DOI:
10.1039/D2RA07496F
(Paper)
RSC Adv., 2023,
13, 1923-1932
An efficient approach for the green synthesis of biologically active 2,3-dihydroquinazolin-4(1H)-ones using a magnetic EDTA coated copper based nanocomposite†
Received
25th November 2022
, Accepted 28th December 2022
First published on 11th January 2023
Abstract
2,3-Dihydroquinazolinone derivatives are known for antiviral, antimicrobial, analgesic, anti-inflammatory, and anticancer activities. However, recent approaches used for their synthesis suffer from various drawbacks. Therefore, we have fabricated a highly efficient magnetic EDTA-coated catalyst, Fe3O4@EDTA/CuI via a simple approach. The ethylenediamine tetraacetic acid (EDTA) plays a crucial role by strongly trapping the catalytic sites of CuI nanoparticles on the surface of the Fe3O4 core. The designed nanocatalyst demonstrates its potential for the catalytic synthesis of 2,3-dihydroquinazolinones using 2-aminobenzamide with aldehydes as the reaction partners. The nanocatalyst was thoroughly characterized through X-ray diffraction (XRD), Fourier-transform infrared spectroscopy (FTIR), vibrating sample magnetometry (VSM), transmission electron microscopy (TEM), scanning electron microscopy (SEM), energy dispersive X-ray analysis (EDX), X-ray photoelectron spectroscopy (XPS) and inductively coupled plasma analysis (ICP). The physiochemically characterized nanocatalyst was tested for synthesis of 2,3-dihydroquinazolinones and higher yields of derivatives were obtained with less time duration. Moreover, the catalytic synthesis is easy to operate without the use of any kind of additives/bases. Furthermore, the catalyst was magnetically recoverable after the completion of the reaction and displayed reusability for six successive rounds without any loss in its catalytic efficiency (confirmed by XRD, SEM, and TEM of the recycled material) along with very low leaching of copper (2.12 ppm) and iron (0.06 ppm) ions. Also, the green metrics were found in correlation with the ideal values (such as E factor (0.10), process mass intensity (1.10), carbon efficiency (96%) and reaction mass efficiency (90.62%)).
1. Introduction
Magnetic nanoparticles have gained great interest in the last few years due to their excellent properties such as low cost, low toxicity, high surface area to bulk ratios, high activity, thermal stability, and the surface modifications capability, easy dispersion, and superparamagnetic behavior.1–3 The modification of their surfaces with different amino ligands prevent them from aggregating which leads to stabilized active metal species over the surface. Magnetic recoverable catalysts have a wide range of uses in organic transformations such as C–H activation,4 coupling reactions,5–7 reduction reactions,8 oxidation reactions,9 and the synthesis of many heterocyclic compounds.10,11 Moreover, these magnetic nanoparticles can be separated from the reaction mixture very easily using a magnet. Magnetic separable catalysts come out to be a bridge between homogenous and heterogeneous catalysts.12 Copper is a 3d transition metal whose materials have been widely used in many catalytic reactions due to its various oxidation states from Cu0 to Cu3+. Also, copper-based nanomaterials have a high boiling point of 2562 °C, which marks them appropriate for high temperature and pressure conditions.13–16
The effective approach for the synthesis of highly robust, selective and low-cost copper based nanoparticles is to create magnetic copper based nanocomposites which have been used in several organic transformation due to their easy separation with low leaching of copper.17–21 Fe3O4 and copper composites have found many application in many organic transformation such as synthesis of propargylamines using Cu(I)-pybox–Fe3O4 nanocomposites, Fe3O4@PmPDs@Cu2O as a nanocatalyst in the synthesis of 5-phenyl-[1,2,3]triazolo[1,5-c]quinazolines, Cu@DOPA@Fe3O4 as a nanocatalyst in the cross coupling of thiols and aryl halides, Fe3O4@CS-TCT-Tet-Cu(II) nanocatalyst in the synthesis of N-sulfonyl-N-aryl tetrazoles and 5-arylamino-1H-tetrazoles etc.22 The development of many heterogeneous catalytic system involving metal–organic frameworks and nanoparticles for the synthesis of various nitrogen containing heterocycles have been extensively reported.23
2,3-Dihydroquinazolinones are essential nitrogen-containing heterocyclic moieties showing various biological and pharmacological activities such as antiviral, analgesic, anti-inflammatory, anticancer and antimicrobial (Fig. 1).24–28 Numerous procedures are known for synthesizing 2,3-dihydroquinazolin-4(1H)-ones like reductive cyclization of 2-azidobenzamides or 2-nitrobenzamides,29 quinazolin-4(3H)-ones reduction,30 condensations of 2-aminobenzamides with benzil,31 desulfurizations of 2-thioxoquinazolin-4(3H)-ones.32 While, the most common method involves the condensation of ketones or aldehydes with 2-aminobenzamide in the presence of various acid catalysts like cellulose-SO3H,33 cerium(IV) ammonium nitrate (CAN),34 p-TSA,35 Y(NO3)3,36 succinimide-N-sulfonic acid,37 and ZrCl4.38 However, these methods have various limitations which includes yields in low amounts, long reaction time, use of harmful solvents, complex work-up procedure, using expensive reagents and lack of catalyst reusability. Therefore, it is essential to develop a simple, efficient, green, and sustainable route to synthesize 2,3-dihydroquinazolinones.
