DOI:
10.1039/D3QO00274H
(Research Article)
Org. Chem. Front., 2023,
10, 2405-2415
Stereoselective access to 2-deoxy-2-trifluoromethyl sugar mimetics by trifluoromethyl-directed 1,2-trans glycosylation†
Received
22nd February 2023
, Accepted 25th March 2023
First published on 14th April 2023
Abstract
Fluorinated carbohydrate mimetics are valuable molecular fragments in contemporary Glycoscience. Available synthetic protocols are mainly restricted to the preparation of ‘standard’ monofluorinated derivatives, whereas their more complex C(sp3)–CF3 congeners remain virtually underdeveloped. A protocol for accessing a series of previously uncharted 2-deoxy-2-trifluoromethyl-D-hexopyranosides from D-glycals is disclosed. The stereoselectivity of the glycosylation step, which is mainly governed by a combination of electronic and more dominant steric factors, revealed a pronounced substrate control rendering 1,2-trans glycosides as a result of the configuration of the CF3 moiety at C-2. The synthetic utility of this approach was demonstrated in the preparation of 2-CF3-glycoconjugates of natural origin, including disaccharides, cholesterol analogs, amino acids, and sphingosine/phytosphingosine derivatives.
Introduction
There is growing interest in the preparation of carbohydrate mimetics that overcome some of the limitations, such as short lifetimes and low absorption properties (reduced hydrophobicity), typically associated with drugs based on natural carbohydrates.1 Among them, F-glycomimetics,2 resulting from the bioisosteric replacement of H and OH by F,3 and particularly those featuring polyfluoroalkyl groups (RF),4 such as CF3
5 (CH3 isoster) and C2F5,6 have recently attracted considerable interest (Fig. 1A).7 However, although the preparation of CF3-containing active principal ingredients (APIs) has been long appreciated in MedChem due to the beneficial properties such polyfluorinated groups impart (e.g., enhanced lipophilicity and metabolic/oxidative stability),8 CF3-glycosides remain largely underrepresented9,10 in Glycoscience compared to their monofluorinated congeners. Interestingly, the latter are widely used as inhibitors of glycosidases,11 lectin ligands,12 building blocks to construct synthetic (glyco)peptides/proteins13–15 for carbohydrate vaccine design,16 as well as radiotracers for imaging applications (e.g., [18F]FDG).17
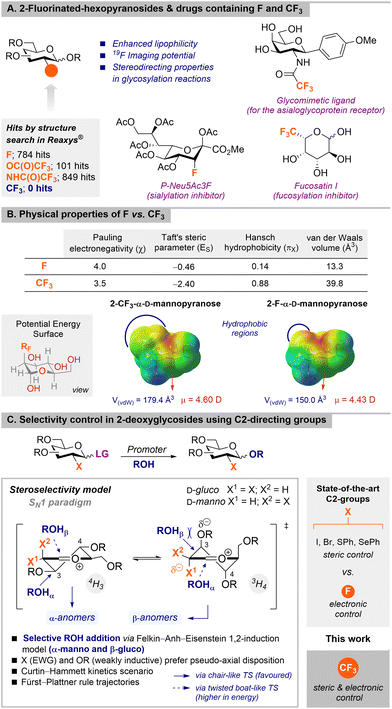 |
| Fig. 1 (A) 2-Fluorinated-hexopyranosides and selected drugs containing F and CF3 groups. Hits obtained with the Reaxys database. (B) Physical properties of F vs. CF3 groups (top). Calculated Electrostatic potential surfaces (EPS), van der Waals volume (blue), and dipole moment (magnitude and vector, red) (bottom) (see the ESI† for details). (C) Selectivity control in 2-deoxyglycosides bearing temporary (X = I, Br, SPh, SePh) and permanent (X = F, CF3) C2-directing groups. | |
We hypothesise the unique properties3 of the CF3 group, with similar polarity but larger steric constraints compared to those of the F atom; will likely influence metabolism, distribution, uptake, and therapeutic potential in CF3-monosaccharides (Fig. 1B, top). This trend is also supported by calculations of electrostatic potential surface (EPS), van der Waals volume, and dipole moment (magnitude and vector) for 2-CFmanno,gluco3vs. 2-Fmanno,gluco hexopyranoses (Fig. 1B, bottom and Figs. S1–S4, ESI†).18,19 Moreover, CF3 moieties may confer conformational constraints and enzymatic stability as well as privileged imaging potential (increased sensitivity in 19F-MRI and possibility to access [18F]-derivatives for PET imaging).17,20 Readily available trifluoroacetamides (NHC(O)CF3) and trifluoroacetoxy (OC(O)CF3) groups are the closest-art and the most frequent motifs among C(sp2)(O)CF3-containing glycosides.9,21 Unfortunately, other CF3-sugars are only limited to α-C(sp3)–CF3 alcohols resulting from 1,2-nucleophilic addition of TMSCF3 to carbonyls22 and, more recently, C(sp2)–CF3-glycals produced by photoredox23/electrochemical24 radical additions or via cross-couplings of CuCF3 with 2-iodoglycals.5 Besides its remarkable biophysical properties, F has also proven an efficient steering group for the stereoselective preparation of 2-deoxy-2-fluoroglycosides.25 In this case, the high 1,2-anti selectivity results from the preferred conformation of the oxocarbenium ion in the transition state reminiscent to half-chair geometries that are favoured by the high electronegativity of F. The energy of the transition state is minimized when the nucleophile trajectory is aligned with the
orbital, consistent with the Felkin–Anh–Eisenstein model for 1,2-stereoinduction.25 This contrasts with the stereoselectivity observed in similar examples using larger temporary C-2-directing groups such as halogens26 and chalcogens27 in which the 1,2-trans stereoselectivity is mainly governed by steric (Pauli) factors (Fig. 1C).28 Removal of these groups affords the corresponding 2-deoxyglycosides.29
In this context, we were intrigued on the behaviour of the CF3 group when placed at the C-2 position of a glycosyl donor, and whether the presence of such an electron-withdrawing and bulky unit may impart stabilizing/destabilizing interactions at the nearby oxocarbenium ion (e.g., nF → 2pC interactions),30 affecting the stereochemical outcome of the glycosylation reaction. Thus, here we report the development of a synthetic route (including a detailed study of the glycosylation reaction) to access new C(sp3)–CF3-(glyco)architectures. In particular, it would be interesting to comparatively determine if the electronic factors that govern the stereochemical outcome of the glycosylation of 2-deoxy-2-fluoro-glycosides are also operating in the case of 2-deoxy-2-CF3-derivatives (Fig. 1C, X = CF3). We anticipate the development of synthetic methods such as the one described in this study will establish the basis for successfully approaching further studies in complex fluorinated glycomimetics.
Results and discussion
Synthesis of glycosyl donors
In light of this, a strategy for the preparation of 2-CF3-glycosyl donors was envisaged from commercially available D-glucals as precursors (Scheme 1A). In our previous work, we reported the synthesis of 3a by iodination of D-glucal 1a to 2a (64% via dehydrative elimination)31 followed by selective trifluoromethylation using CF3H-derived CuCF3 (80%).5 This trifluoromethylation process required the use of a glovebox and manipulation of gaseous reagents. Herein, the overall synthetic sequence was improved by using first a more efficient iodination protocol (79% for 2a; 59% for 2b)32 and second, the use of a trifluoromethylation system (TMSCF3/KF/CuBr) that permits readily scalable synthesis and employs simpler reaction setup to access valuable 2-CF3-glycals (98% for 3a; 93% for 3b). Next, functionalization of the double bond was attempted, but the 2-CF3-alkene proved reluctant to undergo electrophilic additions, including halogenation, H+-mediated hydration, hydroboration, and epoxidation (Scheme S2, ESI†). Polyfluorinated alkenes and aromatic compounds are likely to reverse the natural polarity of such π-systems, making them prone to nucleophilic attacks.33 Nonetheless, structural examination of 3a,b advises a push–pull effect between the electron-donating oxygen of the enol ether and the electron-withdrawal of the CF3 group that in combination with its steric bulkiness, renders this double bond considerable unreactive (Scheme S2, ESI†). To our delight, hydroxymercuration of 3a using Hg(OTFA)2 followed by reductive demercuration with NaBH4 afforded a 1
:
1.6 mixture of gluco4a and manno5a pyranoses in 90% yield that after acetylation yielded chromatographically separable 4b (35%) and 5b (46%) (Scheme S3, ESI†). Collectively, the detection of only α-anomers indicates that similarly to 2-F, 2-CF3 reinforces the anomeric effect.34O-Benzyl protecting groups in 1-O-acetyl-pyranoses 4b and 5b were removed under hydrogenolysis conditions and subsequent acetylation afforded products 4c and 5c in good overall yields (up to 80%). Same reaction sequence starting from parent 1-OH-pyranoses 4a and 5a resulted in detrimental anomerization to afford inseparable α/β mixtures of gluco4c and manno5c pyranoses. Direct hydroxymercuration-reduction of 2-CF3-tri-O-acetyl-D-glucal 3b proved inefficient and 4b was obtained in a poor 34% yield along with unidentified rearrangement byproducts. Analysis of 4c by X-ray diffraction† indicated that typical 4C1 conformation is adopted in the solid state despite the presence of the sterically demanding CF3 group. 1H and 13C NMR data showed that the same geometry is also conserved in solution for both gluco and manno derivatives as determined by diagnostic 1JC1–H1 and 3JH,H coupling constants and key NOE contacts (Scheme 1B and Fig. S141, ESI†).
