DOI:
10.1039/D2RA07466D
(Paper)
RSC Adv., 2023,
13, 4211-4221
Lead accumulation and biochemical responses in Rhus chinensis Mill to the addition of organic acids in lead contaminated soils†
Received
23rd November 2022
, Accepted 23rd January 2023
First published on 2nd February 2023
Abstract
Adding organic acid is an effective approach to assist phytoremediation. The effects of organic acids on phytoremediation efficiency are unknown in Rhus chinensis. This study aimed to evaluate the effect of citric acid (CA) and oxalic acid (OA) on the lead phytoremediation potential of R. chinensis with significantly inhibited growth in Pb-contaminated soil. The experimental pot culture study evaluated the long-term physiological response and metal accumulation patterns of R. chinensis grown in varying Pb-treated soil, and examined the effects of 0.5 and 1.0 mmol L−1 CA and OA on the growth, oxidative stress, antioxidant system, and Pb subcellular distribution of R. chinensis grown in pots with 1000 mg kg−1 Pb. Compared with the control, the biomass, leaf area, root morphological parameters, and chlorophyll concentration of R. chinensis decreased, whereas the carotenoid, malondialdehyde, H2O2, and O2˙− concentrations, and superoxide dismutase (SOD), peroxidase (POD), and catalase (CAT) activity increased under Pb stress. A copious amount of Pb was taken up and mainly stored in the cell walls of the roots. The application of CA and OA increased plant growth. The highest shoots and roots biomass increase recorded was 44.4 and 61.2% in 1.0 mmol L−1 OA and 0.5 mmol L−1 CA treatment, respectively. The presence of CA and OA increased SOD, POD, and CAT activities and decreased the H2O2, O2˙− and malondialdehyde content. A concentration of 0.5 mmol L−1 CA significantly increased the Pb concentration in the organs. The other organic acid treatments changed root Pb concentrations slightly while increasing shoot Pb concentrations. The translocation factor values from organic acid treatments were increased by 38.8–134.1%. Our results confirmed that organic acid could alleviate the toxicity of stunted R. chinensis and improve phytoremediation efficiency.
1. Introduction
With the rapid development and modernization of industry and population growth, serious soil contamination with heavy metals has increased rapidly, becoming a global problem.1,2 Heavy metals cannot be degraded, which makes these toxic elements persistent in the environment and more difficult to control.3,4 Lead (Pb), which is considered the second most toxic contaminant after arsenic,5 exists widely in the ecosystems.6,7 Excessive Pb in the environment has harmful effects on plants and animals and threatens human health throughout the food chain.8,9 In China, Pb pollution is a serious environmental problem, and its concentration exceeds the recommended limits in 1.5% of agricultural land.10 Therefore, the development of efficient techniques is becoming a critical need for solving the problem of metal(loid) pollution.10,11
Compared with conventional methods, phytoremediation is an advancing technology that uses plants to remove or extract pollutants from the environment.12,13 Moreover, phytoremediation is an in situ remediation, simple, low-cost and green technology.14,15 For soil pollution, phytoextraction and phytostabilization are the two main phytoremediation strategies and are the most widely used.16 In recent years, hyperaccumulators have been widely used for soil remediation and have attracted remarkable attention.17,18 Among these species, a few hyperaccumulators are available for Pb-contaminated soil remediation.19,20 Due to the low bioavailability of Pb in soil, many species cannot uptake large quantities of Pb from the soil and translocate it from the roots to the aerial parts. Furthermore, low biomass and remediation efficiency are bottlenecks for the practical application of phytoremediation.11,17 Therefore, looking for new species for heavy metal phytoremediation is the focus of researchers.4 An ideal species for phytoremediation can tolerate and accumulate heavy metals, grow fast, and produce large aboveground biomass and deep and developed root systems. Nevertheless, relatively few species possess all of these properties.21,22 Generally, fast-growing woody species have a large aboveground biomass and deep root system compared with herbaceous plants.23,24 In addition, some woody species can accumulate considerable levels of heavy metals and translocate them to their aboveground parts.12,23 However, many researchers have indicated that Pb can be strongly bound by soil particles, especially in alkaline environments, and thus, its mobility is significantly affected.25,26 Hence, it is difficult for plants especially woody species, to absorb Pb directly from the soil and translocate it to their shoots.27
To further improve Pb removal efficiency, many chelators have been used to increase the bioavailability of Pb in soil.17,28,29 Compared with other chemosynthetic chelators, low-molecular-weight organic acids (LMWOAs) are natural compounds and are favored in metal-mobilizing agents when they are at low toxicity, readily biodegradable and eco-friendly.30–32 Such LMWOAs may increase metal mobilization in soils by promoting soil acidity and forming metal–carboxylic acid complexes,33,34 thereby enhancing heavy metal uptake and accumulation.31 Moreover, they can alleviate oxidative stress by improving the enzyme activity of the antioxidative defense system.35,36 Therefore, LMWOAs may enhance phytoremediation efficiency by promoting heavy metals uptake and accumulation by plants and improving their tolerance to heavy metals.19,20 Cai et al. reported that citric acid, malic acid, and tartaric acid application can significantly increase the Pb phytoremediation efficiency of centipedegrass.19 Similar results were also found in Solanum nigrum31 and Typha latifolia.37 However, some studies pointed out that LMWOAs could not significantly promote the uptake of Pb by plants.38,39 Moreover, the application of high concentration LMWOAs can significantly reduce the plant biomass.40 These results suggested that their efficiency rests on the concentration, method, period of use, and physiological state of the plant.11,41 Therefore, the rational application of LMWOAs can improve the efficiency of phytoremediation effectively.19
Rhus chinensis Mill is a traditional Chinese medicine and a small deciduous tree with the properties of fast growth and strong environmental adaptability.42,43 It is also a pioneer species and can grow in Pb/Zn mine tailing areas.44 Furthermore, R. chinensis is a Pb-tolerant species with the capacity to absorb and accumulate Pb in moderate or higher Pb-contaminated areas.42,43 Therefore, R. chinensis is a potential plant for Pb phytoremediation. However, previous studies mainly focused on hydroponic and short-term (one month) pot experiments.42,43 Therefore, the growth performance and Pb accumulation capacity of R. chinensis for longer periods in soil containing higher Pb concentrations are unknown. Previous studies have also demonstrated that the majority of Pb is stored in roots and that plant growth is significantly inhibited under higher Pb concentration treatments.42,43 To achieve high phytoremediation efficiency for high Pb-contaminated areas, adding LMWOAs is an effective approach. A preliminary study also confirmed that citric acid (CA) and oxalic acid (OA) were the main components of root exudates.42 Their application, along with phytoremediation, can immediately enhance lead accumulation and mitigate toxic effects. However, the effect of CA and OA on the lead phytoremediation potential of R. chinensis with significantly inhibited growth in Pb-contaminated soil are unknown. Therefore, the main objective of this work was to (1) evaluate the physiological response and metal accumulation patterns of R. chinensis grown in high Pb-treated soil for long-term; and (2) investigate the effects of different concentrations of CA and OA on the phytoremediation efficiency and subsequent biological responses of R. chinensis with significantly inhibited growth.
2. Materials and methods
2.1 Plant materials and growth conditions
Seeds were collected from Hangzhou, China (30°057′N, 119°956′E) and sown in a pot (diameter 4 cm × height 8 cm) containing perlite
:
peat (1
:
3, v/v). When the shoots and root systems of seedlings developed well, seedlings with a height of 50–60 cm and a ground diameter of 0.4–0.5 cm were selected for the experiment.