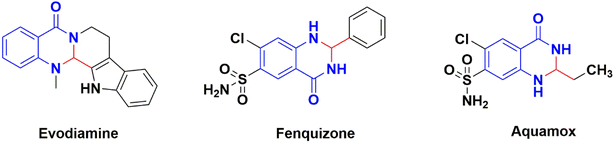 |
| Fig. 1 Biologically active molecules with 2,3-dihydroquinazolin-4(1H)-ones scaffolds. | |
Our research group has earlier successfully fabricated multiple nanocatalysts to synthesize numerous biologically active organic frameworks such as benzimidazoles, polyhydroquinolines, xanthenes, 1,4-dihydropyridines, and 4H-pyrans.39–41 In the present work, we design a novel, efficient, and magnetically recoverable Fe3O4@EDTA/CuI nanocatalyst to synthesize 2,3-dihydroquinazolin-4(1H)-ones through condensation of 2-aminobenzamide with different aldehydes. The surface of Fe3O4 has been modified with ethylenediaminetetraacetic acid (EDTA) via the wet chemical method, and then the CuI is supported over the modified surface of the magnetic nanoparticles. The techniques like FTIR, XRD, EDX, SEM, XPS, VSM, ICP and TEM were used to characterize the nanocatalyst. The fabricated material was relatively stable under the performed reaction conditions giving an excellent yield of the products, and was separated quite easily and reused for six times without much decrease in the % yield.
2. Results and discussion
2.1 Design and synthesis of the nanocatalyst
The design and synthesis of the catalyst are shown in Fig. 2. It involves the synthesis of Fe3O4 nanoparticles by co-precipitation method followed by modification of the surface with EDTA, and further CuI was immobilized on the modified support resulting in Fe3O4@EDTA/CuI.
 |
| Fig. 2 Schematic illustration of the steps involved in the fabrication of Fe3O4@EDTA/CuI. | |
Fig. 3 shows the XRD spectra of Fe3O4@EDTA/CuI nanocatalyst. The peaks appearing at a value of 2θ = 30.2, 35.8, 43.4, 53.7, 57.8 and 63.1 corresponding to (220), (311), (400), (422), (511), and (440) is in good agreement with standard patterns of Fe3O4 whereas the peaks at 2θ = 25.5, 29.4, 42.3, 50.0, 61.3, 67.4 and 77.2 corresponds to (111), (200), (220), (311), (400), (331) and (422) of copper iodide which is also in agreement with the JCPDF file (06-0246).
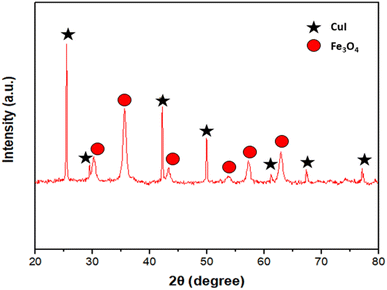 |
| Fig. 3 XRD pattern of nanocatalyst. | |
Fig. 4 displays the FT-IR spectra of the magnetic Fe3O4@EDTA/CuI nanoparticles in the range of 400–4000 cm−1. The peaks observed at 1090, 1390, 1627, 2930 and 3400–3450 cm−1 related to the aliphatic C–N stretching, strong C–C–H stretching, C
O stretch vibration, C–H stretching, O–H vibration stretching, respectively, confirms the existence of EDTA on the surface of Fe3O4.42,43
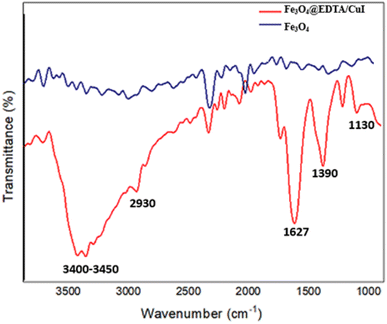 |
| Fig. 4 FTIR Spectra of the nanocatalyst. | |
Fig. 5 shows the magnetic behavior of Fe3O4 (a), Fe3O4@EDTA (b), and Fe3O4@EDTA–CuI evaluated by VSM at room temperature. The saturation magnetizations (Ms) of the magnetic Fe3O4, Fe3O4@EDTA, and Fe3O4@EDTA/CuI were found to be 85.20, 74.25 and 57.20 emu g−1 respectively. There is a decrease in Ms value when EDTA is coated over Fe3O4, and the value further decreases when CuI is immobilized over Fe3O4@EDTA. Also, the external magnet can easily separate the catalyst from the reaction mixture. Moreover, there were no coercivity, hysteresis loop, and remanence detected in any of the prepared nanomaterials, which shows the superparamagnetic nature of all.
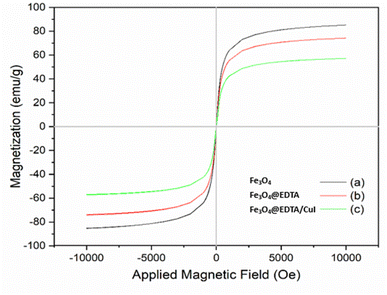 |
| Fig. 5 VSM curves of Fe3O4 (a), Fe3O4@EDTA (b) and Fe3O4@EDTA/CuI (c) nanoparticles. | |
Furthermore, CuI existence in the Fe3O4@EDTA/CuI nanocatalyst was also confirmed by X-ray photoelectron spectroscopy as shown in Fig. 6. The peak binding values at 932.3 eV and 952.2 eV were related to Cu 2p and the values at 619 eV and 632 eV were related to I 3p. The value resembles the reported data of CuI nanoparticles which also confirms the +1 oxidation state of copper.44 The values at 710.8 and 724.3 eV are allocated to the spin–orbit split doublet of Fe 2p1/2 and Fe 2p3/2 respectively which resembles with the reported values of Fe3O4.45 The broadness of the Fe 2p peaks confirms the presence of both oxidation states of iron (Fe2+ and Fe3+).46 The peak at 529.8 eV corresponds to O 1s peak of Fe3O4.