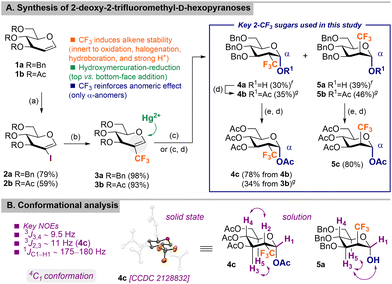 |
| Scheme 1 (A) Synthesis of 2-deoxy-2-trifluoromethyl-D-hexopyranoses of gluco and manno configurations and (B) conformational analysis. Reagents and conditions: (a) 1a,b (1 equiv.), NIS (1.2 equiv.), AgNO3 (0.2 equiv.), dry MeCN, reflux, 1 h (for 1a) up to 4 h (for 1b). (b) 2a,b (1 equiv.), CuCF3 (2 equiv.), 1 : 1 (v/v) DMF/DMI, 40 °C, 17 h and 50 °C, 16 h. (c) (i) 3a,b (1 equiv.), Hg(OTFA)2 (1.5 equiv.), 3 : 1 THF/H2O, 0 °C to rt, 36 h; (ii) NaBH4 (6.3 equiv.), H2O, 0 °C, 20 min. (d) Ac2O (3.6 equiv.), pyridine, rt, 15 h. (e) H2 (1 atm), 10% Pd/C, MeOH, rt, 16 h. fThis yield corresponds to a pure analytical sample obtained after a tedious fractional crystallization process. Prior column chromatography of the crude reaction afforded an inseparable mixture of 1 : 1.6 4a/5a in 90% yield. gYield over three steps from 3a (for 4b, 5b) and 3b (for 4c). Isolated yields given for all compounds unless otherwise indicated (see the ESI† for details). DMF = N,N-dimethylformamide, DMI = 1,3-dimethyl-2-imidazolidinone, TFA = trifluoroacetic acid, THF = tetrahydrofuran. ORTEP drawing of 4c with thermal ellipsoids drawn at the 50% probability level (H atoms omitted for clarity). | |
After optimizing the preparation of 2-CF3-pyranoses, the synthesis of suitable glycosyl donors was further examined (Scheme 2A and Scheme S4, ESI†). We first explored the preparation of trichloroacetimidate 5a-OTCA by reacting 5a with DBU and Cl3CN, but the poor yields obtained (48%) and the need of purification reduced its synthetic utility.
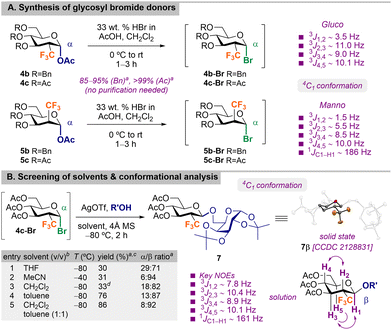 |
| Scheme 2 (A) Synthesis of glycosyl bromide donors and (B) screening of glycosylation solvents and conformational analysis. Reagents and conditions: aYield and selectivity determined by 19F NMR of the crude reaction mixture using 1,4-difluorobenzene as internal standard unless otherwise indicated. bReactions performed at 0.1 M concentration. cYield over two steps from 4c. dPoor solubility of AgOTf observed (see the ESI† for details). MS = molecular sieves, THF = tetrahydrofuran, NR = no reaction. ORTEP drawing of 7β with thermal ellipsoids drawn at the 50% probability level (H atoms omitted for clarity). | |
Reacting 5b with BF3·OEt2 and thiophenol in CH2Cl2 afforded thioglycoside donor 5b-SPh in a very low 23% isolated yield (Scheme S4, ESI†). Notably, reaction of pyranoses 4–5b,c with HBr in AcOH delivered glycosyl bromides (4–5b,c)-Br in almost quantitative yields (Scheme 2A). Glycosyl bromides proved to be stable, and they were directly used as glycosyl donors in subsequent glycosylation reactions without further purification.
Glycosylation reaction
Next, preliminary glycosylation reactions were attempted using anomeric acetates 4b, 5b, trichloroacetimidate 5a-OTCA, and thioglycoside 5b-SPh as donors (Scheme S5, ESI†). While the reaction of anomeric acetates showed no conversion using common promoters (0.1–4 equiv. TMSOTf or 3 equiv. BF3·OEt up to 72 h at room temperature) for both manno and gluco configurations (Scheme S5, entries 1–3, ESI†), trichloroacetimidate 5a-OTCA afforded expected product 6a in a good combined 69% yield and 38
:
62 α/β ratio (Scheme S5, entry 4, ESI†). In contrast, activation of 5b-SPh with the NIS/TfOH35 system only started when temperatures above −35 °C where reached, affording 6a (73%) in a 58
:
42 α/β ratio along with hydrolysis byproducts (∼27%) (Scheme S5, entry 5, ESI†). In summary, the preparation of thioglycoside and trichloroacetimidates resulted problematic, delivering low isolated yields of 5a-OTCA and 5b-SPh, required additional purification steps, and are not applicable to gluco configurations. Moreover, glycosylation of these donors offered substantial hydrolysis, poor 1,2-trans control of the CF3 group (5a-OTCA), required higher activation temperatures (5b-SPh) that compromise good stereoselectivities for general application of this protocol or showed no reactivity (4b and 5b). Next, we focused our attention towards the glycosylation of easily accessible glycosyl bromides (Scheme 2B),36 which showed smooth activation at very low temperatures. Thus, CFgluco34c-Br was selected as a model substrate and 1,2
:
3,4-di-O-isopropylidene-α-D-galactopyranose as the acceptor with 4 Å MS and silver triflate (AgOTf) as the promoter (Scheme 2B). Preliminary tests showed the importance of conducting the reaction at very low temperature (ca. −80 °C), in particular for gluco configuration, due to the propensity of 2-CF3-glucosyl bromides to undergo elimination processes to the parent 2-CF3-D-glucal (5–20%). Solvent screening showed that reactions in THF afforded low yields and the selectivity was poor (Scheme 2B, entry 1). When using acetonitrile at −40 °C, excellent β-selectivities were obtained, albeit with lower yield (Scheme 2B, entry 2). Using either CH2Cl2 or toluene, the selectivity was similar, affording 7β as the major isomer, yet in CH2Cl2, the yield was eroded, probably due to solubility issues with AgOTf (Scheme 2B, entries 3 vs. 4). In this case, no surface effects on selectivity were detected. Finally, best yield and selectivities were obtained in a 1
:
1 (v/v) CH2Cl2/toluene mixture (Scheme 2B, entry 5). Treatment of 7β under these optimized glycosylation conditions discarded anomerization of final 2-CF3-glycosides during the reaction. Similarly to their hexopyranose precursors and the resulting glycosyl donors (Schemes 1B and 2A), glycosylation products also maintain the same 4C1 conformation both in the solid state (X-ray for 7β)† and in solution (Scheme 2B and Fig. S5 and S142, ESI†). This observation suggests that a priori, 2-deoxy-2-CF3-glycoside analogs of natural products could be designed to maintain molecular recognition events (unless key specific interactions are operative at C-2) by biological entities, validating our starting hypothesis (Fδ− → CF3δ−).