2.2 Experimental method
Red soil (0–30 cm) was collected from Fuyang District, Hangzhou (30°03′43′′N, 119°57′12′′E). Red soil is more viscous, and the effective and total lead concentrations were 24.0 and 40.8 mg kg−1, respectively. The concentrations of available phosphorus, hydrolyzed nitrogen, and available potassium were 3.53, 56.3 and 124.3 mg kg−1, respectively, and the pH value was 5.59. This soil was air-dried, sieved, and then used in the subsequent pot experiments. Based on the results of preliminary tests and field investigations in Pb/Zn mining areas, soil Pb concentrations of 0, 500, 750, 1000, 1250 and 1500 mg kg−1 were designed. Pb(NO3)2 solution was prepared and sprayed on the soil, and then the soil was equilibrated for one month. Due to losses during the experiment, the final Pb concentration was lower than the design concentration. The final Pb, available nitrogen, phosphorus and potassium concentrations and pH are listed in Table 1. In May 2016, uniform seedlings with their nutrient medium were transplanted into black cylindrical plastic pots with 3 kg of soil, and each pot contained one seedling. After 3 months of the trial, similar toxic symptoms were found in plants treated with high Pb concentrations (≥1000 mg kg−1), and seedling growth was significantly inhibited. Hence, 0.5 and 1.0 mmol L−1 CA and 0.5 and 1.0 mmol L−1 OA, respectively, were added to the 1000 mg kg−1 Pb(NO3)2 treatment to verify whether organic acids could alleviate the toxic effects of seedlings and improve the efficiency of phytoremediation. Therefore, there were 10 treatment groups in this experiment, and the 10 treatments were as follows: T0 (0 mg kg−1 Pb(NO3)2), T1 (500 mg kg−1 Pb(NO3)2), T2 (750 mg kg−1 Pb(NO3)2), T3 (1000 mg kg−1 Pb(NO3)2), T4 (1250 mg kg−1 Pb(NO3)2), T5 (1500 mg kg−1 Pb(NO3)2), T6 (1000 mg kg−1 Pb(NO3)2 and 0.5 mmol L−1 CA), T7 (1000 mg kg−1 Pb(NO3)2 and 1.0 mmol L−1 CA), T8 (1000 mg kg−1 Pb(NO3)2 and 0.5 mmol L−1 OA), T9 (1000 mg kg−1 Pb(NO3)2 and 1 mmol L−1 OA). Each treatment had 9 seedlings per replicate, for a total of 27 seedlings of R. chinensis per treatment. The experiment lasted 150 days.
Table 1 Chemical characteristics of test soil. Each value represents the mean of three replicates ± SD. The same below
Pb/(mg kg−1) |
Available Pb/(mg kg−1) |
Total Pb/(mg kg−1) |
Hydrolysable nitrogen/(mg kg−1) |
Available phosphorus/(mg kg−1) |
Available potassium/(mg kg−1) |
pH |
0 |
24.0 ± 4.7 |
40.4 ± 1.1 |
56.3 ± 2.5 |
3.53 ± 0.06 |
124.3 ± 0.6 |
5.59 ± 0.02 |
500 |
254.7 ± 9.9 |
409 ± 32.5 |
92.7 ± 2.1 |
5.62 ± 0.03 |
130.0 ± 1.0 |
5.66 ± 0.02 |
750 |
429.0 ± 11.8 |
622.3 ± 40.0 |
83.5 ± 3.1 |
5.54 ± 0.02 |
136.7 ± 0.6 |
5.43 ± 0.02 |
1000 |
630.3 ± 32.5 |
867.7 ± 19.1 |
76.8 ± 1.0 |
5.51 ± 0.02 |
135.0 ± 3.0 |
5.39 ± 0.02 |
1250 |
738.3 ± 28.4 |
1051.7 ± 51.9 |
72.9 ± 1.7 |
5.37 ± 0.02 |
133.7 ± 1.2 |
5.35 ± 0.01 |
1500 |
939.7 ± 24.9 |
1232.3 ± 33.5 |
74.9 ± 1.7 |
5.47 ± 0.02 |
134.0 ± 2.6 |
5.31 ± 0.02 |
2.3 Biomass measurements
After harvest, the leaves (leaflet and petiole), stems, roots (taproot and lateral roots) were separated. Biomass measurement and calculation of the tolerance index (TI) are detailed in a previous paper.42
2.4 Measurement of root traits and leaflet area
The traits of taproots and lateral roots from an individual plant were determined as described by Shi et al.45 The leaf area was scanned using a CI-203 laser area meter (CID Bio-Science Inc, USA).
2.5 Estimation of photosynthetic pigment concentrations
The concentrations of chlorophyll (Chl), Chl a, Chl b and carotenoids (Car) were estimated according to Poursattari and Hadi.46
2.6 Hydrogen peroxide, superoxide and MDA concentrations and antioxidative enzyme activity
The hydrogen peroxide (H2O2), superoxide (O2˙−), and malondialdehyde (MDA) concentrations in the fresh leaflets and lateral roots (0.2 g) of the control and treated seedlings were estimated according to Shi et al.47 Fresh leaflets and lateral roots (0.5 g) were used to determine antioxidative enzyme activity. The superoxide dismutase (SOD), peroxidase (POD), and catalase (CAT) activities of leaflets and lateral roots were determined as described by Amjad et al.48
2.7 Determination of Pb and sub-cellular distribution
The plant samples were cut and ground with a ball mill, and then passed through a 0.149 mm sieve to determine the Pb concentration. Each dried powdered sample (0.2 g) was digested with a 5 mL mixture (4
:
1 (v/v), 65% HNO3 and 70% HClO4). The Pb concentration was determined using inductively coupled plasma mass spectrometry (ICP-MS, Perkin Elmer NexION300D, USA).
Fresh leaflets and lateral roots (0.5 g) were used to determine the subcellular distribution. The Pb concentrations in the cell wall fraction (F1), the nucleus rich fraction (F2), the mitochondrial fraction (F3) and the soluble fraction (F4) were determined as reported by Zhou et al.43
The bioconcentration factor (BCF) and translocation factor (TF) are two important indicators for evaluating phytoremediation efficiency. They were calculated according to Li et al.17
2.8 Determination of EC50
The EC50 (concentration for 50% of maximal effect) is the metal concentration that resulted in a 50% reduction in plant biomass compared to the control.49 Critical toxicity thresholds were estimated according to Wang et al.49
2.9 Soil indicators
Soil samples were passed through 2 and 0.149 mm sieves. The former was used to determine the soil pH and the concentration of available soil Pb and K, and the latter was used to determine the total Pb concentration. The available soil Pb and K were extracted with DTPA (The components are 0.005 mol L−1 DTPA-0.01 mol L−1 CaCl2-0.1 mol L−1 TEA) and then measured using ICP-MS. The total Pb and K concentrations were determined using ICP-MS after extraction with aqua regia. The pH value of the soil sample (soil
:
distilled ratio of 1
:
2.5, v/v) was measured using a Metro-pH320 pH meter (Mettler Toledo Instruments Ltd, China). Soil organic matter, available phosphorus and hydrolyzed nitrogen were determined following the K2Cr2O7 external heating method, alkaline hydrolysis-diffusion method and Molybdenum blue colorimetric method, respectively.50
2.10 Statistical analysis
Statistical software SPSS 22.0 was used for variance analysis to test the significance of differences with Fisher's least significant difference (LSD) procedure. Pearson's correlation analysis was performed to investigate the relationship between biomass and other studied parameters. The results were standardized and subsequently calculated using R (Ver. 4.2.0, package “FactoMineR” and “factoextra”) for principal component analysis (PCA). The data were visualized using OriginPro (Ver. 9.5).