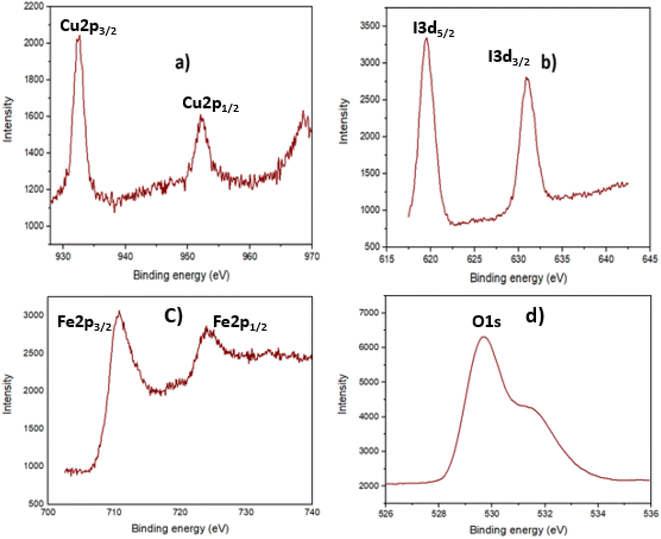 |
| Fig. 6 XPS spectrum of Fe3O4@EDTA/CuI in the region (a) Cu 2p (b) I 3d (c) Fe 2p (d) O 1 s. | |
Fig. 7 and 8 show the SEM and TEM images of Fe3O4@EDTA/CuI which clearly shows the accumulation of CuI over EDTA modified spherical Fe3O4 nanoparticles. The SEM images show Fe3O4@EDTA/CuI nanoparticles agglomerate into larger aggregates. The 20 nm, 50 nm, and 100 nm TEM images determine the synthesized material morphology and size. The images show spherical nanoparticles formation with 12–20 nm of the mean size range.
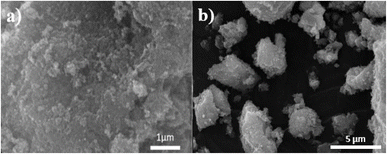 |
| Fig. 7 SEM of Fe3O4@EDTA/CuI. | |
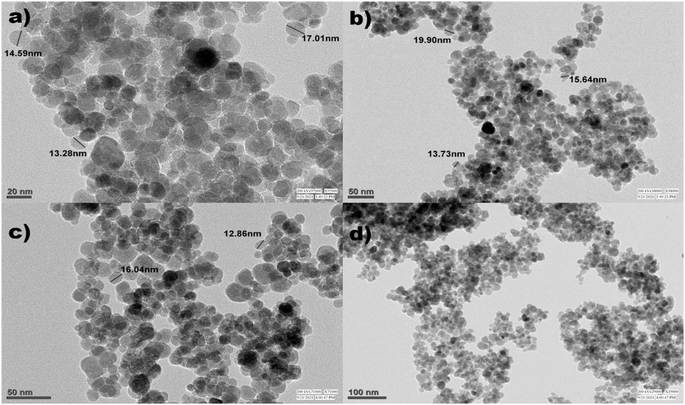 |
| Fig. 8 TEM of Fe3O4@EDTA/CuI. | |
Fig. 9 displays the catalyst EDX spectra that clearly show the presence of Cu and I over Fe3O4@EDTA surface and the percentage composition of copper, iodine, iron, oxygen and nitrogen are 12.61 19.41, 22.44, 26.04, and 19.5 wt%, respectively.
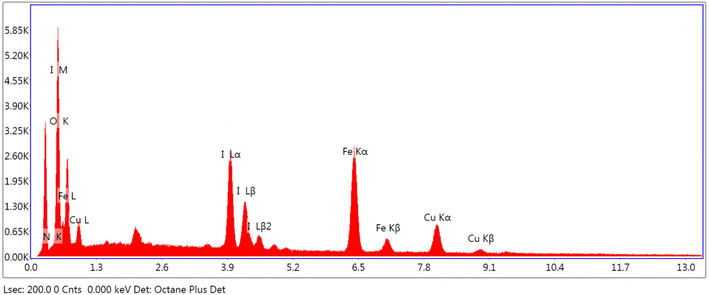 |
| Fig. 9 EDX of Fe3O4@EDTA/CuI nanocatalyst. | |
2.2 Synthesis of 2,3-dihydroquinazolin-4(1H)-ones via Fe3O4@EDTA/CuI catalyst
Primarily, the o-aminobenzamide (1), p-methylbenzaldeyde (2a) and Fe3O4@EDTA/CuI catalyst (20 mg) were used in different solvents and neat condition (Table 1). It was observed that no product was obtained when toluene was used (entry: 1, Table 1). Then solvents such as DMF, DMSO, THF and acetonitrile, which are polar aprotic, were used. It was found that the product formed was 43%, 56%, 11%, and 23% in DMF, DMSO, acetonitrile, and THF respectively (entries: 2–5, Table 1).