Aiming to analyze the directing effect of the CF3 on the stereoselectivity of the glycosylation reaction, donors with different 2-CF3-configuration and electronically different protecting groups were examined (Table 1). To facilitate direct comparison of the stereodirecting value of CF3vs. F, isopropanol was chosen as nucleophile following seminal reports by Gilmour.25Gluco-CF3 glycosyl donors favoured formation of β-products, whereas α-glycosides were preferred in manno-CF3 donors (Table 1, entries 1–4). The best selectivities obtained resulted from the combination of OAc-protecting groups with CFmanno35c-Br (95
:
5 α/β) and OBn groups with CFgluco34b-Br (3
:
97 α/β). We first evaluated the role of the concentration (0.05 vs. 0.1 M)37 and stoichiometry (1
:
1, 1
:
2, and 1
:
15 donor/acceptor molar ratio),38 which are critical parameters for the glycosylation outcome (Table S1, entries 1–4, ESI†).39 Only little yield (except in the case of using 1 equiv. of acceptor where more byproducts are detected) and selectivity differences were found in reactions conducted at different reaction concentrations or with increased acceptor stoichiometry that suggests a more SN1-like dissociative mechanism. The use of promoters bearing non-nucleophilic counter ions such as AgBF4
40 and AgSbF6 (unable to provide OTf to the reaction medium) afforded similar selectivities that those with AgOTf, which strongly suggests that anomeric triflates are not likely responsible for the selectivity observed (Table S1, entries 1–4 vs. 5 and 6, ESI†). Our findings are in line with the results obtained in the glycosylation of 2-fluorosugars, yet in this case, trichloroacetimidates were used as glycosyl donors.25 Remarkably, the role of the leaving group in the stereoselectivity seems important (CFmanno35a-OTCA; 38
:
62 α/β ratio; Scheme S5, ESI†) vs. (CFmanno35b-SPh; 58
:
42 α/β ratio; Scheme S5, ESI†) vs. (CFmanno35b-Br, 79
:
21 α/β; Table 1, entry 4).39 Reactions using α-Br/SPh as leaving groups seem to be placed closer to the SN1 end of the glycosylation mechanistic continuum, proceeding via a common oxocarbenium ion/solvent-separated ion pair (SSIP) species. Small differences in selectivity may be attributed to slightly different activation temperatures (−80 °C for Br vs. −35 °C for SPh).41 Attempts to shed light on putative reactive intermediates were performed by 19F and 1H variable temperature (VT)-NMR experiments42 with bromide 4c-Br using our optimized reaction conditions but no intermediates were detected under preactivation conditions (preferred to detect transient intermediates). Subsequent addition of the nucleophile (iPrOH) delivered a complex mixture of signals from which only traces of final glycosylation product 10 could be observed. This result is in line with that using only 1 equiv. of acceptor in which increased amounts of byproducts are detected (Table S1, entry 3, ESI†). On the other hand, as expected,42,43 a more biased SN2-like scenario with a larger contribution of α-contact ion pair (CIP) species or even α-anomeric triflates from 5a-OTCA to tentatively rationalize the increased β-selectivity is invoked.44 Thus, for the sake of a more accurate comparison (F vs. CF3), 2-deoxy-2-fluoroglycosyl bromides 8–9a,b-Br14 were synthetized from 8–9a,b and submitted to the same reaction conditions of the present study. Stereoselectivities in 2-deoxy-2-fluoro derivatives 14–16 were similar to those of trifluoromethylated analogs 10–12 (Table 1, entries 1–3 vs. 5–7). However, inversion of the selectivity was observed in the OBn-manno-sugars 13 and 17 (Table 1, entry 4 vs. 8). Limited stereoselective control was also detected for OBn-manno derivatives in previous works,25 due to mismatched effects between protecting groups and the 2-fluoro substituent. Stereoselectivity in 2-deoxy-2-fluorosugars is rationalized by the Felkin-Anh-Eisenstein 1,2-induction model (Fig. 1C, X = F) in which stereoselective control is reinforced or diminished depending on the electronic nature of protecting groups (due to electrostatic and hyperconjugative interactions).45 The higher α-stereoselectivity observed in CFmanno3vs. Fmanno OBn-derivatives may arise from a higher contribution of the steric control, which is reminiscent to that observed with methyl groups in substitution reactions of acyclic α-methyl-β-alkoxy acetals via electrostatically stabilized oxocarbenium ion intermediates.46 Next, the effect of the acceptor nucleophilicity was examined with donor CFgluco34c-Br. No remarkable differences were observed when isopropanol and ethanol were used as acceptors and high 1,2-trans stereoselectivities were again obtained (Table 1, entry 1 vs. 9). On the other hand, lower or even inverted (1,2-cis) stereoselectivities were found when larger/weaker nucleophilic tert-butyl alcohol and trifluoroethanol were used as acceptors as they require donors with substantial oxocarbenium ion character and are engaged in dissociative SN1-like reactions (Table 1, entries 10 and 11).47 The increased steric hindrance of the acceptor may disfavour its approach by the top face due to steric (Pauli) repulsions with protecting groups (Fig. 2, middle). Results are compatible with a Curtin-Hammett scenario, where ΔG‡II increases energy more than ΔG‡I for bulky and/or weak nucleophiles (Fig. 2, bottom).
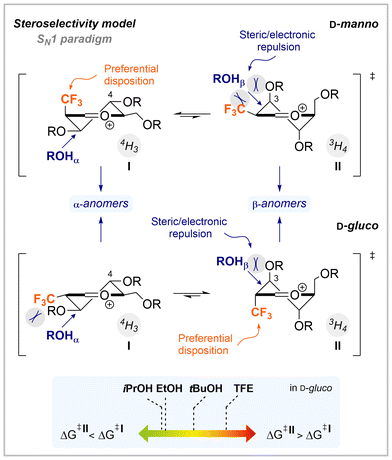 |
| Fig. 2 Stereoselectivity rationalization of the glycosylation step in D-manno (top) and D-gluco series (middle). Analysis of the glycosylation of D-gluco donors with small alcohols possessing different stereoelectronic properties as acceptors (bottom). | |
Table 1 Effect of pyranose configuration, C-2 substituent, protecting groups, and acceptor nucleophilicity in the glycosylation reactiona
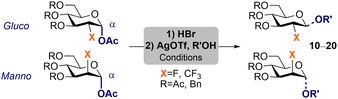
|
Entry |
2-X-pyranose |
R |
R′ |
Product yieldb (%) |
α/β ratiob |
General conditions: (1) 2-X-pyranose (1 equiv.), 33 wt% HBr in AcOH, 0 °C to rt, 1–3 h; (2) glycosyl bromide donor (1 equiv.), R'OH (2 equiv.), and AgOTf (2 equiv.) in 1 : 1 (v/v) CH2Cl2/toluene (0.05 M), −80 °C, 3 h unless otherwise indicated. Variable amounts of elimination side-reactions to the parent 2-CF3-D-glucal were detected by 19F NMR (9–32% in Glc and <10% in Man).
Determined by 19F NMR analysis of the crude reaction mixture using 1,4-difluorobenzene as internal standard (see the ESI† for details).
|
1 |
CFgluco3 |
4c
|
Ac |
iPr |
10, 61 |
33 : 67 |
2 |
CFmanno3 |
5c
|
Ac |
iPr |
11, 91 |
95 : 5 |
3 |
CFgluco3 |
4b
|
Bn |
iPr |
12, 72 |
3 : 97 |
4 |
CFmanno3 |
5b
|
Bn |
iPr |
13, 62 |
79 : 21 |
5 |
Fgluco |
8a
|
Ac |
iPr |
14, 95 |
24 : 76 |
6 |
Fmanno |
9a
|
Ac |
iPr |
15, 84 |
95 : 5 |
7 |
Fgluco |
8b
|
Bn |
iPr |
16, 78 |
4 : 96 |
8 |
Fmanno |
9b
|
Bn |
iPr |
17, 65 |
20 : 80 |
9 |
CFgluco3 |
4c
|
Ac |
Et |
18, 78 |
22 : 78 |
10 |
CFgluco3 |
4c
|
Ac |
tBu |
19, 58 |
42 : 58 |
11 |
CFgluco3 |
4c
|
Ac |
CH2CF3 |
20, 62 |
72 : 28 |
Glycosylation scope
Finally, reaction scope was further examined using a diversity of representative acceptors, including primary and secondary glycosides, amino acids, and lipid aglycones such as cholesterol and sphingosine/phytosphingosine derivatives (Scheme 3). Thus, sequential bromination of 4b, 5b,c and subsequent glycosylation with the primary acceptor 1,2
:
3,4-di-O-isopropylidene-α-D-galactopyranose afforded products 21–23 in good yields (up to 80%) with expected 1,2-trans stereoselectivities (up to 94
:
6 α/β in manno; 6
:
94 α/β in gluco). In most cases, the major anomer was obtained as a single product after chromatographic purification. When the minor isomer was produced to some extent, the epimers could be successfully separated and structurally characterized. Again, analysis of 8, 21–23 by 1H NMR showed 4C1 conformations dominate in all cases (Fig. S5, ESI†). It should be noted that 2-CF3-D-glucal byproduct was obtained in variable amounts (10–35%) upon competing elimination process using CFgluco3 donors that resulted in reduced overall yields for certain glycosylations. As expected, CFmanno3 afforded preferably α-glycosides 24 (67%) and 25 (78%) with excellent stereoselectivities (up to 97
:
3 α/β) and slightly better yields when reacted with these secondary alcohols, since elimination side-reactions are significantly less operative from manno-configured donors (<10%). Reaction with biphenyl-2-methanol occurred smoothly with gluco donors to afford β-glycosides and selectivity was slightly benefited using OBn groups (8
:
92 α/β in 26vs. 18
:
82 α/β in 27). In this case, the lower yield obtained for peracetylated biphenyl methyl 27 is caused by the higher amount of elimination (29%) in comparison to the perbenzylated counterpart (11%).
