziej, M. Boubelik, M. Kubecova and R. M. Hoffman, A clinically relevant, syngeneic model of spontaneous, highly metastatic B16 mouse melanoma, Anticancer Res., 2010, 30, 4799–4803 Search PubMed.
- B. Chen, B. Dong, J. Wang, S. Zhang, L. Xu, W. Yu and H. Song, Amphiphilic silane modified NaYF4:Yb,Er loaded with Eu(TTA)3(TPPO)2 nanoparticles and their multi-functions: dual mode temperature sensing and cell imaging, Nanoscale, 2013, 5, 8541–8549 RSC.
- M. D. Liu, D. K. Guo, R. Y. Zeng, J. J. Ye, S. B. Wang, C. X. Li, Y. X. Sun, S. X. Cheng and X. Z. Zhang, Yolk-shell structured nanoflowers induced intracellular oxidative/thermal stress damage for cancer treatment, Adv. Funct. Mater., 2020, 30, 2006098 CrossRef CAS.
- J. Zhang, M. Xu, Y. Mu, J. Li and H. Han, Reasonably retard O2 consumption through a photoactivity conversion nanocomposite for oxygenated photodynamic therapy, Biomaterials, 2019, 218, 119312 CrossRef CAS PubMed.
- J. Q. Huang, Y. Huang, Z. L. Xue and S. J. Zeng, Tumor microenvironment responsive hollow mesoporous Co9S8@MnO2-ICG/DOX intelligent nanoplatform for synergistically enhanced tumor multimodal therapy, Biomaterials, 2020, 262, 120346 CrossRef CAS PubMed.
- R. Kumar, M. Nyk, T. Y. Ohulchanskyy, C. A. Flask and P. N. Prasad, Combined optical and MR bioimaging using rare earth ion doped NaYF4 nanocrystals, Adv. Funct. Mater., 2009, 19, 853–859 CrossRef CAS.
- Q. S. Chen, X. J. Xie, B. L. Huang, L. L. Liang, S. Y. Han, Z. G. Yi, Y. Wang, Y. Li, D. Y. Fan, L. Huang and X. G. Liu, Confining Excitation Energy in Er3+-Sensitized Upconversion Nanocrystals through Tm3+-Mediated Transient Energy Trapping, Angew. Chem., Int. Ed., 2017, 56, 7605–7609 CrossRef CAS.
- J. E. Choi, D. Kim and H. S. Jang, Intense upconversion red emission from Gd-doped NaErF4:Tm-based core/shell/shell nanocrystals under 980 and 800 nm near infrared light excitations, Chem. Commun., 2019, 55, 2261–2264 RSC.
- X. S. Cui, Y. Cheng, H. Lin, Q. P. Wu, J. Xu and Y. S. Wang, Boosting single-band red upconversion luminescence in colloidal NaErF4 nanocrystals: Effects of doping and inert shell, J. Rare Earths, 2019, 37, 573–579 CrossRef CAS.
- S. G. Rhee, H2O2, a necessary evil for cell signaling, Science, 2006, 312, 1882–1883 CrossRef.
- R. Chen, L. Zhang, J. Gao, W. Wu, Y. Hu and X. Jiang, Chemiluminescent nanomicelles for imaging hydrogen peroxide and self-therapy in photodynamic therapy, J. Biomed. Biotechnol., 2011, 2011, 679492–679492 Search PubMed.
- H. W. Xu, B. Dong, S. H. Xu, S. Xu, X. K. Sun, J. Sun, Y. D. Yang, L. Xu, X. Bai, S. Zhang, Z. Yin and H. W. Song, High purity microfluidic sorting and in situ inactivation of circulating tumor cells based on multifunctional magnetic composites, Biomaterials, 2017, 138, 69–79 CrossRef CAS.
- E. Eruslanov and S. Kusmartsev, Identification of ROS using oxidized DCFDA and flow-cytometry, Methods Mol. Biol., 2010, 594, 57–72 CrossRef CAS PubMed.
- K. Nakajima, H. Takakura, Y. Shimizu and M. Ogawa, Changes in plasma membrane damage inducing cell death after treatment with near-infrared photoimmunotherapy, Cancer Sci., 2018, 109, 2889–2896 CrossRef CAS PubMed.
- E. Madan, B. Dikshit, S. H. Gowda, C. Srivastava, C. Sarkar, P. Chattopadhyay, S. Sinha and K. Chosdol, FAT1 is a novel upstream regulator of HIF1α and invasion of high grade glioma, Int. J. Cancer, 2016, 139, 2570–2582 CrossRef CAS.
- C. Gstalder, I. Ader and O. Cuvillier, FTY720 (Fingolimod) inhibits HIF1 and HIF2 signaling, promotes vascular remodeling, and chemosensitizes in renal cell carcinoma animal model, Mol. Cancer Ther., 2016, 15, 2465–2474 CrossRef CAS.
- G.-L. Semenza, Intratumoral hypoxia, radiation resistance, and HIF-1, Cancer Cell, 2004, 5, 405–406 CrossRef CAS.
- V. Infantino, A. Santarsiero, P. Convertini, S. Todisco and V. Iacobazzi, Cancer Cell Metabolism in Hypoxia: Role of HIF-1 as Key Regulator and Therapeutic Target, Int. J. Mol. Sci., 2021, 22, 5703 CrossRef CAS PubMed.
- N. G. Ordóñez, Value of melanocytic-associated immunohistochemical markers in diagnosing malignant melanoma: a review and update, Hum. Pathol., 2014, 45, 191–205 CrossRef.
- A. Bresnick, D. Weber and D. Zimmer, S100 proteins in cancer, Nat. Rev. Cancer, 2015, 15, 96–109 CrossRef CAS PubMed.
Footnote |
† These authors contribute equally to this work. |
|
This journal is © the Partner Organisations 2023 |
Click here to see how this site uses Cookies. View our privacy policy here.