Table 1 Optimization study for the nanocatalytic synthesis of 2,3-dihydroquinazolin-4(1H)-ones by using o-aminobenzamide (1) and p-methylbenzaldehyde (2a) as precursors and Fe3O4@EDTA/CuI as nanocatalysta
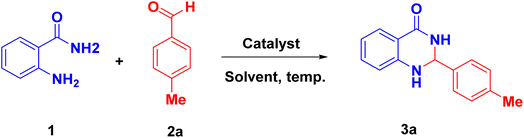
|
Entry |
Catalyst (mg) |
Solvent |
Temp (°C) |
Time (min) |
Yield (%) |
Reaction condition: o-benzamide 1 (0.5 mmol), aldehyde 2 (0.5 mmol), Fe3O4@EDTA/CuI (10–30 mg) and solvent (3 mL) were stirred at appropriate temperature. |
1 |
Fe3O4@EDTA/CuI (20) |
Toluene |
60 |
60 |
— |
2 |
Fe3O4@EDTA/CuI (20) |
DMF |
60 |
60 |
43 |
3 |
Fe3O4@EDTA/CuI (20) |
DMSO |
60 |
60 |
56 |
4 |
Fe3O4@EDTA/CuI (20) |
Acetonitrile |
60 |
60 |
23 |
5 |
Fe3O4@EDTA/CuI (20) |
THF |
60 |
60 |
11 |
6 |
Fe3O4@EDTA/CuI (20) |
Water |
60 |
60 |
— |
7 |
Fe3O4@EDTA/CuI (20) |
Neat |
60 |
60 |
— |
8 |
Fe3O4@EDTA/CuI (20) |
EG |
60 |
60 |
58 |
9 |
Fe3O4@EDTA/CuI (20) |
Ethanol |
60 |
20 min |
62 |
10 |
Fe3O4@EDTA/CuI (20) |
Methanol |
Reflux |
20 min |
78 |
11 |
Fe3O4@EDTA/CuI (20) |
Ethanol |
Reflux |
20 min |
97 |
12 |
Fe3O4@EDTA/CuI (20) |
Ethanol |
Reflux |
10 min |
76 |
13 |
Fe3O4@EDTA/CuI (20) |
Ethanol |
Reflux |
30 min |
97 |
14 |
Fe3O4@EDTA/CuI (10) |
Ethanol |
Reflux |
20 min |
81 |
15 |
Fe3O4@EDTA/CuI (30) |
Ethanol |
Reflux |
20 min |
97 |
16 |
CuI (20) |
Ethanol |
Reflux |
20 min |
56 |
When solvents like EG (ethylene glycol), water, methanol, ethanol were used, no reaction in water was observed (entry: 6, Table 1); while using ethanol and EG, the product formed was 62% and 58% respectively (entry: 8–9, Table 1). Also, no product was formed in neat reaction conditions (entry: 7, Table 1). Then the reaction was refluxed in methanol and ethanol. The yields were 78% and 97% respectively (entry: 10–11, Table 1). The better appropriate solvent which affords the product was ethanol (entry: 11, Table 1). The effect of reaction time on yield was checked, and it was found to be 76% and 97% after 10 min and 30 min (entry: 12–13, Table 1). The effect of catalyst was also investigated, which shows that on decreasing and increasing the amount of catalyst, the yield observed was 81 and 97% (entry: 14–15, Table 1). Hence, no change was observed in yield on increasing the catalyst amount. When CuI was used, the yield obtained was 56% (entry: 16, Table 1). Hence, the appropriate reaction condition for nanocatalytic synthesis was 20 mg of Fe3O4@EDTA/CuI refluxed for 20 min in ethanol.
Derivatives of 2,3-dihydroquinazolin-4(1H)-ones were synthesized using the most appropriate reaction conditions as shown in Scheme 1. The excellent yield of the products were obtained in all from the various benzaldehydes (3a–3l).
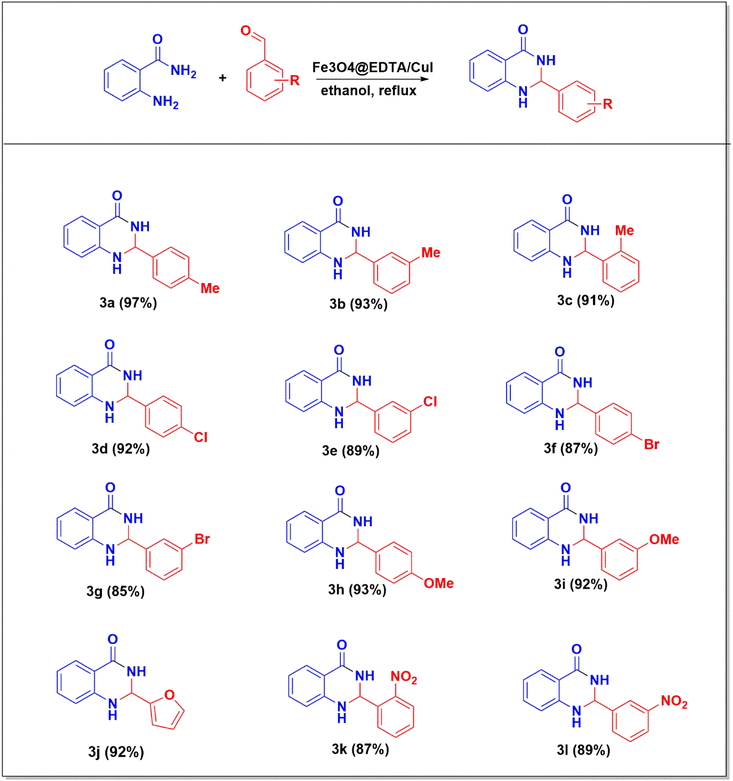 |
| Scheme 1 Fe3O4@EDTA/CuI catalyzed synthesis of 2,3-dihydroquinazolin-4(1H)-ones derivatives.a aReaction condition: o-benzamide 1 (0.5 mmol), substituted aldehyde 2 (0.5 mmol), Fe3O4@EDTA/CuI (20 mg) and ethanol (3 mL) were refluxed for 20 min. | |
Fig. 10 shows the plausible mechanism for synthesizing 2,3-dihydroquinazolin-4(1H)-ones by Fe3O4@EDTA/CuI nanocatalyst. The first step involves reaction between 2-aminobenzamide (1) and aldehyde (2) in the presence of catalyst, where catalyst behaves as Lewis acid and interacts with oxygen atom of the carbonyl to increase the electrophilicity of aldehydic carbon to give Schiff base (3) by elimination of water molecule. The next step involves the amide nitrogen attack on electrophilic carbon of the imine followed by proton transfer to obtain 2,3-dihydroquinazolin-4(1H)-ones (4).