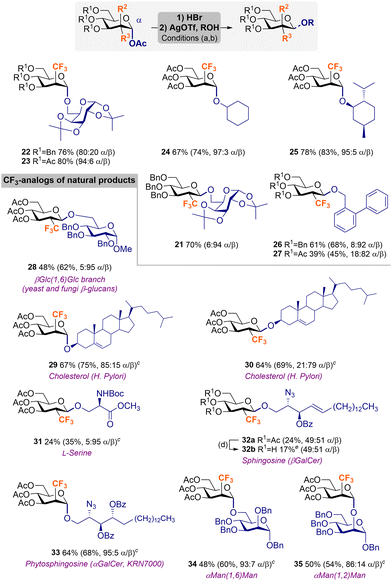 |
| Scheme 3 Glycosylation scope. Reagents and conditions: (a) 4–5b,c (1 equiv.), 33 wt% HBr in AcOH, 0 °C to rt, 4 h. (b) Glycosyl donor (1 equiv.), ROH (2 equiv.), and AgOTf (2 equiv.) in 1 : 1 (v/v) CH2Cl2/toluene (0.05 M), −80 °C, 2 h. (c) To facilitate purification, the crude was subjected to acetylation; Ac2O (6 equiv.), 1 : 1 (v/v) pyridine/CH2Cl2, rt, 16 h and the residue purified by flash column chromatography. (d) To facilitate purification, the crude was subjected to Zemplén deacetylation; NaOMe, MeOH, rt, 6 h and the residue purified by flash column chromatography. eYield over three steps from 4c. Isolated yields given for all compounds unless otherwise indicated. In parenthesis yield and/or diastereomeric ratio calculated by 19F NMR of the crude reaction mixture using 1,4-difluorobenzene as internal standard (see the ESI† for details). Variable amounts of elimination side-reactions to the parent 2-CF3-D-glucal were detected by 19F NMR (10–35% in Glc and <10% in Man). Boc = tert-butoxycarbonyl. | |
The presented protocol was applied to the stereoselective synthesis of 2-CF3-analogs of some relevant natural products. Despite the higher amount of side-elimination reactions using peracetylated glycosyl donors (typically in CFgluco3), these were chosen as reactants in the glycosylation scope, mainly due to their higher flexibility for accessing final unprotected derivatives (via Zemplén deacetylation) in comparison to the perbenzylated analogs. Fair yield, although excellent selectivity, was observed in the synthesis of 28 (βGlc(1,6)Glc branch found in yeast and fungi β-glucans) from the mismatched donor 4c-Br (48%, 5
:
95 α/β). Reaction of cholesterol with both gluco and manno epimers afforded 29 and 30 (mimetics of natural cholesteryl glucosides from H. pylori) with good isolated yields (up to 67%) and stereoselectivities (85
:
15 α/β in 29; 21
:
79 α/β in 30) and only minute elimination (10–15%). When using protected L-serine as acceptor, low yield of the desired product 31 (24%) was obtained due to competing elimination process (25%). However, excellent β-selectivity (5
:
95 α/β) was achieved by virtue of the equatorial CF3 configuration. Unexpectedly, the stereoselective control was completely eroded using a protected azido-sphingosine analog48 that reacted with CFgluco34c-Br producing an equimolar anomeric mixture of the desired product 32a (24% by 19F NMR). Moreover, its problematic purification required the hydrolysis of acetyl groups to obtain 32b in pure form and a poor 17% isolated yield (over three steps from 4c). In stark contrast, when protected azido-phytosphingosine acceptor was reacted with CFmanno35c-Br, excellent α-selectivity was obtained (95
:
5 α/β) and 33 (KRN7000 precursor)49 was isolated in 64% yield. The same glycosyl donor smoothly reacted with primary and secondary sugar acceptors to afford important αMan(1,6)Man and αMan(1,2)Man epitopes 34 (48%) and 35 (50%), respectively with preferred α-configuration (up to 93
:
7 α/β).
Conclusion
In summary, we have disclosed the unprecedented synthesis and glycosylation of 2-deoxy-2-trifluoromethylsugars that are in turn prepared from readily available D-glycals.
The study of the stereoelectronic properties of the CF3 group highlighted the selectivity control of glycosylation affording 1,2-trans glycosides. Structural screening indicated that best stereoselectivities are reinforced by the effect of protecting groups (OBn/CFgluco3 as β-selective; OAc/CFmanno3 as α-selective). Glycosylation proved successful for a range of different alcohols including primary and secondary aliphatic-OH, sugars, and complex aglycones. Interestingly, conformational analyses of resulting CF3-glycosides showed no perturbations in the molecular geometry, which may stimulate the development of new CF3-sugar mimetics with interesting physicochemical properties (e.g., log
P enhancement and/or increased 19F NMR sensitivity) to complement the applications of traditional F-sugars. Indeed, reaction scope also proved successful for the preparation biologically relevant C(sp3)–CF3-glycosides including amino acids, cholesterol, and sphingosine/phytosphingosine analogs. Collectively, it has been demonstrated that the method is synthetically useful for accessing novel 2-deoxy-2-CF3-glycosides bearing aglycones of different structural nature in very high stereoselectivities even when a non-optimal combination of protecting groups and CF3 configuration is used. We expect this report will help to flourish the field of polyfluoroalkyl sugar chemistry by establishing a new direction of development in glycomimetic design. Further synthetic developments and mechanistic investigations on the role of CF3 in the stability of the glycosidic bond and the modulation of physicochemical and recognition/processing properties are subjects of ongoing studies in our laboratory.
Author contributions
J.M and I.B. performed all of the experiments. M.B. performed the computational calculations. O.B. supervised the project. O.B. and S.C. were responsible for funding acquisition. All authors contributed to the preparation of the manuscript.
Conflicts of interest
There are no conflicts to declare.
Acknowledgements
We thank the Spanish Government-MCIU/MCIN, the national agency of investigation-AEI/10.13039/501100011033, and the European Regional Development Fund-ERDF (projects; CTQ2017-90088-R and PID2020-120584RB-I00 to O. B., CTQ2017-89750-R to S. C. FPI Fellowship; PRE2018-085730 to I. B. and FPU Fellowship; FPU19/01969 to M. B.) and the Universitat Rovira i Virgili (Martí i Franquès Research Fellowship Programme; 2012BPURV-67 to J. M.) for financial support. We also thank the ICIQ X-ray diffraction unit for the crystallographic studies and Aria Valaei for preliminary experiments. O. B. was a Ramón y Cajal Fellow (RYC-2015-17705).
References
-
(a) R. Hevey, Strategies for the development of glycomimetic drug candidates, Pharmaceuticals, 2019, 12, 55 CrossRef CAS PubMed;
(b) R. C. Saliba and N. L. B. Pohl, Designing sugar mimetics: non-natural pyranosides as innovative chemical tools, Curr. Opin. Chem. Biol., 2016, 34, 127–134 CrossRef CAS PubMed.
-
(a) R. Hevey, The role of fluorine in glycomimetic drug design, Chem. – Eur. J., 2021, 27, 2240–2253 CrossRef CAS PubMed;
(b) B. Linclau, A. Ardá, N. C. Reichardt, M. Sollogoub, L. Unione, S. P. Vincent and J. Jiménez-Barbero, Fluorinated carbohydrates as chemical probes for molecular recognition studies. Current status and perspective, Chem. Soc. Rev., 2020, 49, 3863–3888 RSC.
-
(a) R. Hevey, Bioisosteres of carbohydrate functional groups in glycomimetic design, Biomimetics, 2019, 4, 53 CrossRef CAS PubMed;
(b) N. A. Meanwell, Fluorine and fluorinated motifs in the design and application of bioisosteres for drug design, J. Med. Chem., 2018, 61, 5822–5880 CrossRef CAS PubMed;
(c) G. A. Patani and E. J. LaVoie, Bioisosterism: a rational approach in drug design, Chem. Rev., 1996, 96, 3147–3176 CrossRef CAS PubMed.
- M. L. Uhrig, E. W. Mora Flores and A. Postigo, Approaches to the synthesis of perfluoroalkyl-modified carbohydrates and derivatives: thiosugars, iminosugars, and tetrahydro(thio)pyrans, Chem. – Eur. J., 2021, 27, 7813–7825 CrossRef CAS PubMed.
- J. Mestre, A. Lishchynskyi, S. Castillón and O. Boutureira, Trifluoromethylation of electron-rich alkenyl iodides with fluoroform-derived “ligandless” CuCF3, J. Org. Chem., 2018, 83, 8150–8160 CrossRef CAS PubMed.
- J. Mestre, S. Castillón and O. Boutureira, “Ligandless” pentafluoroethylation of unactivated (hetero)aryl and alkenyl halides enabled by the controlled self-condensation of TMSCF3-derived CuCF3, J. Org. Chem., 2019, 84, 15087–15097 CrossRef CAS PubMed.
- M. A. Miller and E. M. Sletten, Perfluorocarbons in chemical biology, ChemBioChem, 2020, 21, 3451–3462 CrossRef CAS PubMed.