3 Results and discussion
3.1 Plant growth and tolerance index
In this study, the heights of R. chinensis did not increase after a 2 months trial in T3, T4, and T5, and toxicity symptoms, such as yellowing from the leaf tip and a reduction in leaf area, were also observed (Fig. S1†). Compared to T0, the dry biomass was significantly reduced in all Pb treatments (p < 0.01, R2 = 0.865–0.937, Table S1†), and the highest reduction recorded was 73.2% in T5 (Table 2). Compared with T0, the reduction in lateral root biomass under different treatments was lower than that in other organs and reduced by 7.9% (T1), 37.2% (T2), 39.9% (T3), 44.4% (T4) and 48.2% (T5), respectively. The reduction of the other organs' biomass was greater than 50% in all treatments, except T1. The TI values for plants in all Pb treatments were only 0.27–0.60 (Table 2). In addition, the Pb toxicity thresholds were determined for the leaves, stems, and roots (Table S2†). All EC50 values were 709.0 mg kg−1 (roots), 565.4 mg kg−1 (stems) and 756.4 mg kg−1 (leaves). Pb is not an essential nutrient for plants and its threshold value in plants is very low.51 Thus, it is not surprising that the biomass of R. chinensis decreased and that visual damage under high Pb stress was observed in this study. Correlation analysis also verified that dry weight yield was negatively correlated with Pb concentrations in all organs (Fig. S2†). The results were similar to the findings of the dry biomass in Chenopodium quinoa,48 Sophora alopecuroides,2 and Festuca arundinacea6 which decreased at a higher Pb stress. However, compared to the other species, the TI values were lower, especially under a higher Pb concentration.20,52,53 This might be due to the soil pH value in this study being 5.31–5.66, making the available Pb concentration significantly higher than that in other studies. Generally, the availability of heavy metals is very important for the phytoremediation of contaminated soils.54 However, the high mobility of Pb in soil can also affect plant growth.
Table 2 Mean harvest dry weights (g) of the component parts, the root-to-shoot ratio, and the tolerance index (TI) in R. chinensis under different treatments; different letters indicate significant difference between the treatments (p < 0.05). The same below
Treatment |
Petiole |
Leaflet |
Stem |
Taproot |
Lateral root |
Root/shoot |
TI |
T0 |
2.28 ± 0.18 a |
7.10 ± 0.46 a |
9.73 ± 0.37 a |
4.58 ± 0.05 a |
1.57 ± 0.24 a |
0.32 ± 0.01 bc |
— |
T1 |
1.05 ± 0.07 b |
4.97 ± 0.21 b |
5.11 ± 0.49 b |
2.65 ± 0.79 b |
1.45 ± 0.21 a |
0.37 ± 0.06 b |
0.60 ± 0.06 a |
T2 |
1.07 ± 0.19 b |
3.89 ± 0.93 bc |
4.45 ± 0.55 b |
1.49 ± 0.10 defg |
0.99 ± 0.13 b |
0.26 ± 0.02 c |
0.47 ± 0.04 b |
T3 |
0.98 ± 0.17 bc |
1.81 ± 0.29 e |
3.20 ± 0.60 c |
1.30 ± 0.25 efg |
0.94 ± 0.09 b |
0.38 ± 0.06 b |
0.33 ± 0.05 cde |
T4 |
0.76 ± 0.18 cd |
2.03 ± 0.42 de |
3.04 ± 0.46 c |
1.22 ± 0.28 fg |
0.87 ± 0.16 b |
0.36 ± 0.04 b |
0.31 ± 0.06 de |
T5 |
0.53 ± 0.10 d |
1.71 ± 0.28 e |
2.64 ± 0.39 c |
1.05 ± 0.20 g |
0.81 ± 0.14 b |
0.39 ± 0.09 b |
0.27 ± 0.02 e |
T6 |
0.89 ± 0.17 bc |
1.96 ± 0.32 de |
3.16 ± 0.26 c |
2.17 ± 0.30 bc |
1.44 ± 0.08 a |
0.60 ± 0.05 a |
0.38 ± 0.04 c |
T7 |
0.84 ± 0.08 bc |
1.99 ± 0.09 de |
3.00 ± 0.60 c |
1.88 ± 0.10 cd |
1.17 ± 0.16 ab |
0.53 ± 0.08 a |
0.35 ± 0.01 cd |
T8 |
0.91 ± 0.17 bc |
2.02 ± 0.07 de |
3.27 ± 0.63 c |
1.60 ± 0.15 def |
0.93 ± 0.05 b |
0.41 ± 0.03 b |
0.35 ± 0.03 cd |
T9 |
1.10 ± 0.18 b |
3.13 ± 0.46 cd |
4.42 ± 0.58 b |
1.81 ± 0.13 cde |
1.01 ± 0.11 b |
0.33 ± 0.04 bc |
0.45 ± 0.04 b |
F |
27.463 |
53.034 |
51.918 |
33.785 |
10.303 |
9.855 |
19.227 |
p |
<0.001 |
<0.001 |
<0.001 |
<0.001 |
<0.001 |
<0.001 |
<0.001 |
The addition of different concentrations of CA and OA into the T3 growth medium ameliorated Pb toxicity in R. chinensis. However, the leaf area from T6 to T9 also decreased compared to T3, especially in T8 (Fig. S1†). Nevertheless, exogenous organic acids increased the leaflet biomass, indicating that they delayed the senescence process of R. chinensis under Pb stress. This phenomenon could also be explained by the fact that exogenous organic acids can compensate for the reduction of photosynthesis.1 Furthermore, CA and OA application significantly increased the root biomass of seedlings compared to T3 (Table 2). The Pb-stressed seedlings treated with CA exhibited a decrease in the biomass of stem and petiole, respectively, compared with T3. The biomass of the stem under 1.0 mM OA treatments increased significantly (p < 0.05), and those treated with 0.5 mM OA were not significantly different from those of T3 (Table 2). The biomass under organic acid treatments was lower than that under T0 (p < 0.05). The TI values of exogenous CA and OA treatments were 0.35–0.45, which were higher than those of the T3 (0.33) (Table 2). The biomass of R. chinensis was increased through the addition of CA and OA, although marginally relative to T3. This might be because CA and OA are the main constituents of R. chinensis root exudates and could decrease the toxic effects of free Pb ions as a result of organic acids providing vast amounts of small molecule ligands that induce metal chelation to form lower toxicity metal–organic complexes.32,55 Moreover, the cause of this phenomenon probably lies in the fact that organic acids promote the uptake of nutrients in soils.11 However, the results also showed that the organic acid treatments had different effects in mitigating toxicity. This difference might be due to the dose and type of organic acids.
3.2 Biomass allocation
After the experiment, the biomass allocation of R. chinensis was significantly different (p < 0.05, Table 2), and the lowest root-to-shoot ratio was found in T2. The root-to-shoot ratios in T6 and T7 were significantly higher than those in other treatments, and the values were 0.60 and 0.53, respectively. However, there were no statistically significant differences between the T3 and OA treatments according to the root-to-shoot ratio. Generally, plants under unfavorable conditions often adapt by altering their biomass allocation patterns.1 In this study, the root-to-shoot ratio in Pb and organic acid treatments increased, except in T2. Thus, maintaining a certain level of biomass accumulation and steadily allocating more biomass to the root system is one of the reasons for R. chinensis to realize long-term adaptation in Pb-contaminated soil.1 In the Pb treatments with combined CA and OA applications, there was a difference in the pattern of biomass allocation. After applying CA, a similar strategy of increasing root biomass allocation was found in this study. However, the root-to-shoot ratio decreased with increasing CA concentration, which might be related to the fact that a higher CA concentration was more likely to increase Pb availability in the soil, ultimately affecting root system development. When OA was added in T3, the opposite strategy of distributing more biomass to shoots was observed. This might be because OA could precipitate in the soil in the form of PbC2O4, which reduces free Pb2+ ions in soil, thus decreasing Pb concentrations in the roots to meet the demands of leaves for photosynthetic assimilates. Increasing the shoot biomass, especially leaflet biomass, compensates for the reduction in photosynthesis and thus promotes R. chinensis growth.1
3.3 Photosynthetic pigment concentration
Both Chl a, Chl b and Chl concentrations were reduced with the increase in Pb concentrations (R2 = 0.930–0.947, Table S1†), and the highest reduction recorded was 33.6, 56.3, and 42.3%, respectively, in T5. When CA and OA were added to 1000 mg kg−1 Pb, the Chl concentration increased except in T7 with respect to T3 (Table S3†). In this study, biomass was positively correlated with Chl concentration (Fig. S2†), indicating that chlorophyll is an important component of plant growth.29 In this study, the decrease in the chlorophyll concentration of R. chinensis under Pb exposure was a manifestation of impaired photosynthesis.11,26 However, there were no statistically significant differences between the organic acid treatments and T3 in terms of chlorophyll concentration (Table S3†). This signified that the addition of CA and OA could alleviate the toxic effects of plants whose growth had been severely inhibited, but the results were not satisfactory. In addition, based on the chlorophyll concentration, it is speculated that one of the reasons for the increased biomass accumulation may be that exogenous LWMOAs promote photosynthesis.19,56 Simultaneously, the chlorophyll concentration of organic acid treatments was in the order of T9 > T8 > T6 > T7 (Table S3†). These results also further demonstrated that the application of oxalic acid could better promote plant shoot development.