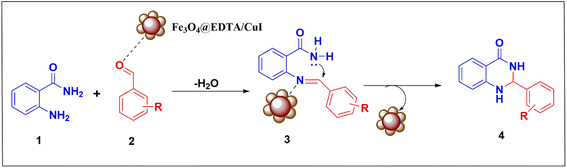 |
| Fig. 10 Plausible mechanism. | |
Then, we investigated the recyclability of the catalyst. The reaction was setup on a large scale using 5 mmol of reactants and 200 mg of catalyst. At the completion of every reaction cycle, the external magnet helped in separating the catalyst from the reaction mixture. The catalyst was washed with water and ethanol, dried and reused for subsequent reactions. The catalyst was reused six more successions and yield of the product was found to be 83% after sixth catalytic cycle as shown in Fig. 11. The stability of the material was checked after six runs by XRD, SEM and TEM which indicates that the structure and morphology of the catalyst remain unchanged (ESI Fig. S1–S3†). The ICP analysis of the filtrate was carried out after the removal of the catalyst from the reaction mixture and it was found that leached metal ion concentrations for copper and iron ion are 2.12 ppm and 0.06 ppm respectively which are lower than the authentic concentration of respective ions as per WHO terms.47
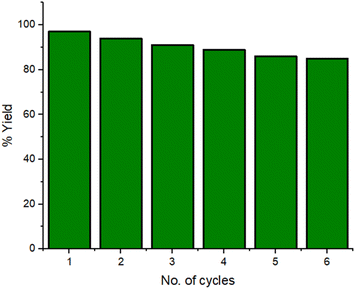 |
| Fig. 11 Recyclability of Fe3O4@EDTA/CuI nanocatalyst. | |
The calculative values of green metrics are shown in Table 2 (detail calculations in ESI†). The current method is green and sustainable as the green metrics are near ideal values. Also, the present catalyst shows better value of metrics than the previous reported methods.
Table 2 Comparison of green metrics with previously reported catalyst
S. No |
Catalyst |
E factor |
Process mass intensity |
Carbon efficiency |
Reaction mass efficiency |
1 |
Fe3O4@EDTA/CuI |
0.10 |
1.10 |
96% |
90.62% |
2 (ref. 36) |
Y(NO3)3 |
16.56 |
17.56 |
93% |
75% |
3 (ref. 47) |
Gr@SO3H |
0.16 |
1.16 |
92% |
85.54% |
4 (ref. 49) |
g-C3N4@SO3Ch |
0.31 |
1.31 |
82% |
76.17% |
Table 3 shows the comparison of Fe3O4@EDTA/CuI catalyst with various catalysts for the synthesis of 2-(4-methylphenyl)-2,3-dihydroquinazolin-4(1H)-one. It can be seen that the current catalyst has better reaction conditions when compared with other various catalysts.
Table 3 Comparison of the catalytic efficiency of the Fe3O4@EDTA/CuI with other various reported catalysts for the synthesis 2-(4-methylphenyl)-2,3-dihydroquinazolin-4(1H)-one
Entry |
Catalyst |
Solvent/condition |
Time (min) |
Yield (%) |
Ref. |
1 |
Cu(I)-modified-SBA-15 |
CH2Cl2, rt |
90 |
94% |
48 |
2 |
Gr@SO3H |
Ethanol, reflux |
40 |
92% |
49 |
3 |
[Ce(L-Pro)2]2 (oxa) |
Ethanol, 50–55 °C |
240 |
87% |
50 |
4 |
g-C3N4@SO3Ch |
PEG, rt |
140 |
82% |
51 |
5 |
Y(NO3)3·6H2O |
CH3CN, rt |
300 |
96% |
35 |
6 |
Fe3O4@EDTA/CuI |
Ethanol, reflux |
20 |
98% |
This study |
3. Experimental section
3.1 Preparation of Fe3O4, Fe3O4@EDTA, and Fe3O4@EDTA/CuI nanocomposites
The Fe3O4 nanoparticles were prepared by co-precipitation method. Briefly, 5.6 g of FeCl3·6H2O and 2.3 g of FeCl2·4H2O were dispersed in 100 mL of distilled water and stirred for 1 hour at 60 °C. Furthermore, 10 mL of ammonia (25%) solution was added dropwise with continuous stirring. The color changed instantly to black, and further, the reaction was stirred for 1 hour at 60 °C. The Fe3O4 nanoparticles were separated magnetically and washed with water and ethanol four times to remove any impurities. Lastly, the material was dried in the oven at 60 °C for 12 hours.
In 100 mL of distilled water, 1 g of synthesized Fe3O4 was dispersed with 1 g of 2Na-EDTA, and the mixture was sonicated for 30 min. The obtained Fe3O4@EDTA was isolated magnetically, washed with water followed by ethanol, and then dried in a vacuum oven at 60 °C for 12 hours.
500 mg of Fe3O4@EDTA was dispersed in 20 mL of distilled water and then 100 mg of CuI was added, and the mixture was stirred for 2 hours at room temperature. The final product was collected using a magnet and washed with distilled water and ethanol many times to eliminate impurities. The obtained catalyst Fe3O4@EDTA/CuI was dried at 60 °C for 12 hours.
3.2 General procedure for the synthesis of 2,3-dihydroquinazolin-4(1H)-ones
For the reaction, 0.5 mmol of aldehyde, 0.5 mmol of 2-aminobenzamide, 3 mL of ethanol and 20 mg of catalyst were added in a round bottom flask, and the mixture was refluxed under continuous stirring for the given time. The reaction was constantly monitored with the help of thin-layer chromatography. On completion of the reaction, the magnet was used to separate the catalyst from the reaction mixture. The product was purified using column chromatography to afford the final pure product.