-
(a)
D. Cahard and J.-A. Ma, Emerging fluorinated motifs: synthesis, properties, and applications, Wiley-VCH, Weinheim, 2020 Search PubMed;
(b) B. M. Johnson, Y.-Z. Shu, X. Zhuo and N. A. Meanwell, Metabolic and pharmaceutical aspects of fluorinated compounds, J. Med. Chem., 2020, 63, 6315–6386 CrossRef CAS PubMed;
(c) E. P. Gillis, K. J. Eastman, M. D. Hill, D. J. Donnelly and N. A. Meanwell, Applications of fluorine in medicinal chemistry, J. Med. Chem., 2015, 58, 8315–8359 CrossRef CAS PubMed.
-
(a) C. Song, X.-J. Zheng, H. Guo, Y. Cao, F. Zhang, Q. Li, X.-S. Ye and Y. Zhou, Fluorine-modified sialyl-Tn-CRM197 vaccine elicits a robust immune response, Glycoconjugate J., 2019, 36, 399–408 CrossRef CAS PubMed;
(b) C.-X. Huo, X.-J. Zheng, A. Xiao, C.-C. Liu, S. Sun, Z. Lv and X.-S. Ye, Synthetic and immunological studies of N-acyl modified S-linked STn derivatives as anticancer vaccine candidates, Org. Biomol. Chem., 2015, 13, 3677–3690 RSC;
(c) H.-Y. Lee, C.-Y. Chen, T.-I. Tsai, S.-T. Li, K.-H. Lin, Y.-Y. Cheng, C.-T. Ren, T.-J. R. Cheng, C.-Y. Wu and C.-H. Wong, Immunogenicity study of Globo H analogues with modification at the reducing or nonreducing end of the tumor antigen, J. Am. Chem. Soc., 2014, 136, 16844–16853 CrossRef CAS PubMed;
(d) F. Yang, X.-J. Zheng, C.-X. Huo, Y. Wang, Y. Zhang and X.-S. Ye, Enhancement of the immunogenicity of synthetic carbohydrate vaccines by chemical modifications of STn antigen, ACS Chem. Biol., 2011, 6, 252–259 CrossRef CAS PubMed;
(e) L. Dafik, M. d'Alarcao and K. Kumar, Modulation of cellular adhesion by glycoengineering, J. Med. Chem., 2010, 53, 4277–4284 CrossRef CAS PubMed.
- R. F. G. Fröhlich, E. Schrank and K. Zangger, 2,2,2-Trifluoroethyl 6-thio-β-D-glucopyranoside as a selective tag for cysteines in proteins, Carbohydr. Res., 2012, 361, 100–104 CrossRef PubMed.
-
(a) L. Wu, Z. Armstrong, S. P. Schröder, C. de Boer, M. Artola, J. M. Aerts, H. S. Overkleeft and G. J. Davies, An overview of activity-based probes for glycosidases, Curr. Opin. Chem. Biol., 2019, 53, 25–36 CrossRef CAS PubMed;
(b) B. P. Rempel and S. G. Withers, Covalent inhibitors of glycosidases and their applications in biochemistry and biology, Glycobiology, 2008, 18, 570–586 CrossRef CAS PubMed;
(c) S. J. Williams and S. G. Withers, Glycosyl fluorides in enzymatic reactions, Carbohydr. Res., 2000, 327, 27–46 CrossRef CAS PubMed.
-
(a) G. Fittolani, E. Shanina, M. Guberman, P. H. Seeberger, C. Rademacher and M. Delbianco, Automated glycan assembly of 19F-labeled glycan probes enables high-throughput NMR studies of protein–glycan interactions, Angew. Chem., Int. Ed., 2021, 60, 13302–13309 CrossRef CAS PubMed;
(b) J. D. Martínez, A. I. Manzano, E. Calviño, A. D. Diego, B. Rodriguez de Francisco, C. Romanò, S. Oscarson, O. Millet, H. J. Gabius, J. Jiménez-Barbero and F. J. Cañada, Fluorinated carbohydrates as lectin ligands: simultaneous screening of a monosaccharide library and chemical mapping by 19F NMR spectroscopy, J. Org. Chem., 2020, 85, 16072–16081 CrossRef PubMed;
(c) J. D. Martínez, A. S. Infantino, P. Valverde, T. Diercks, S. Delgado, N.-C. Reichardt, A. Ardá, F. J. Cañada, S. Oscarson and J. Jiménez-Barbero, The interaction of fluorinated glycomimetics with DC-SIGN: multiple binding modes disentangled by the combination of NMR methods and MD simulations, Pharmaceuticals, 2020, 13, 179 CrossRef PubMed;
(d) J. D. Martínez, P. Valverde, S. Delgado, C. Romanò, B. Linclau, N.-C. Reichardt, S. Oscarson, A. Ardá, J. Jiménez-Barbero and F. J. Cañada, Unraveling sugar binding modes to DC-SIGN by employing fluorinated carbohydrates, Molecules, 2019, 24, 2337 CrossRef PubMed;
(e) T. Diercks, A. S. Infantino, L. Unione, J. Jiménez-Barbero, S. Oscarson and H. J. Gabius, Fluorinated carbohydrates as lectin ligands: synthesis of OH/F-substituted N-glycan core trimannoside and epitope mapping by 2D STD-TOCSYreF NMR spectroscopy, Chem. – Eur. J., 2018, 24, 15761–15765 CrossRef CAS PubMed;
(f) J. P. Ribeiro, T. Diercks, J. Jiménez-Barbero, S. André, H. J. Gabius and F. J. Cañada, Fluorinated carbohydrates as lectin Ligands: 19F-based direct STD monitoring for detection of anomeric selectivity, Biomolecules, 2015, 5, 3177–3192 CrossRef CAS PubMed;
(g) E. Matei, S. André, A. Glinschert, A. S. Infantino, S. Oscarson, H.-J. Gabius and A. M. Gronenborn, Fluorinated carbohydrates as lectin ligands: dissecting glycan–cyanovirin interactions by using 19F NMR spectroscopy, Chem. – Eur. J., 2013, 19, 5364–5374 CrossRef CAS PubMed;
(h) S. André, F. J. Cañada, T. C. Shiao, L. Largartera, T. Diercks, M. Bergeron-Brlek, K. el Biari, A. Papadopoulos, J. P. Ribeiro, M. Touaibia, D. Solís, M. Menéndez, J. Jiménez-Barbero, R. Roy and H.-J. Gabius, Fluorinated carbohydrates as lectin ligands: biorelevant sensors with capacity to monitor anomer affinity in 19F-NMR-based inhibitor screening, Eur. J. Org. Chem., 2012, 4354–4364 CrossRef;
(i) T. Diercks, J. P. Ribeiro, F. J. Cañada, S. André, J. Jiménez-Barbero and H. J. Gabius, Fluorinated carbohydrates as lectin ligands:
versatile sensors in 19F–detected saturation transfer difference NMR spectroscopy, Chem. – Eur. J., 2009, 15, 5666–5668 CrossRef CAS PubMed.
-
(a) M. Johannes, M. Reindl, B. Gerlitzki, E. Schmitt and A. Hoffmann-Röder, Synthesis and biological evaluation of a novel MUC1 glycopeptide conjugate vaccine candidate comprising a 4′-deoxy-4′-fluoro-Thomsen–Friedenreich epitope, Beilstein J. Org. Chem., 2015, 11, 155–161 CrossRef PubMed;
(b) S. Lamandé-Langle, C. Collet, R. Hensienne, C. Vala, F. Chrétien, Y. Chapleur, A. Mohamadi, P. Lacolley and V. Regnault,
‘Click’ glycosylation of peptides through cysteine propargylation and CuAAC, Bioorg. Med. Chem., 2014, 22, 6672–6683 CrossRef PubMed;
(c) C. Vala, F. Chrétien, E. Balentova, S. Lamandé-Langle and Y. Chapleur, Neoglycopeptides through direct functionalization of cysteine, Tetrahedron Lett., 2011, 52, 17–20 CrossRef CAS;
(d) T. Oberbillig, C. Mersch, S. Wagner and A. Hoffmann-Röder, Antibody recognition of fluorinated MUC1 glycopeptide antigens, Chem. Commun., 2012, 48, 1487–1489 RSC;
(e) S. Wagner, C. Mersch and A. Hoffmann-Röder, Fluorinated glycosyl amino acids for mucin–like glycopeptide antigen analogues, Chem. – Eur. J., 2010, 16, 7319–7330 CrossRef CAS PubMed;
(f) A. Hoffmann-Röder, A. Kaiser, S. Wagner, N. Gaidzik, D. Kowalczyk, U. Westerlind, B. Gerlitzki, E. Schmitt and H. Kunz, Synthetic antitumor vaccines from tetanus toxoid conjugates of MUC1 glycopeptides with the Thomsen–Friedenreich antigen and a fluorine–substituted analogue, Angew. Chem., Int. Ed., 2010, 49, 8498–8503 CrossRef PubMed.