Overall, the carotenoid concentration was increased with an increasing Pb dose in this study (R2 = 0.899, Table S1 and S3†). Moreover, the carotenoid concentrations of T6–T9 were higher than those of T0. Biswas et al.52 and González-Peña et al.57 indicated that non-enzymatic antioxidants, including carotenoids, can scavenge oxygen species (ROS) under heavy metal stress. Therefore, the carotenoid concentration increased with increasing Pb dosage in this study. However, variance analysis also showed that there were no significant differences between the organic acid treatments and T3. These results indicate that the non-enzymatic antioxidant carotenoids play a lesser mitigating role with the addition of CA and OA.
3.4 H2O2, O2˙− and MDA concentration
Typically, Pb can induce excessive ROS production, causing severe oxidative damage in plants.4,51 In the present study, the MDA, H2O2, and O2˙− concentrations of leaflets increased with increasing Pb dosage, especially in T3–T5 (R2 = 0.888–0.976, Table S1†). Overall, Pb stress (T1–T5) increased leaflet MDA, H2O2, and O2˙− accumulation by 1.27–2.14 folds, 1.12–1.76 fold, and 1.13–1.88 fold, respectively, compared to T0. Similar to the leaflets, the MDA, H2O2, and O2˙− concentrations of the lateral roots were also significantly elevated with Pb treatment alone (Fig. 1). Furthermore, correlation analysis revealed a very significant positive correlation between the tissues for MDA, H2O2, and O2˙− concentration and Pb concentration and a significant negative correlation with biomass (Fig. S2†). Most studies have shown that LMWOAs alleviate the toxicity of heavy metals in plants by reducing the level of membrane lipid peroxidation.19,58 In the present study, this phenomenon was also observed. The MDA, H2O2, and O2˙− concentrations of leaflets and lateral roots in T6–T9 decreased compared to T3, except in T7 and T8 in leaflets (Fig. 1), suggesting that CA and OA could ameliorate oxidative stress in plants through complexation with Pb.32,59
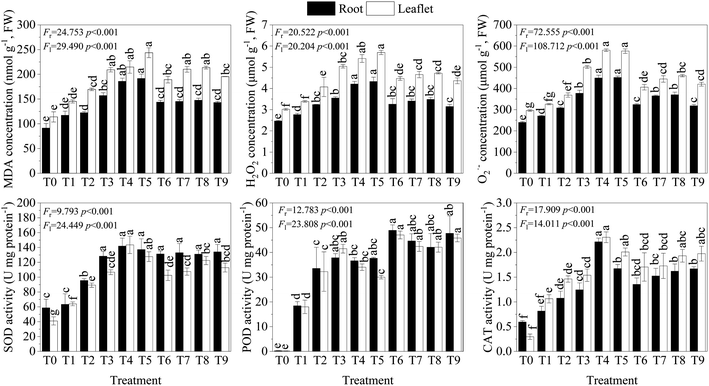 |
| Fig. 1 Effects of different Pb and organic acid treatments on antioxidant enzyme (SOD, POD and CAT) activity and MDA H2O2, and O2˙− concentration in R. chinensis. Data over bars marked by the same letters are not significantly different at p < 0.05. Each value represents the mean of three replicates ± SE. The same below. | |
3.5 Antioxidant activities in R. chinensis
To protect against the damage caused by elevated ROS levels, plants possess certain defense mechanisms, such as antioxidative systems, which have enzymatic and non-enzymatic systems.51 Among these, the enzymatic antioxidant system of plants is one of the most effective mechanisms for coping with the toxic effects of free radicals.6 In this study, antioxidant activity (SOD, POD, and CAT) was significantly stimulated in solo Pb treatment (T1–T5) in relation to the control (T0), except for SOD and CAT activity of lateral roots in T1 (Fig. 1). Except for the POD activity of the leaves, all of them can be described using a linear equation (p < 0.05, R2 = 0.752–0.794, Table S1†). The highest values of SOD and CAT activity were found in T4, and the highest value of POD activity was observed in T3. Additionally, in this study, biomass was negatively correlated with antioxidant enzyme activities (Fig. S2†). These phenomena are in line with findings in Festuca arundinacea,6 Trifolium pratense.9 Furthermore, the activities of SOD, POD, and CAT showed a low–high–low trend with the increase in Pb concentration. This may be due to the oxidative stress induced by the high Pb concentration, which exceeds the ability of the antioxidant system to cope.11,60 Many studies have indicated that under heavy metal stress, LMWOAs can help plants mitigate damage by improving the activity of antioxidant enzymes.32,36 In this study, the application of organic acids also notably stimulated antioxidant activities in R. chinensis compared with T0 (Fig. 1). Although variance analysis also showed that there were no significant differences between organic acid treatments and T3 according to antioxidative enzyme activity, the POD and CAT activities in leaflets and lateral roots in organic acid treatments increased by 10–30% compared with T3 (Fig. 1). These results indicate that CA and OA can help R. chinensis mitigate damage by improving the activity of antioxidant enzymes.32,36
3.6 Accumulation and translocation of Pb
Linear regression analysis revealed that Pb accumulation was strongly dependent on Pb concentration in the soil in the case of each stem and root (R2 = 0.861 and 0.872, respectively, Table 3 and S1†). The Pb concentration across organs decreased in the order lateral roots > petiole > stem > taproot > leaves. Rhus chinensis accumulated more Pb in T4 than in the other Pb treatments. Moreover, only a small quantity of Pb was translocated to the shoots, and the BCF of shoots and TF values of R. chinensis were less than 0.2 and 1.0, respectively (Fig. S3†). Under similar Pb concentration treatments, the Pb concentration in the roots of R. chinensis was higher than that of other species.1,51,54,61 This might be related to the lower soil pH in this experiment. Generally, higher Pb solubility with lower soil pH is therefore available for plant uptake.7,54 Nevertheless, the highest Pb concentration did not exceed 1000 mg kg−1 in the lateral roots in T4 and T6, and the root BCF values were less than 0.5 in this study (Table 3 and Fig. S3†). However, previous studies have shown that R. chinensis can accumulate more than 1000 Pb mg kg−1 in roots under higher Pb stress in the relatively short term.42,43 The difference indicated that it was difficult for R. chinensis to uptake a large amount of Pb under long-term stress with a high concentration of available Pb. In the current study, only a small quantity of Pb was translocated to shoots (Fig. S3†), which is similar to previous studies42,43 and other non-hyperaccumulator plants.4,6,9,31 One possible explanation is the exclusion strategy in plants.29
Table 3 The Pb concentration (mg kg−1) in R. chinensis under different treatments
Treatment |
Petiole |
Leaflet |
Stem |
Taproot |
Lateral root |
T0 |
1.23 ± 0.40 h |
0.93 ± 0.32 f |
1.20 ± 0.17 d |
1.97 ± 0.57 f |
4.43 ± 0.50 e |
T1 |
109.87 ± 3.23 f |
24.60 ± 2.38 e |
67.39 ± 3.19 c |
23.73 ± 1.64 ef |
242.70 ± 41.10 d |
T2 |
119.87 ± 3.11 e |
27.10 ± 8.90 de |
61.47 ± 3.01 c |
33.90 ± 8.43 de |
396.80 ± 61.10 c |
T3 |
123.60 ± 2.50 e |
38.30 ± 8.49 cd |
73.54 ± 1.44 c |
58.53 ± 4.81 bcd |
583.90 ± 93.00 b |
T4 |
133.33 ± 1.80 d |
43.60 ± 8.74 c |
97.31 ± 8.04 b |
82.13 ± 7.69 ab |
808.80 ± 33.10 a |
T5 |
102.93 ± 3.78 g |
27.40 ± 10.83 de |
94.80 ± 15.50 b |
95.20 ± 32.00 a |
557.80 ± 64.50 b |
T6 |
246.13 ± 2.41 a |
87.10 ± 9.61 a |
117.08 ± 12.03 a |
72.40 ± 30.30 abc |
896.00 ± 81.50 a |
T7 |
136.80 ± 1.74 d |
48.70 ± 3.06 c |
118.03 ± 7.51 a |
94.67 ± 2.41 a |
575.10 ± 96.10 b |
T8 |
144.00 ± 8.27 c |
46.37 ± 1.35 c |
103.23 ± 16.74 ab |
62.53 ± 1.01 bc |
358.47 ± 6.65 c |
T9 |
155.47 ± 1.67 b |
63.20 ± 3.00 b |