4. Conclusion
In this work, we have developed a novel and efficient Fe3O4@EDTA/CuI catalyst to synthesize biologically interesting molecule 2,3-dihydroquinazolin-4(1H)-ones by reaction between 2-aminobenzamide and different aldehydes under green conditions. The method includes advantages like short reaction time, high yield, ambient reaction conditions, no additives, greener pathway, and the excellent value of green chemistry metrics. Furthermore, a magnet can easily collect the nanocatalyst and reuse it six more times with a very slight reduction in its catalytic action.
5. Spectral data
5.1 2-(4-Methylphenyl)-2,3-dihydroquinazolin-4(1H)-one (3a)
White solid; yield: 97%; mp: 230–232 °C; 1H NMR (DMSO-d6, 400 MHz): δ 8.21 (s, 1H), 7.58–7.56 (d, J = 9.21 Hz, 1H), 7.35–7.33 (d, J = 8.11 Hz, 2H), 7.22–7.14 (m, 3H), 7.03 (s, 1H), 6.72–6.61 (m, 2H), 5.67 (s, 1H), 2.25 (s, 3H). 13C NMR (DMSO-d6, 100 MHz): δ 164.19, 148.45, 139.17, 138.25, 133.79, 129.34, 127.87, 127.33, 117.60, 115.52, 114.94, 66.92, 21.26. Anal. calcd for C15H14N2O: C, 75.61; H, 5.92; N, 11.76; found C, 75.58; H, 5.94; N, 11.77.
5.2 2-(3-Methylphenyl)-2,3-dihydroquinazolin-4(1H)-one (3b)
Liquid; yield: 93%; 1H NMR (DMSO-d6, 400 MHz): δ 8.19 (s, 1H), 7.59–7.57 (d, J = 7.70 Hz, 1H), 7.28–7.12 (m, 5H), 7.03 (s, 1H), 7.35–7.33 (d, J = 8.11 Hz, 2H), 6.72–6.70 (d, J = 7.97 Hz, 1H), 6.65–6.62 (t, J = 7.83 Hz, 1H), 5.68 (s, 1H). 13C NMR (DMSO-d6, 100 MHz): δ 164.15, 148.44, 142.04, 137.95, 133.82, 129.61, 128.76, 128.03, 124.55, 117.61, 115.45, 114.91, 67.17, 21.60. Anal. calcd for C15H14N2O: C, 75.61; H, 5.92; N, 11.76; found C, 75.64; H, 5.93; N, 11.79.
5.3 2-(2-Methylphenyl)-2,3-dihydroquinazolin-4(1H)-one (3c)
Liquid; yield: 91%; 1H NMR (DMSO-d6, 400 MHz): δ 8.08 (s, 1H), 7.69–7.67 (d, J = 7.56 Hz, 1H), 7.59–7.58 (d, J = 6.60 Hz, 1H), 7.27–7.21 (m, 4H), 6.88 (s, 1H), 6.79–6.69 (s, 2H), 6.02 (s, 1H). 13C NMR (DMSO-d6, 100 MHz): δ 164.70, 149.12, 138.62, 136.68, 133.81, 131.25, 129.05, 128.02, 126.49, 117.81, 115.46, 115.07, 65.29, 19.35. Anal. calcd for C15H14N2O: C, 75.61; H, 5.92; N, 11.76; found C, 75.62; H, 5.89; N, 11.72.
5.4 2-(4-Chlorophenyl)-2,3-dihydroquinazolin-4(1H)-one (3d)
White solid; yield: 92%; mp: 197–199 °C; 1H NMR (DMSO-d6, 400 MHz): δ 8.33 (s, 1H), 7.59–7.57 (d, J = 7.56 Hz, 1H), 7.49–7.41 (m, 4H), 7.24–7.19 (m, 1H), 7.12 (s, 1H), 6.73–6.71 (d, J = 8.11 Hz, 1H), 6.66–6.63 (t, J = 7.56 Hz, 1H), 5.75 (s, 1H). 13C NMR (DMSO-d6, 100 MHz): δ 164.06, 148.20, 141.17, 133.95, 129.30, 128.85, 127.92, 117.83, 115.47, 115.01, 66.31. Anal. calcd for C14H11ClN2O: C, 65.00; H, 4.29; N, 10.83; found C, 64.98; H, 4.26; N, 10.85.
5.5 2-(3-Chlorophenyl)-2,3-dihydroquinazolin-4(1H)-one (3e)
White solid; yield: 89%; mp: 184–186 °C; 1H NMR (DMSO-d6, 400 MHz): δ 8.38 (s, 1H), 7.58–7.57 (d, J = 7.01 Hz, 1H), 7.50 (s, 1H), 7.40–7.37 (m, 3H), 7.24–7.20 (m, 2H), 6.74–6.72 (d, J = 7.97 Hz, 1H), 6.67–6.63 (t, J = 7.42 Hz, 1H), 5.75 (s, 1H). 13C NMR (DMSO-d6, 100 MHz): δ 163.98, 148.05, 144.91, 134.02, 133.51, 130.85, 128.82, 127.91, 127.29, 125.95, 117.87, 115.43, 115.02, 66.08. Anal. calcd for C14H11ClN2O: C, 65.00; H, 4.29; N, 10.83; found C, 65.01; H, 4.33; N, 10.87.
5.6 2-(4-Bromophenyl)-2,3-dihydroquinazolin-4(1H)-one (3f)
White solid; yield: 87%; mp: 199–201 °C; 1H NMR (DMSO-d6, 400 MHz): δ 8.28 (s, 1H), 7.58–7.54 (t, J = 7.56 Hz, 3H), 7.42–7.39 (d, J = 8.38 Hz, 2H), 7.23–7.19 (t, J = 7.56 Hz 1H), 7.09 (s, 1H), 6.72–6.63 (m, 2H), 5.72 (s, 1H). 13C NMR (DMSO-d6, 100 MHz): δ 164.00, 148.15, 141.65, 133.93, 131.76, 129.61, 127.89, 122.08, 117.82, 115.47, 114.99, 66.34. Anal. calcd for C14H11BrN2O: C, 55.47; H, 3.66; N, 9.24; found C, 55.48; H, 3.63; N, 9.26.