- M. Salvadó, B. Amgarten, S. Castillón, G. J. L. Bernardes and O. Boutureira, Synthesis of fluorosugar reagents for the construction of well-defined fluoroglycoproteins, Org. Lett., 2015, 17, 2836–2839 CrossRef PubMed.
-
(a) C.-C. Liu, C.-X. Huo, C. Zhai, X.-J. Zheng, D.-C. Xiong and X.-S. Ye, Synthesis and immunological evaluation of pentamannose-based HIV-1 vaccine candidates, Bioconjugate Chem., 2022, 33, 807–820 CrossRef CAS PubMed;
(b)
P. M. S. D. Cal, G. J. L. Bernardes and O. Boutureira, Fluoroglycoproteins by Copper-Free Strain-Promoted Azide–Alkyne Cycloaddition in Peptide and Protein Engineering, ed. O. Iranzo and A. C. Roque, Springer Protocols Handbooks, Humana, New York, 2020, p. 53 Search PubMed;
(c) J. Orwenyo, W. Huang and L. X. Wang, Chemoenzymatic synthesis and lectin recognition of a selectively fluorinated glycoprotein, Bioorg. Med. Chem., 2013, 21, 4768–4777 CrossRef CAS PubMed;
(d) O. Boutureira, G. J. L. Bernardes, M. Fernández-González, D. C. Anthony and B. G. Davis, Selenenylsulfide–Linked Homogeneous Glycopeptides and Glycoproteins: Synthesis of Human “Hepatic Se Metabolite A”, Angew. Chem., Int. Ed., 2012, 51, 1432–1436 CrossRef CAS PubMed;
(e) O. Boutureira, G. J. L. Bernardes, F. D'Hooge and B. G. Davis, Direct radiolabelling of proteins at cysteine using [18F]-fluorosugars, Chem. Commun., 2011, 47, 10010–10012 RSC;
(f) M. Fernández-González, O. Boutureira, G. J. L. Bernardes, J. M. Chalker, M. A. Young, J. C. Errey and B. G. Davis, Site-selective chemoenzymatic construction of synthetic glycoproteins using endoglycosidases, Chem. Sci., 2010, 1, 709–715 RSC;
(g) O. Boutureira, F. D'Hooge, M. Fernández-González, G. J. L. Bernardes, M. Sánchez-Navarro, J. R. Koeppe and B. G. Davis, Fluoroglycoproteins: ready chemical site-selective incorporation of fluorosugars into proteins, Chem. Commun., 2010, 46, 8142–8144 RSC.
-
(a)
O. Boutureira, Site-Selective Conjugation Chemistry for Synthetic Glycoconjugate Vaccine Development in Recent Trends in Carbohydrate Chemistry: Synthesis and Biomedical Applications of Glycans and Glycoconjugates, ed. A. P. Rauter, B. E. Christensen, L. Somsák, P. Kosma and R. Adamo, Elsevier, 2020, vol. 2, p. 335 Search PubMed;
(b) R. Adamo, A. Nilo, B. Castagner, O. Boutureira, F. Berti and G. J. L. Bernardes, Synthetically defined glycoprotein vaccines: current status and future directions, Chem. Sci., 2013, 4, 2995–3008 RSC.
-
(a) S. S. Shinde, S. Maschauer and O. Prante, Sweetening pharmaceutical radiochemistry by 18F-fluoroglycosylation: recent progress and future prospects, Pharmaceuticals, 2021, 14, 1175 CrossRef CAS PubMed;
(b) O. Jacobson, D. O. Kiesewetter and X. Chen, Fluorine-18 radiochemistry, labeling strategies and synthetic routes, Bioconjugate Chem., 2015, 26, 1–18 CrossRef CAS PubMed;
(c) I. Tirotta, V. Dichiarante, C. Pigliacelli, G. Cavallo, G. Terraneo, F. B. Bombelli, P. Metrangolo and G. Resnati,
19F Magnetic resonance imaging (MRI): from design of materials to clinical applications, Chem. Rev., 2015, 115, 1106–1129 CrossRef CAS PubMed;
(d) G. R. Morais, R. A. Falconer and I. Santos, Carbohydrate-based molecules for molecular imaging in nuclear medicine, Eur. J. Org. Chem., 2013, 1401–1414 CrossRef.
- Electrostatic potential surfaces were calculated after geometry optimization at the CPCM (water) B3LYP/6–311+G(d,p) level of theory using Gaussian 09.
M. J. Frisch, G. W. Trucks, H. B. Schlegel, G. E. Scuseria, M. A. Robb, J. R. Cheeseman, G. Scalmani, V. Barone, B. Mennucci, G. A. Petersson, H. Nakatsuji, M. Caricato, X. Li, H. P. Hratchian, A. F. Izmaylov, J. Bloino, G. Zheng, J. L. Sonnenberg, M. Hada, M. Ehara, K. Toyota, R. Fukuda, J. Hasegawa, M. Ishida, T. Nakajima, Y. Honda, O. Kitao, H. Nakai, T. Vreven, J. A. Montgomery Jr., J. E. Peralta, F. Ogliaro, M. Bearpark, J. J. Heyd, E. Brothers, K. N. Kudin, V. N. Staroverov, T. Keith, R. Kobayashi, J. Normand, K. Raghavachari, A. Rendell, J. C. Burant, S. S. Iyengar, J. Tomasi, M. Cossi, N. Rega, J. M. Millam, M. Klene, J. E. Knox, J. B. Cross, V. Bakken, C. Adamo, J. Jaramillo, R. Gomperts, R. E. Stratmann, O. Yazyev, A. J. Austin, R. Cammi, C. Pomelli, J. W. Ochterski, R. L. Martin, K. Morokuma, V. G. Zakrzewski, G. A. Voth, P. Salvador, J. J. Dannenberg, S. Dapprich, A. D. Daniels, O. Farkas, J. B. Foresman, J. V. Ortiz, J. Cioslowski and D. J. Fox, Gaussian 09, revision D.01, Gaussian, Inc., Wallingford, CT, 2013 Search PubMed.
- Y. Zhao, M. Abraham and A. Zissimos, Fast calculation of van der Waals volume as a sum of atomic and bond contributions and its application to drug compounds, J. Org. Chem., 2003, 68, 7368–7373 CrossRef CAS PubMed.
-
(a) S. Preshlock, M. Tredwell and V. Gouverneur, Labeling of arenes and heteroarenes for applications in positron emission tomography, Chem. Rev., 2016, 116, 719–766 CrossRef CAS PubMed;
(b) D. Van der Born, C. Sewing, J. K. D. M. Herscheid, A. D. Windhorst, R. V. A. Orru and D. J. A. Vugts, Universal procedure for the [18F]trifluoromethylation of aryl iodides and aryl boronic acids with highly improved specific activity, Angew. Chem.,
Int. Ed., 2014, 53, 11046–11050 CrossRef CAS PubMed.
- L. Unione, M. Alcalá, B. Echeverria, S. Serna, A. Ardá, A. Franconetti, F. J. Cañada, T. Diercks, N. Reichardt and J. Jiménez-Barbero, Fluoroacetamide moieties as NMR spectroscopy probes for the molecular recognition of GlcNAc-containing sugars: modulation of the CH–π stacking interactions by different fluorination patterns, Chem. – Eur. J., 2017, 23, 3957–3965 CrossRef CAS PubMed.
-
(a) A. R. Mandhapati, T. Kato, T. Matsushita, B. Ksebati, A. Vasella, E. C. Böttger and D. Crich, Fluorine-decoupled carbon spectroscopy for the determination of configuration at fully substituted, trifluoromethyl- and perfluoroalkyl-bearing carbons: comparison with 19F–1H heteronuclear overhauser effect spectroscopy, J. Org. Chem., 2015, 80, 1754–1763 CrossRef CAS PubMed;
(b) D. Crich and O. Vinogradova, Synthesis and glycosylation of a series of 6-mono-, di-, and trifluoro S-phenyl 2,3,4-tri-O-benzyl-thiorhamnopyranosides. Effect of the fluorine substituents on glycosylation stereoselectivity, J. Am. Chem. Soc., 2007, 129, 11756–11765 CrossRef CAS PubMed;
(c) R. Plantier-Royon and C. Portella, Difluoromethylene-containing, C-trifluoromethyl and C-perfluoroalkyl carbohydrates. Synthesis by carbohydrate transformation or building block methods, Carbohydr. Res., 2000, 327, 119–146 CrossRef CAS PubMed.
- B. Wang, D.-C. Xiong and X.-S. Ye, Direct C–H trifluoromethylation of glycals by photoredox catalysis, Org. Lett., 2015, 17, 5698–5701 CrossRef CAS PubMed.
- M. Liu, Z.-X. Luo, T. Li, D.-C. Xiong and X.-S. Ye, Electrochemical trifluoromethylation of glycals, J. Org. Chem., 2021, 86, 16187–16194 CrossRef CAS PubMed.