96.75 ± 1.21 b |
50.00 ± 4.85 cd |
525.00 ± 37.00 b |
F |
865.021 |
35.767 |
44.482 |
13.316 |
55.609 |
p |
<0.001 |
<0.001 |
<0.001 |
<0.001 |
<0.001 |
The application of LMWOAs significantly increased the Pb concentration in plants.20,31,37 However, many studies have suggested that LMWOAs have no significant effect on plant Pb uptake.38,39 In this study, the Pb concentration in the lateral roots, stem, petiole, and leaflets in T6 increased significantly by 1.53, 1.59, 1.99, and 2.27 times compared with T3. The Pb concentration in T7 also increased significantly compared with T3, except in the lateral roots. The Pb concentrations of the aboveground parts of R. chinensis in T8 and T9 increased significantly compared with that of T3, while the Pb concentrations in the roots were slightly reduced in T9 and significantly decreased in T8. In addition, while CA and OA applications significantly increased the TF values compared to T3, the TF values were 0.32 (T6), 0.33 (T7), 0.54 (T8), and 0.42 (T9) (Fig. S3†). The cause of this phenomenon probably lies in the fact that exogenous LMWOAs promote radial transport of Pb in roots.19 In general, the increase in Pb accumulation and translocation might be attributed to the acidifying soil pH. However, after the experiment, there was no statistically significant difference in pH among the treatments (pH: 4.75–4.86), which is consistent with the conclusion of many studies indicating that the application of LMWOAs has little effect on the pH of contaminated soil.31,62 Therefore, the complexation of CA and OA plays a major role in promoting the bioavailability and mobility of Pb in the soil. The current study showed that CA and OA have different performances in Pb accumulation and translocation in plants. Overall, the Pb concentration in the organs of R. chinensis in OA treatment was lower than that in CA treatment, especially in the roots. The difference in efficiency between CA and OA was due to the following three aspects: first, the different complexation ability of the two organic acids in Pb. Second, the different degree of H+ provided by CA and OA, and then, the different effects on soil pH in the short term. Third, the heavy metal concentrations in the roots were significantly different in different order roots.63 Generally, low-order roots are responsible for water and nutrient uptake.64 In this study, the development of lateral roots under CA treatment was significantly better than that under OA treatment (Table 2 and S4†), which might explain why CA treatment generally had a higher Pb concentration than OA treatment in this study. Simultaneously, with the further increase in the CA concentration in the soil, the Pb concentration in organs decreased, which might be attributed to the fact that a high CA concentration increased phytotoxicity to plants.65 OA showed the opposite trend. A low concentration of oxalic acid is easily adsorbed by aggregates and organic matter in soil and has a poor activation effect on Pb in soil.
3.7 Sub-cellular distribution of Pb
Compared to T0, the ratio of Pb distributed in F1 significantly decreased in the Pb treatment alone (Fig. 2B and D). In addition, the proportions of Pb deposited in the mitochondrial fraction and soluble fraction increased in the leaflets (Fig. 2B). In the lateral roots, the proportions of trophoplasts and soluble fractions also increased with respect to T0 (Fig. 2D). Furthermore, a large amount of Pb remained and was immobilized in the cell wall or vacuole, and the biomass was closely related to the subcellular distribution ratio of Pb. Similar results were also found in Eremochloa ophiuroides19 and Sasa argenteostriata66 under Pb stress. Therefore, the storage of Pb in the cell wall and soluble fractions would help R. chinensis alleviate Pb toxicity.42,43 Compared with T3, the organic acid treatment did not significantly change the subcellular distribution of Pb in the leaflets and lateral roots, except leaflets in T6 (Fig. 2). One reason could be that organic acids can be degraded in the soil in a shorter time.
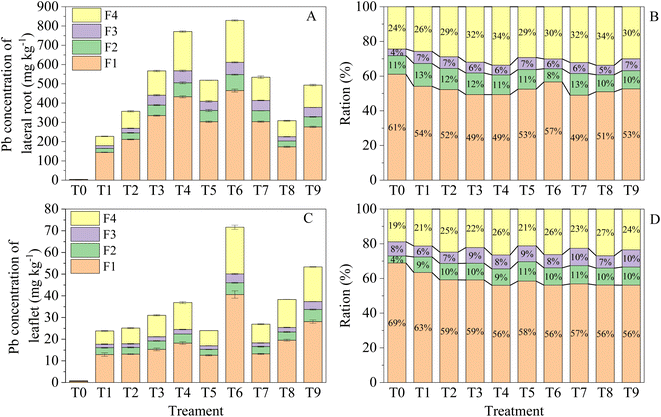 |
| Fig. 2 Pb subcellular distribution in R. chinensis under different treatments. (A) and (C): Pb concentrations of four subcellular fractions in lateral root and leaflet tissues, respectively. (B) and (D): Pb distribution ratios of four subcellular fractions in lateral root and leaflet tissues, respectively. F1: cell wall fraction; F2: nucleus rich fraction; F3: mitochondrial fraction; F4: the soluble fraction. | |
3.8 Phytoremediation efficiency
Phytoremediation efficiency is related to biomass production and heavy metal concentrations in plants; the total content of heavy metals accumulated by plants is the more important factor for evaluating phytoremediation efficiency.4,20,67 In this study, Pb accumulation in acidic soil without chelator application ranged between 0.91 and 1.29 mg per plant, and the highest Pb content was observed in T4 due to the highest concentration in tissues (Table S5†). Moreover, the highest Pb content in leaflets was found in T1 because its biomass production was significantly higher than that of the other treatments (Table S5†). Compared with other studies, the Pb content of R. chinensis in this study was relatively high.1,51 The application of CA and OA increased Pb accumulation, except in T8 (Table S5†). This was mainly due to the biomass significantly increasing as the Pb concentration changed slightly. By comparing both chelators, 0.5 mM CA performed better in enhancing Pb accumulation, and the Pb content was 2.20 mg per plant. Exogenous 1.0 mM OA showed the best promoting effects on Pb transport, and the shoot Pb content was 0.79 mg per plant. Therefore, the application of 0.5 mM CA and 1.0 mM OA can obtain twice the phytoremediation efficiency.
3.9 PCA analyses
To discuss the responses of R. chinensis to exposure to Pb and Pb + organic acids, PCA analysis was performed using the biomass, leaf area, root length, ROS, antioxidant, and Pb concentration data from all organs. According to the PCA results, two principal components explained 82.85% of the total variance (Table S6†). Table S6† shows that the first component represented 72.22% of the total variance and was positively linked to the Pb concentration in the roots and stems, carotenoid concentration, membrane lipid peroxidation levels, and antioxidase activity in leaflets and negatively linked to biomass (except the lateral roots), leaf area, lateral root length, and chlorophyll concentration. PC2 accounted for 10.63% of the total variance and was positively linked to the biomass of the lateral roots, Pb concentration in leaflets and petiole. PC1 delineated the effect of Pb treatments, and PC2 reflected variations in the influence of Pb combined with organic acid treatments (Fig. 3). These results further indicate that exogenous LMWOAs actively regulate the physiological growth process of R. chinensis under Pb stress in different ways. Their positive effects were reduced in the order of T6 > T9 > T7 > T8 (Fig. 3).