5.7 2-(3-Bromophenyl)-2,3-dihydroquinazolin-4(1H)-one (3g)
White solid; yield: 85%; mp: 199–201 °C; 1H NMR (DMSO-d6, 400 MHz): δ 8.38 (s, 1H), δ 7.64 (s, 1H), 7.58–7.57 (d, J = 7.70 Hz, 1H), 7.51–7.45 (m, 2H), 7.33–7.29 (t, J = 7.83 Hz, 1H), 7.24–7.20 (m, 2H), 6.74–6.72 (d, J = 7.97 Hz, 1H), 6.67–6.63 (t, J = 7.83 Hz, 1H), 5.75 (s, 1H). 13C NMR (DMSO-d6, 100 MHz): δ 163.98, 148.03, 145.14, 134.03, 131.71, 131.13, 130.18, 127.92, 126.32, 122.15, 117.88, 115.41, 115.02, 66.04. Anal. calcd for C14H11BrN2O: C, 55.47; H, 3.66; N, 9.24; found C, 55.44; H, 3.66; N, 9.23.
5.8 2-(4-Methoxyphenyl)-2,3-dihydroquinazolin-4(1H)-one (3h)
White solid; yield: 93%; mp: = 181–183 °C; 1H NMR (DMSO-d6, 400 MHz): δ 8.17 (s, 1H), 7.57–7.56 (d, J = 7.83 Hz, 1H), 7.38–7.36 (d, J = 8.79, 2H), 7.21–7.17 (m, 1H), 6.98 (s, 1H), 6.91–6.88 (d, J = 8.66 Hz, 2H), 6.70–6.68 (d, J = 7.83 Hz, 1H), 6.65–6.61 (m, 1H), 5.66 (s, 1H), 3.69 (s, 3H). 13C NMR (DMSO-d6, 100 MHz): δ 164.25, 159.95, 148.56, 133.95, 133.79, 128.76, 127.87, 117.623, 115.51, 114.94, 114.14, 66.83, 55.69. Anal. calcd for C15H14N2O2: C, 70.85; H, 5.55; N, 11.02; found C, 70.86; H, 5.55; N, 11.03.
5.9 2-(3-Methoxyphenyl)-2,3-dihydroquinazolin-4(1H)-one (3i)
Liquid; yield: 92%; 1H NMR (DMSO-d6, 400 MHz): δ 8.27 (s, 1H), 7.58–7.56 (d, J = 7.70 Hz, 1H), 7.28–7.19 (m, 2H), 7.09 (s, 1H), 7.03 (s, 2H), 6.88–6.86 (d, J = 8.11 Hz, 1H), 6.73–6.71 (s, J = 8.11 Hz, 1H), 6.65–6.62 (m, 1H), 5.68 (s, 1H), 3.71 (s, 3H). 13C NMR (DMSO-d6, 100 MHz): δ 164.09, 159.75, 148.34, 143.86, 133.85, 129.96, 127.87, 119.46, 117.65, 115.51, 114.94, 114.21, 113.10, 66.81, 55.61. Anal. calcd for C15H14N2O2: C, 70.85; H, 5.55; N, 11.02; found C, 70.88; H, 5.53; N, 11.01.
5.10 2-(Furan-2-yl-phenyl)-2,3-dihydroquinazolin-4(1H)-one (3j)
White solid; yield: 92%; mp: 168–170 °C; 1H NMR (DMSO-d6, 400 MHz): δ 8.38 (s, 1H), 7.58–7.56 (d, J = 7.56 Hz, 2H), 7.22–7.18 (m, 2H), 6.72–6.69 (d, J = 7.97 Hz 1H), 6.65–6.62 (t, J = 7.70 Hz 2H), 6.34–6.33 (m, 1H), 6.23–6.22 (d, J = 3.16 Hz, 1H), 5.71 (s, 1H). 13C NMR (DMSO-d6, 100 MHz): δ 163.80, 155.04, 147.66, 143.29, 133.81, 127.79, 117.75, 115.46, 114.99, 110.83, 107.67, 60.71. Anal. calcd for C12H10N2O2: C, 67.28; H, 4.71; N, 13.08; found C, 67.29; H, 4.69; N, 13.09.
5.11 2-(2-Nitrophenyl)-2,3-dihydroquinazolin-4(1H)-one (3k)
Yellow solid; yield: 87%; 1H NMR (DMSO-d6, 400 MHz): δ 8.18 (s, 1H), 7.95 (s, 1H), 7.78–7.48 (m, 2H), 7.15 (s, 2H), 6.93 (s, 2H), 6.70–6.62 (d, J = 34.90 Hz, 2H), 6.29 (s, 1H). 13C NMR (DMSO-d6, 100 MHz): δ 163.63, 147.72, 147.24, 136.07, 134.22, 133.99, 133.71, 129.93, 129.02, 127.47, 124.84, 117.83, 115.04, 114.60, 62.34. Anal. calcd for C14H11N3O3: C, 62.45; H, 4.12; N, 15.61; found C, 62.47.; H, 4.11; N, 15.64.