-
(a) C. S. Teschers and R. Gilmour, Fluorine-directed automated mannoside assembly, Angew. Chem., Int. Ed., 2023, 62, e202213304 CrossRef CAS PubMed;
(b) A. Axer, R. P. Jumde, S. Adam, A. Faust, M. Schäfers, M. Fobker, J. Koehnke, A. K. H. Hirsch and R. Gilmour, Enhancing glycan stability via site-selective fluorination: modulating substrate orientation by molecular design, Chem. Sci., 2021, 12, 1286–1294 RSC;
(c) T. J. Kieser, N. Santschi, L. Nowack, A. Axer, G. Kehr, S. Albrecht and R. Gilmour, Total chemical syntheses of the GM3 and F–GM3 ganglioside epitopes and comparative pre-clinical evaluation for non-Invasive imaging of oligodendrocyte differentiation, ACS Chem. Neurosci., 2020, 11, 2129–2136 CrossRef CAS PubMed;
(d) T. Hayashi, A. Axer, G. Kehr, K. Bergander and R. Gilmour, Halogen-directed chemical sialylation: pseudo-stereodivergent access to marine ganglioside epitopes, Chem. Sci., 2020, 11, 6527–6531 RSC;
(e) T. Hayashi, G. Kehr, K. Bergander and R. Gilmour, Stereospecific α-Sialylation by site–selective fluorination, Angew. Chem., Int. Ed., 2019, 58, 3814–3818 CrossRef CAS PubMed;
(f) T. J. Kieser, N. Santschi, L. Nowack, G. Kehr, T. Kuhlmann, S. Albrecht and R. Gilmour, Single site fluorination of the GM4 ganglioside epitope upregulates oligodendrocyte differentiation, ACS Chem. Neurosci., 2018, 9, 1159–1165 CrossRef CAS PubMed;
(g) A. Sadurni, G. Kehr, M. Ahlqvist, J. Wernevik, H. Peilot-Sjögren, C. Kankkonen, L. Knerr and R. Gilmour, Fluorine-directed glycosylation enables the stereocontrolled synthesis of selective SGLT2 inhibitors for type II diabetes, Chem. – Eur. J., 2018, 24, 2832–2836 CrossRef CAS PubMed;
(h) A. Sadurni and R. Gilmour, Stereocontrolled synthesis of 2-fluorinated C-glycosides, Eur. J. Org. Chem., 2018, 3684–3687 CrossRef CAS PubMed;
(i) N. Santschi and R. Gilmour, Comparative analysis of fluorine-directed glycosylation selectivity: interrogating C2 [OH → F] Substitution in D-glucose and D-galactose, Eur. J. Org. Chem., 2015, 6983–6987 CrossRef CAS;
(j) N. Aiguabella, M. C. Holland and R. Gilmour, Fluorine-directed 1,2-trans glycosylation of rare sugars, Org. Biomol. Chem., 2016, 14, 5534–5538 RSC;
(k) E. Durantie, C. Bucher and R. Gilmour, Fluorine–Directed β-Galactosylation: chemical glycosylation development by molecular editing, Chem. – Eur. J., 2012, 18, 8208–8215 CrossRef CAS PubMed;
(l) C. Bucher and R. Gilmour, Fluorine-directed glycosylation, Angew. Chem., Int. Ed., 2010, 49, 8724–8728 CrossRef CAS PubMed.
-
(a) J. Mestre, D. Collado, D. Benito-Alifonso, M. A. Rodríguez, M. I. Matheu, Y. Díaz, S. Castillón and O. Boutureira, Highly reactive 2-deoxy-2-iodo-d-allo and D-gulo pyranosyl sulfoxide donors ensure β-stereoselective glycosylations with steroidal aglycones, RSC Adv., 2018, 8, 30076–30079 RSC;
(b) J. Mestre, M. I. Matheu, Y. Díaz, S. Castillón and O. Boutureira, Chemical access to D-Sarmentose units enables the total synthesis of cardenolide monoglycoside N-1 from Nerium oleander, J. Org. Chem., 2017, 82, 3327–3333 CrossRef CAS PubMed;
(c) T. Kimura, D. Takahashi and K. Toshima, Glycosylations of glycals using N-Iodosuccinimide (NIS) and phosphorus compounds for syntheses of 2-iodo-and 2-deoxyglycosides, J. Org. Chem., 2015, 80, 9552–9562 CrossRef CAS PubMed;
(d) A. Kövér, O. Boutureira, M. I. Matheu, Y. Díaz and S. Castillón, Tuning the stereoelectronic properties of 1-sulfanylhex-1-enitols for the sequential stereoselective synthesis of 2-deoxy-2-iodo-β-D-allopyranosides, J. Org. Chem., 2014, 79, 3060–3068 CrossRef PubMed;
(e) M. A. Rodríguez, O. Boutureira, M. I. Matheu, Y. Díaz and S. Castillón, Stereoselective synthesis of 2-deoxyglycosides from sulfanyl alkenes by consecutive “one pot” cyclization and glycosylation reactions, Eur. J. Org. Chem., 2007, 2470–2476 CrossRef;
(f) M. A. Rodríguez, O. Boutureira, X. Arnés, Y. Díaz, M. I. Matheu and S. Castillón, Stereoselective synthesis of 2-deoxy-2-iodo-glycosides from furanoses. A new route to 2-deoxy-glycosides and 2-deoxy-oligosaccharides of ribo and xylo configuration, J. Org. Chem., 2005, 70, 10297–10310 CrossRef PubMed.
-
(a) O. Boutureira, M. A. Rodríguez, D. Benito, M. I. Matheu, Y. Díaz and S. Castillón, Stereoselective synthesis of 2-deoxy-2-phenylselenenyl glycosides from furanoses: implication of the phenylselenenyl group in the stereocontrolled preparation of 2-deoxy-ribo- and 2-deoxy-xylo-oligosaccharides, Eur. J. Org. Chem., 2007, 3564–3572 CrossRef CAS;
(b) K. C. Nicolaou, H. J. Mitchell, K. C. Fylaktakidou, H. Suzuki and R. M. Rodríguez, 1,2-Seleno migrations in carbohydrate chemistry: solution and solid-phase synthesis of 2-deoxy glycosides, orthoesters, and allyl orthoesters, Angew. Chem., Int. Ed., 2000, 39, 1089–1093 CrossRef CAS.
-
(a) K. M. Demkiw, W. A. Remmerswaal, T. Hansen, G. A. van der Marel, J. D. C. Codée and K. A. Woerpel, Halogen atom participation in guiding the stereochemical outcomes of acetal substitution reactions, Angew. Chem., Int. Ed., 2022, 61, e202209401 CrossRef CAS PubMed;
(b) A. Franconetti, A. Ardá, J. L. Asensio, Y. Blériot, S. Thibaudeau and J. Jiménez-Barbero, Glycosyl oxocarbenium ions: structure, conformation, reactivity, and interactions, Acc. Chem. Res., 2021, 54, 2552–2564 CrossRef CAS PubMed;
(c) L. Lebedel, A. Ardá, A. Martin, J. Désiré, A. Mingot, M. Aufiero, N. Aiguabella Font, R. Gilmour, J. Jiménez-Barbero, Y. Blériot and S. Thibaudeau, Structural and computational analysis of 2-halogeno-glycosyl cations in the presence of a superacid: an expansive platform, Angew. Chem., Int. Ed., 2019, 58, 13758–13762 CrossRef CAS PubMed;
(d) M. G. Beaver, S. B. Billings and K. A. Woerpel, Nucleophilic substitution reactions of 2-phenylthio-substituted carbohydrate acetals and related systems: episulfonium ions vs. oxocarbenium ions as reactive intermediates, Eur. J. Org. Chem., 2008, 771–781 CrossRef CAS;
(e) F. Bravo, A. Viso, E. Alcázar, P. Molas, C. Bo and S. Castillón, Computational insight into the reaction intermediates in the glycosylation reaction assisted by donor heteroatoms, J. Org. Chem., 2003, 68, 686–691 CrossRef CAS PubMed.
- C. S. Bennett and M. C. Galan, Methods for 2-deoxyglycoside synthesis, Chem. Rev., 2018, 118, 7931–7985 CrossRef CAS PubMed.
- A. J. Fernandes, A. Panossian, B. Michelet, A. Martin-Mingot, F. R. Leroux and S. Thibaudeau, CF3-substituted carbocations: underexploited intermediates with great potential in modern synthetic chemistry, Beilstein J. Org. Chem., 2021, 17, 343–378 CrossRef CAS PubMed.
-
(a) I. Cobo, M. I. Matheu, S. Castillón, O. Boutureira and B. G. Davis, Phosphine-free Suzuki–Miyaura cross-coupling in aqueous media enables access to 2-C-aryl-glycosides, Org. Lett., 2012, 14, 1728–1731 CrossRef CAS PubMed;
(b) M. A. Rodríguez, O. Boutureira, Y. Díaz, M. I. Matheu, S. Castillón and P. H. Seeberger, Synthesis of 2-iodoglycals, glycals, and 1,1′-disaccharides from 2-deoxy-2-iodopyranoses under dehydrative glycosylation conditions, J. Org. Chem., 2007, 72, 8998–9001 CrossRef PubMed.