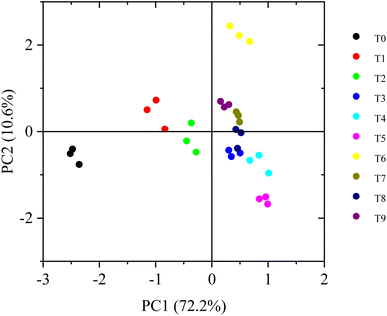 |
| Fig. 3 Principal component analysis of physiological growth and Pb accumulation indexes under different Pb and organic acid treatments. | |
4. Conclusion
In short, the toxic effects of exogenous Pb exhibited a concentration-dependent pattern. Rhus chinensis allocates more biomass to the roots and promotes lateral root development. In addition, we found that R. chinensis retained a large amount of Pb in the cell walls of tissues, which probably improved the tolerance in Pb-contaminated soil. Furthermore, to protect against the damage, SOD, POD, and CAT were significantly stimulated in all treatments. Our findings indicate that organic acids can use two different strategies of biomass allocation and Pb accumulation and distribution to mitigate Pb stress. Citric acid application increased Pb accumulation in roots and shoots and promoted root system development. Oxalic acid improved shoot biomass production and the capacity for Pb translocation. The order of their promoting effects was 0.5 mM CA > 1.0 mM OA > 1.0 mM CA = 0.5 mM OA. Therefore, the combined application of 0.5 mM CA and 1.0 mM OA in R. chinensis could effectively remediate Pb-contaminated soil.
Ethical statement
The authors declare that there is no conflicts of interest exists among fundings, and no Human Participants and/or Animals involved.
Author contributions
Xiang Shi: conceptualization, methodology, original draft preparation. Shufeng Wang: methodology. Wenxiang He: investigation. Yangdong Wang: reviewing and editing.
Conflicts of interest
The authors declare that they have no conflict of interest.
Acknowledgements
This work was supported by the National Natural Science Funds of China (No. 31870583).
References
- X. Y. Cai, M. Y. Jiang, J. R. Liao, Y. X. Yang, N. F. Li, Q. B. Cheng, X. Li, H. X. Song, Z. H. Luo and S. L. Liu, Biomass allocation strategies and Pb-enrichment characteristics of six dwarf bamboos under soil Pb stress, Ecotoxicol. Environ. Saf., 2021, 207, 111500 CrossRef CAS PubMed.
- S. Cheraghi-Aliakbari, A. Beheshti-Alagha, F. Ranjbar and I. Nosratti, Comparison of Myagrum perfoliatum and Sophora alopecuroides in phytoremediation of Cd-and Pb-contaminated soils: a chemical and biological investigation, Chemosphere, 2020, 259, 127450 CrossRef CAS PubMed.
- I. Diarra, K. K. Kotra and S. Prasad, Assessment of biodegradable chelating agents in the phytoextraction of heavy metals from multi-metal contaminated soil, Chemosphere, 2021, 273, 128483 CrossRef CAS PubMed.
- Y. X. Yang, J. R. Liao, Y. H. Chen, Y. Tian, Q. B. Chen, S. P. Gao, Z. H. Luo, X. F. Yu, T. Lei and M. Y. Jiang, Efficiency of heterogeneous chelating agents on the phytoremediation potential and growth of Sasa argenteostriata (Regel) EG Camus on Pb-contaminated soil, Ecotoxicol. Environ. Saf., 2022, 238, 113603 CrossRef CAS PubMed.
- U. Zulfiqar, M. Farooq, S. Hussain, M. Maqsood, M. Hussain, M. Ishfaq, M. Ahmad and M. Z. Anjum, Lead toxicity in plants: impacts and remediation, J. Environ. Manage., 2019, 250, 109557 CrossRef CAS PubMed.
- J. Zhang, Y. G. Qian, Z. B. Chen, M. Amee, H. Niu, D. Y. Du, J. Yao, K. Chen, L. Chen and J. Sun, Lead-induced oxidative stress triggers root cell wall remodeling and increases lead absorption through esterification of cell wall polysaccharide, J. Hazard. Mater., 2020, 385, 121524 CrossRef CAS PubMed.
- S. Collin, A. Baskar, D. M. Geevarghese, M. N. V. S. Ali, P. Bahubali, R. Choudhary, V. Lvov, G. I. Tovar, F. Senatov and S. Koppala, Bioaccumulation of Lead (Pb) and its effects in plants: a review, J. Hazard. Mater. Lett., 2022, 100064 CrossRef CAS.
- F. Chen, M. Aqeel, M. F. Maqsood, N. Khalid, M. K. Irshad, M. Ibrahim, N. Akhter, M. Afzaal, J. Ma and M. Hashem, Mitigation of lead toxicity in Vigna radiata genotypes by silver nanoparticles, Environ. Pollut., 2022, 308, 119606 CrossRef CAS PubMed.
- L. D. Meng, Y. P. Yang, Z. W. Ma, J. W. Jiang, X. M. Zhang, Z. R. Chen, G. W. Cui and X. J. Yin, Integrated physiological, transcriptomic and metabolomic analysis of the response of Trifolium pratense L. to Pb toxicity, J. Hazard. Mater., 2022, 436, 129128 CrossRef CAS PubMed.
- J. M. Guo, G. D. Zheng, J. X. Yang, T. B. Chen, X. F. Meng and T. X. Xia, Safe utilization of cadmium-and lead-contaminated farmland by cultivating a winter rapeseed/maize rotation compared with two phytoextraction approaches, J. Environ. Manage., 2022, 304, 114306 CrossRef CAS PubMed.
- Y. Q. Liang, X. Y. Xiao, Z. H. Guo, C. Peng, P. Zeng and X. Y. Wang, Co-application of indole-3-acetic acid/gibberellin and oxalic acid for phytoextraction of cadmium and lead with Sedum alfredii Hance from contaminated soil, Chemosphere, 2021, 285, 131420 CrossRef CAS PubMed.
- W. D. Yang, D. Liu, Y. Y. Wang, B. Hussain, F. L. Zhao, Z. L. Ding, X. E. Yang, Z. Q. Zhu and M. Dawood, Variations in phytoremediation potential and phytoavailability of heavy metals in different Salix genotypes subjected to seasonal flooding, J. Environ. Manage., 2021, 299, 113632 CrossRef CAS PubMed.
- L. J. Yan, Q. V. Le, C. Sonne, Y. F. Yang, H. Yang, H. P. Gu, N. L. Ma, S. S. Lam and W. X. Peng, Phytoremediation of radionuclides in soil, sediments and water, J. Hazard. Mater., 2020, 407, 124771 CrossRef PubMed.
- J. J. Espada, R. Rodríguez, V. Gari, P. Salcedo-Abraira and L. F. Bautista, Coupling phytoremediation of Pb-contaminated soil and biomass energy production: a comparative life cycle assessment, Sci. Total Environ., 2022, 840, 156675 CrossRef CAS PubMed.
- M. Kamaruzzaman, S. Abdullah, H. A. Hasan, M. Hassan, A. Othman and M. Idris, Characterisation of Pb-resistant plant growth-promoting rhizobacteria (PGPR) from Scirpus grossus, Biocatal. Agric. Biotechnol., 2020, 23, 101456 CrossRef.
- X. Shen, M. Dai, J. W. Yang, L. Sun, X. Tan, C. S. Peng, I. Ali and I. Naz, A critical review on the phytoremediation of heavy metals from environment: performance and challenges, Chemosphere, 2021, 132979 Search PubMed.
- Y. Q. Li, Y. J. Wang, M. A. Khan, W. X. Luo, Z. C. Xiang, W. J. Xu, B. Zhong, J. W. Ma, Z. Q. Ye and Y. W. Zhu, Effect of plant extracts and citric acid on phytoremediation of metal-contaminated soil, Ecotoxicol. Environ. Saf., 2021, 211, 111902 CrossRef CAS PubMed.