- S. Dharuman and Y. D. Vankar, Halosuccinimide/AgNO3-efficient reagent systems for one-step synthesis of 2-haloglycals from glycals: application in the synthesis of 2C-branched sugars via Heck coupling reactions, Org. Lett., 2014, 16, 1172–1175 CrossRef CAS PubMed.
- S. Rohrbach, A. J. Smith, J. H. Pang, D. L. Poole, T. Tuttle, S. Chiba and J. A. Murphy, Concerted nucleophilic aromatic substitution reactions, Angew. Chem., Int. Ed., 2019, 58, 16368–16388 CrossRef CAS PubMed.
-
(a) I. V. Alabugin, L. Kuhn, N. V. Krivoshchapov, P. Mehaffy and M. G. Medvedev, Anomeric effect, hyperconjugation and electrostatics: Lessons from complexity in a classic stereoelectronic phenomenon, Chem. Soc. Rev., 2021, 50, 10212–10252 RSC;
(b) T. M. Barbosa, R. V. Viesser, R. J. Abraham, R. Rittner and C. F. Tormeno, Experimental and theoretical evaluation of trans-3-halo-2-hydroxy-tetrahydropyran conformational preferences. Beyond anomeric interaction, RSC Adv., 2015, 5, 35412–35420 RSC.
- G. Lian, X. Zhang and B. Yu, Thioglycosides in carbohydrate research, Carbohydr. Res., 2015, 403, 13–22 CrossRef CAS PubMed.
- Y. Singh, S. A. Geringer and A. V. Demchenko, Synthesis and glycosidation of anomeric halides: evolution from early studies to modern methods of the 21st century, Chem. Rev., 2022, 122, 11701–11758 CrossRef CAS PubMed.
-
(a) L. O. Kononov, N. N. Malysheva, A. V. Orlova, A. I. Zinin, T. V. Laptinskaya, E. G. Kononova and N. G. Kolotyrkina, Concentration dependence of glycosylation outcome: a clue to reproducibility and understanding the reasons behind, Eur. J. Org. Chem., 2012, 1926–1934 CrossRef CAS;
(b) C.-S. Chao, C.-W. Li, M.-C. Chen, S.-S. Chang and K.-K. T. Mong, Low-concentration 1,2-trans-β-selective glycosylation strategy and its applications in oligosaccharide synthesis, Chem. – Eur. J., 2009, 15, 10972–10982 CrossRef CAS PubMed.
-
(a) P. O. Adero, T. Furukawa, M. Huang, D. Mukherjee, P. Retailleau, L. Bohé and D. Crich, Cation clock reactions for the determination of relative reaction kinetics in glycosylation reactions: Applications to gluco- and mannopyranosyl sulfoxide and trichloroacetimidate type donors, J. Am. Chem. Soc., 2015, 137, 10336–10345 CrossRef CAS PubMed;
(b) M. Huang, P. Retailleau, L. Bohé and D. Crich, Cation clock permits distinction between the mechanisms of α- and β-O-and β-C-glycosylation in the mannopyranose series: evidence for the existence of a mannopyranosyl oxocarbenium ion, J. Am. Chem. Soc., 2012, 134, 14746–14749 CrossRef CAS PubMed.
-
(a) P. R. Andreana and D. Crich, Guidelines for O-glycoside formation from first principles, ACS Cent. Sci., 2021, 7, 1454–1462 CrossRef CAS PubMed;
(b) H. H. Trinderup, S. M. Andersen, M. Heuckendorff and H. H. Jensen, How do various reaction parameters influence anomeric selectivity in chemical glycosylation with thioglycosides and NIS/TfOH activation?, Eur. J. Org. Chem., 2021, 3251–3259 CrossRef CAS;
(c) S. Chatterjee, S. Moon, F. Hentschel, K. Gilmore and P. H. Seeberger, An empirical understanding of the glycosylation reaction, J. Am. Chem. Soc., 2018, 140, 11942–11953 CrossRef CAS PubMed.
- S. Kaeothip, P. Pornsuriyasak and A. V. Demchenko, Silver(I) tetrafluoroborate as a potent promoter for chemical glycosylation, Tetrahedron Lett., 2008, 49, 1542–1545 CrossRef CAS PubMed.
- O. T. Tuck, E. T. Sletten, J. Danglad-Flores and P. H. Seeberger, Towards a systematic understanding of the influence of temperature on glycosylation reactions, Angew. Chem., Int. Ed., 2022, 61, e202115433 CrossRef CAS PubMed.
- T. G. Frihed, M. Bols and C. M. Pedersen, Mechanisms of glycosylation reactions studied by low-temperature nuclear magnetic resonance, Chem. Rev., 2015, 115, 4963–5013 CrossRef CAS PubMed.
-
(a) M. M. Nielsen, T. Holmstrøm and C. M. Pedersen, Stereoselective O-Glycosylations by pyrylium salt organocatalysis, Angew. Chem., Int. Ed., 2022, 61, e202115394 CrossRef CAS PubMed;
(b) T. Li, T. Li, H. Zhuang, F. Wang, R. R. Schmidt and P. Peng,
O-Glycosyl trichloroacetimidates as glycosyl donors and platinum(IV) chloride as a dual catalyst permitting stereo- and regioselective glycosidations, ACS Catal., 2021, 11, 10279–10287 CrossRef CAS;
(c) M. M. Nielsen, P. Mała, E. Þ. Baldursson and C. M. Pedersen, Self-promoted and stereospecific formation of N-glycosides, Chem. Sci., 2019, 10, 5299–5307 RSC;
(d) K. Kowalska and C. M. Pedersen, Catalytic stereospecific O-glycosylation, Chem. Commun., 2017, 53, 2040–2043 RSC;
(e) P. Peng and R. R. Schmidt, An alternative reaction course in O-glycosidation with O-glycosyl trichloroacetimidates as glycosyl donors and Lewis acidic metal salts as catalyst: Acid–base catalysis with gold chloride-glycosyl acceptor adducts, J. Am. Chem. Soc., 2015, 137, 12653–12659 CrossRef CAS PubMed.
-
(a) P. Merino, I. Delso, S. Pereira, S. Orta, M. Pedrón and T. Tejero, Computational evidence of glycosyl cations, Org. Biomol. Chem., 2021, 19, 2350–2365 RSC;
(b) P. O. Adero, H. Amarasekara, P. Wen, L. Bohé and D. Crich, The experimental evidence in support of glycosylation mechanisms at the SN1–SN2 interface, Chem. Rev., 2018, 118, 8242–8284 CrossRef CAS PubMed;
(c) L. Bohé and D. Crich, A propos of glycosyl cations and the mechanism of chemical glycosylation; the current state of the art, Carbohydr. Res., 2015, 403, 48–59 CrossRef PubMed.
-
(a) K. M. Demkiw, C. T. Hu and K. A. Woerpel, Hyperconjugative interactions of the carbon–halogen bond that influence the geometry of cyclic α-haloacetals, J. Org. Chem., 2022, 87, 5315–5327 CrossRef CAS PubMed;
(b) D. M. Smith and K. A. Woerpel, Electrostatic interactions in cations and their importance in biology and chemistry, Org. Biomol. Chem., 2006, 4, 1195–1201 RSC.
- A. Ramdular and K. A. Woerpel, Diastereoselective substitution reactions of acyclic β-alkoxy acetals via electrostatically stabilized oxocarbenium ion intermediates, Org. Lett., 2022, 24, 3217–3222 CrossRef CAS PubMed.
- S. van der Vorm, T. Hansen, H. S. Overkleeft, G. A. van der Marel and J. D. C. Codée, The influence of acceptor nucleophilicity on the glycosylation reaction mechanism, Chem. Sci., 2017, 8, 1867–1875 RSC.
-
(a) J. A. Morales-Serna, O. Boutureira, Y. Díaz, M. I. Matheu and S. Castillón, Highly efficient and stereoselective synthesis of β-glycolipids, Org. Biomol. Chem., 2008, 6, 443–446 RSC;
(b) J. A. Morales-Serna, O. Boutureira, Y. Díaz, M. I. Matheu and S. Castillón, Recent advances in the glycosylation of sphingosines and ceramides, Carbohydr. Res., 2007, 342, 1595–1612 CrossRef CAS PubMed.
- O. Boutureira, J. A. Morales-Serna, Y. Díaz, M. I. Matheu and S. Castillón, Direct and efficient glycosylation protocol for synthesizing α-glycolipids: application to the synthesis of KRN7000, Eur. J. Org. Chem., 2008, 1851–1854 CrossRef CAS.
|
This journal is © the Partner Organisations 2023 |
Click here to see how this site uses Cookies. View our privacy policy here.