- S. Ashraf, Q. Ali, Z. A. Zahir, S. Ashraf and H. N. Asghar, Phytoremediation: environmentally sustainable way for reclamation of heavy metal polluted soils, Ecotoxicol. Environ. Saf., 2019, 174, 714–727 CrossRef CAS PubMed.
- X. Y. Cai, J. Y. Fu, X. Li, L. L. Peng, L. Q. Yang, Y. H. Liang, M. Y. Jiang, J. Ma, L. X. Sun and B. M. Guo, Low-molecular-weight organic acid-mediated tolerance and Pb accumulation in centipedegrass under Pb stress, Ecotoxicol. Environ. Saf., 2022, 241, 113755 CrossRef CAS PubMed.
- I. Gul, M. Manzoor, J. Kallerhoff and M. Arshad, Enhanced phytoremediation of lead by soil applied organic and inorganic amendments: Pb phytoavailability, accumulation and metal recovery, Chemosphere, 2020, 258, 127405 CrossRef CAS PubMed.
- H. Ali, E. Khan and M. A. Sajad, Phytoremediation of heavy metals-concepts and applications, Chemosphere, 2013, 91, 869–881 CrossRef CAS PubMed.
- J. Y. Yang, Y. Q. Huang, G. J. Zhao, B. Q. Li, X. S. Qin, J. C. Xu and X. Li, Chemosphere, 2022, 296, 134045 CrossRef CAS PubMed.
- Z. B. Luo, J. L. He, A. Polle and H. Rennenberg, Heavy metal accumulation and signal transduction in herbaceous and woody plants: paving the way for enhancing phytoremediation efficiency, Biotechnol. Adv., 2016, 34, 1131–1148 CrossRef CAS PubMed.
- M. O. Mendez and R. M. Maier, Phytostabilization of mine tailings in arid and semiarid environments—an emerging remediation technology, Environ. Health Perspect., 2008, 116, 278–283 CrossRef CAS PubMed.
- I. Gul, M. Manzoor, N. Hashim, G. M. Shah, S. P. T. Waani, M. Shahid, V. Antoniadis, J. Rinklebe and M. Arshad, Challenges in microbially and chelate-assisted phytoextraction of cadmium and lead–a review, Environ. Pollut., 2021, 287, 117667 CrossRef CAS PubMed.
- L. W. Wang, D. Y. Hou, Z. T. Shen, J. Zhu, X. Y. Jia, Y. S. Ok, F. M. Tack and J. Rinklebe, Field trials of phytomining and phytoremediation: a critical review of influencing factors and effects of additives, Crit. Rev. Environ. Sci. Technol., 2020, 50, 2724–2774 CrossRef.
- S. Akhtar, Z. I. Khan, K. Ahmad, M. Nadeem, A. Ejaz, M. I. Hussain and M. A. Ashraf, Assessment of lead toxicity in diverse irrigation regimes and potential health implications of agriculturally grown crops in Pakistan, Agric. Water Manag., 2022, 271, 107743 CrossRef.
- M. M. Hasan, M. N. Uddin, I. Ara-Sharmeen, H. F. Alharby, Y. Alzahrani, K. R. Hakeem and L. Zhang, Assisting phytoremediation of heavy metals using chemical amendments, Plants, 2019, 8, 295 CrossRef CAS PubMed.
- C. Q. Xiao, S. Y. Guo, Q. Wang and R. Chi, Enhanced reduction of lead bioavailability in phosphate mining wasteland soil by a phosphate-solubilizing strain of Pseudomonas sp., LA, coupled with ryegrass (Lolium perenne L.) and sonchus (Sonchus oleraceus L.), Environ. Pollut., 2021, 274, 116572 CrossRef CAS PubMed.
- B. Li, M. M. Duan, X. B. Zeng, Q. Zhang, C. Xu, H. H. Zhu, Q. H. Zhu and D. Y. Huang, Effects of composited organic mobilizing agents and their application periods on cadmium absorption of Sorghum bicolor L. in a Cd-contaminated soil, Chemosphere, 2021, 263, 128136 CrossRef CAS PubMed.
- R. Han, H. P. Dai, L. D. Skuza and S. H. Wei, Comparative study on different organic acids for promoting Solanum nigrum L. hyperaccumulation of Cd and Pb from the contaminated soil, Chemosphere, 2021, 278, 130446 CrossRef CAS PubMed.
- Z. H. Yang, B. H. Xue, G. L. Song and S. Shi, Effects of citric acid on antioxidant system and carbon–nitrogen metabolism of Elymus dahuricus under Cd stress, Ecotoxicol. Environ. Saf., 2022, 233, 113321 CrossRef CAS PubMed.
- M. A. Khan, S. Khan, A. Khan and M. Alam, Soil contamination with cadmium, consequences and remediation using organic amendments, Sci. Total Environ., 2017, 601, 1591–1605 CrossRef PubMed.
- M. Piri, E. Sepehr and Z. Rengel, Citric acid decreased and humic acid increased Zn sorption in soils, Geoderma, 2019, 341, 39–45 CrossRef CAS.
- N. Ilyas, N. Akhtar, H. Yasmin, S. Sahreen, Z. Hasnain, P. Kaushik, A. Ahmad and P. Ahmad, Efficacy of citric acid chelate and Bacillus sp. in amelioration of cadmium and chromium toxicity in wheat, Chemosphere, 2022, 290, 133342 CrossRef CAS PubMed.
- R. Rathika, A. Y. Khalifa, P. Srinivasan, L. Praburaman, S. Kamala-Kannan, T. Selvankumar, W. Kim and M. Govarthanan, Effect of citric acid and vermi-wash on growth and metal accumulation of Sorghum bicolor cultivated in lead and nickel contaminated soil, Chemosphere, 2020, 243, 125327 CrossRef CAS PubMed.
- W. Amir, M. Farid, H. K. Ishaq, S. Farid, M. Zubair, H. F. Alharby, A. A. Bamagoos, M. Rizwan, N. Raza and K. R. Hakeem, Accumulation potential and tolerance response of Typha latifolia L. under citric acid assisted phytoextraction of lead and mercury, Chemosphere, 2020, 257, 127247 CrossRef CAS PubMed.
- X. Y. Chen, Q. Lin, Y. M. Luo, Y. F. He, S. J. Zhen, G. M. Tian and M. H. Wong, The role of citric acid on the phytoremediation of heavy metal contaminated soil, Chemosphere, 2003, 50, 807–811 CrossRef PubMed.
- P. Romkens, L. Bouwman, J. Japenga and C. Draaisma, Potentials and drawbacks of chelate-enhanced phytoremediation of soils, Environ. Pollut., 2002, 116, 109–121 CrossRef CAS PubMed.
- Y. Q. Li, Y. J. Wang, M. A. Khan, W. X. Luo, Z. C. Xiang, W. J. Xu, B. Zhong, J. W. Ma, Z. Q. Ye, Y. W. Zhu, L. L. Duan and D. Liu, Effect of plant extracts and citric acid on phytoremediation of metal-contaminated soil, Ecotoxicol. Environ. Saf., 2021, 211, 111902 CrossRef CAS PubMed.
- D. Guo, A. Ali, C. Y. Ren, J. Du, R. H. Li, A. H. Lahori, R. Xiao, Z. Y. Zhang and Z. Q. Zhang, EDTA and organic acids assisted phytoextraction of Cd and Zn from a smelter contaminated soil by potherb mustard (Brassica juncea, Coss) and evaluation of its bioindicators, Ecotoxicol. Environ. Saf., 2019, 167, 396–403 CrossRef CAS PubMed.
- X. Shi, S. F. Wang, D. X. Wang, H. J. Sun, Y. T. Chen, J. F. Liu and Z. P. Jiang, Woody species Rhus chinensis Mill. seedlings tolerance to Pb: physiological and biochemical response, J. Environ. Sci., 2019, 78, 63–73 CrossRef CAS PubMed.
- C. F. Zhou, M. Y. Huang, H. J. Ren, J. D. Yu, J. M. Wu and X. Q. Ma, Bioaccumulation and detoxification mechanisms for lead uptake identified in Rhus chinensis Mill. seedlings, Ecotoxicol. Environ. Saf., 2017, 142, 59–68 CrossRef CAS PubMed.
- X. Shi, Y. T. Chen, S. F. Wang and J. C. Li, Pb, Zn accumulation and nutrient uptake of 15 plant species grown in abandoned mine tailings, Environ. Sci., 2012, 33, 2021–2027 Search PubMed.
- X. Shi, S. Wang, H. Sun, Y. Chen, D. Wang, H. Pan, Y. Zou, J. Liu, L. Zheng, X. Zhao and Z. Jiang, Comparative of Quercus spp. and Salix spp. for phytoremediation of Pb/Zn mine tailings, Environ. Sci. Pollut. Res., 2017, 24, 3400–3411 CrossRef CAS PubMed.
- R. Poursattari and H. Hadi, Lead phytoremediation, distribution, and toxicity in rapeseed (Brassica napus L.): the role of single and combined use of plant growth regulators and chelators, J. Soil Sci. Plant Nutr., 2022, 1–18 Search PubMed.
- W. G. Shi, J. Zhou, J. Li, C. F. Ma, Y. H. Zhang, S. R. Deng, W. J. Yu and Z. B. Luo, Lead exposure-induced defense responses result in low lead translocation from the roots to aerial tissues of two contrasting poplar species, Environ. Pollut., 2021, 271, 116346 CrossRef CAS PubMed.
- M. Amjad, M. M. Iqbal, G. Abbas, A. B. U. Farooq, M. A. Naeem, M. Imran, B. Murtaza, M. Nadeem and S.-E. Jacobsen, Assessment of cadmium and lead tolerance potential of quinoa (Chenopodium quinoa Willd) and its implications for phytoremediation and human health, Environ. Geochem. Health, 2022, 44, 1487–1500 CrossRef CAS PubMed.
- S. Wang, X. Shi, H. Sun, Y. Chen, H. Pan, X. E. Yang and T. Rafiq, Variations in metal tolerance and accumulation in three hydroponically cultivated varieties of Salix integra treated with lead, PLoS One, 2014, 9, e108568 CrossRef PubMed.
- S. D. Bao, Routine methods for soil and agrochemistry analyses, Beijing, Agriculture Press, 3rd edn, 2000 Search PubMed.
- N. Pal and S. Sukul, Consequences of copper and lead stress on biochemical properties and mitotic chromosomal behavior of two thelypteroid ferns and their potential in tolerance of those metals, S. Afr. J. Bot., 2022, 147, 53–62 CrossRef CAS.
- T. Biswas, O. Parveen, V. P. Pandey, A. Mathur and U. N. Dwivedi, Heavy metal accumulation efficiency, growth and centelloside production in the medicinal herb Centella asiatica (L.) urban under different soil concentrations of cadmium and lead, Ind. Crops Prod., 2020, 157, 112948 CrossRef CAS.
- C. V. Cid, M. L. Pignata and J. H. Rodriguez, Effects of co-cropping on soybean growth and stress response in lead-polluted soils, Chemosphere, 2020, 246, 125833 CrossRef PubMed.
- M. Arshad, N. Naqvi, I. Gul, K. Yaqoob, M. Bilal and J. Kallerhoff, Lead phytoextraction by Pelargonium hortorum: comparative assessment of EDTA and DIPA for Pb mobility and toxicity, Sci. Total Environ., 2020, 748, 141496 CrossRef CAS PubMed.
- D. M. Qiao, Y. Han and Y. L. Zhao, Organic acids in conjunction with various oilseed sunflower cultivars promote Cd phytoextraction through regulating micro-environment in root zone, Ind. Crops Prod., 2022, 183, 114932 CrossRef CAS.
- H. C. Chen, S. L. Zhang, K. J. Wu, R. Li, X. R. He, D. N. He, C. Huang and H. Wei, The effects of exogenous organic acids on the growth, photosynthesis and cellular ultrastructure of Salix variegata Franch. under Cd stress, Ecotoxicol. Environ. Saf., 2020, 187, 109790 CrossRef CAS PubMed.
- M. A. González-Peña, J. D. Lozada-Ramírez and A. E. Ortega-Regules, Carotenoids from mamey (Pouteria sapota) and carrot (Daucus carota) increase the oxidative stress
resistance of Caenorhabditis elegans, Biochem. Biophys. Rep., 2021, 26, 100989 Search PubMed.
- S. S. Hosseini, A. Lakzian, A. Halajnia and B. S. Razavi, Optimization of EDTA and citric acid for risk assessment in the remediation of lead contaminated soil, Rhizosphere, 2020, 17, 100277 CrossRef.
- I. Khan, M. Iqbal, S. H. Raza, S. Anwar, M. Ashraf and F. Shafiq, Tartaric acid soil-amendment increases phytoextraction potential through root to shoot transfer of lead in turnip, Chemosphere, 2022, 296, 134055 CrossRef CAS PubMed.
- B. Murtaza, F. Naeem, M. Shahid, G. Abbas, N. S. Shah, M. Amjad, H. F. Bakhat, M. Imran, N. K. Niazi and G. Murtaza, A multivariate analysis of physiological and antioxidant responses and health hazards of wheat under cadmium and lead stress, Environ. Sci. Pollut. Res., 2019, 26, 362–370 CrossRef CAS PubMed.
- A. Malik, T. A. Butt, S. Naqvi, S. Yousaf and M. Iqbal, Lead tolerant endophyte Trametes hirsuta improved the growth and lead accumulation in the vegetative parts of Triticum aestivum L, Heliyon, 2020, 6, e04188 CrossRef PubMed.
- H. Ma, X. D. Li, M. Y. Wei, G. Q. Zeng, S. Y. Hou, D. Li and H. Xu, Elucidation of the mechanisms into effects of organic acids on soil fertility, cadmium speciation and ecotoxicity in contaminated soil, Chemosphere, 2020, 239, 124706 CrossRef CAS PubMed.
- J. J. Wang, Y. Y. Guo, D. L. Guo, S. L. Yin, D. L. Kong, Y. S. Liu and H. Zeng, Fine root mercury heterogeneity: metabolism of lower-order roots as an effective route for mercury removal, Environ. Sci. Technol., 2012, 46, 769–777 CrossRef CAS PubMed.
- Z. F. Xu, L. H. Chen, S. S. Tang, L. Y. Zhuang, W. Q. Yang, L. H. Tu, B. Tan and L. Zhang, Sex-specific responses to Pb stress in Populus deltoides: root architecture and Pb translocation, Trees, 2016, 30, 2019–2027 CrossRef CAS.
- H. Liu, Y. G. Liu, G. M. Zeng, J. l. Xie, B. H. Zheng, X. F. Tan, D. F. Wang, Z. C. Sun, J. Nie and Z. J. Jiang, Mitigation mechanism of Cd-contaminated soils by different levels of exogenous low-molecular-weight organic acids and Phytolacca americana, RSC Adv., 2015, 5, 45502–45509 RSC.
- M. Y. Jiang, X. Y. Cai, J. R. Liao, Y. X. Yang, Q. B. Chen, S. P. Gao, X. F. Yu, Z. H. Luo, T. Lei and B. Y. Lv, Different strategies for lead detoxification in dwarf bamboo tissues, Ecotoxicol. Environ. Saf., 2020, 193, 110329 CrossRef CAS PubMed.
- J. Zhan, Q. Zhang, T. Li, H. Yu, X. Zhang and H. Huang, Effects of NTA on Pb phytostabilization efficiency of Athyrium wardii (Hook.) grown in a Pb-contaminated soil, J. Soils Sediments, 2019, 19, 3576–3584 CrossRef CAS.
|
This journal is © The Royal Society of Chemistry 2023 |
Click here to see how this site uses Cookies. View our privacy policy here.