5.12 2-(3-Nitrophenyl)-2,3-dihydroquinazolin-4(1H)-one (3l)
Yellow solid; yield: 89%; 1H NMR (DMSO-d6, 400 MHz): δ 8.59 (s, 1H), 8.38 (s, 1H), 8.19–8.17 (d, J = 8.11 Hz, 1H), 7.96–7.94 (d, J = 7.70 Hz, 1H), 7.68–7.63 (m, 2H), 7.56–7.54 (d, J = 7.83 Hz, 1H), 7.37 (s, 1H), 7.28–7.24 (t, J = 7.28 Hz, 1H), 7.14–7.10 (t, J = 7.28 Hz, 1H), 5.97 (s, 1H). 13C NMR (DMSO-d6, 100 MHz): δ 163.58, 147.78, 147.40, 133.73, 133.44, 132.02, 130.07, 128.87, 117.68, 116.54, 115.01, 114.71, 114.52, 65.32. Anal. calcd for C14H11N3O3: C, 62.45; H, 4.12; N, 15.61; found C, 62.44.; H, 4.12; N, 15.62.
Author contributions
S. K., G. R., S. H., and R. C. designed the schemes. S. K. performed the experiments. S. K., and G. R. evaluated the data and prepared the figures and tables. G. R., S. K., S. H., and R. C. revised and reviewed the manuscript.
Conflicts of interest
The authors declare no competing financial interest.
Acknowledgements
S. K. acknowledges CSIR for the award of Junior Research Fellowship (File No. 08/0529(13252)/2022-EMR-I) and also thankful to the University of Delhi, India-110007.
References
- K. Zhu, Y. Ju, J. Xu, Z. Yang, S. Gao and Y. Hou, Acc. Chem. Res., 2018, 51, 404–413 CrossRef CAS PubMed.
- L. Shiri, H. Narimani and M. Kazemi, Appl. Organomet. Chem., 2018, 32, 3927 CrossRef.
- L. Shiri, S. Zarei, M. Kazemi and D. Sheikh, Appl. Organomet. Chem., 2017, 32, 3938 CrossRef.
- R. Sharma, S. Dutta and S. Sharma, Dalton Trans., 2015, 44, 1303–1316 RSC.
- Y. Long, K. Liang, J. Niu, X. Tong, B. Yuan and J. Ma, New J. Chem., 2015, 39, 2988–2996 RSC.
- W. Li, Y. Tian, B. Zhang, L. Tian, X. Li, H. Zhang, N. Ali and Q. Zhang, New J. Chem., 2015, 39, 2767–2777 RSC.
- J. Niu, F. Wang, X. Zhu, J. Zhao and J. Ma, RSC Adv., 2014, 4, 37761–37766 RSC.
- T. C. Nugent and M. El-Shazly, Adv. Synth. Catal., 2010, 352, 753–819 CrossRef CAS.
- L. Lykke, C. Rodríguez-Escrich and K. A. Jørgensen, J. Am. Chem. Soc., 2011, 133, 14932–14935 CrossRef CAS PubMed.
- Y.-L. Shi and M. Shi, Org. Biomol. Chem., 2007, 5, 1499–1504 RSC.
- W. Xie and J. Wang, Energy Fuels, 2014, 28, 2624–2631 CrossRef CAS.
- S. Shylesh, V. Sch€unemann and W. R. Thiel, Angew. Chem., Int. Ed., 2010, 49, 3428 CrossRef CAS PubMed.
- R. Kottappara, S. C. Pillai, B. K. Vijayan and B. K. Inorg, Chem. Commun., 2020, 121, 108181 CAS.
- M. R. Decan, S. Impellizzeri, M. L. Marin and J. C. Scaiano, Nat. Commun., 2014, 5, 1–8 Search PubMed.
- K. Yoshida, C. Gonzalez-Arellano, R. Luque and P. L. Gai, Appl. Catal., A, 2010, 379, 38–44 CrossRef CAS.
- S. Reymond and J. Cossy, Chem. Rev., 2008, 108, 535931 CrossRef PubMed.
- R. Zhang, J. Liu, S. Wang, J. Niu, C. Xia and W. Sun, ChemCatChem, 2011, 3, 146–149 CrossRef CAS.
- K. Swapna, S. N. Murthy, M. T. Jyothi and Y. V. D. Nageswar, Org. Biomol. Chem., 2011, 9, 5989–5996 RSC.
- K. Swapna, S. N. Murthy and Y. V. D. Nageswar, Eur. J. Org. Chem., 2011, 10, 1940–1946 CrossRef.
- S. Ishikawa, R. Hudson and A. Moores, Heterocycles, 2012, 86, 1023–1030 CrossRef CAS PubMed.
- B. A. Kumar, K. H. V. Reddy, B. Madhav, K. Ramesh and Y. V. D. Nageswar, Tetrahedron Lett., 2012, 53, 4595–4599 CrossRef.
-
(a) M. J. Aliaga, D. J. Ramón and M. Yus, Org. Biomol. Chem., 2010, 8, 43–46 RSC;
(b) M. Rawat, T. Taniike and D. S. Rawat, ChemCatChem, 2022, 14, e202101926 CrossRef CAS;
(c) R. N. Baig and R. S. Varma, Chem. Commun., 2012, 48, 2582–2584 RSC;
(d) M. Nasrollahzadeh, N. Motahharifar, Z. Nezafat and M. Shokouhimehr, Colloid Interface Sci. Commun., 2021, 44, 100471 CrossRef CAS.
-
(a) T. Song, P. Ren, Z. Ma, J. Xiao and Y. Yang, ACS Sustainable Chem. Eng., 2019, 8, 267–277 CrossRef;
(b) S. Yadav, A. Jain and P. Malhotra, Green Chem., 2019, 21, 937–955 RSC;
(c) M. H. D. Dang, T. T. M. Nguyen, L. H. T. Nguyen, T. T. T. Nguyen, T. B. Phan, P. H. Tran and T. L. H. Doan, New J. Chem., 2020, 44, 14529–14535 RSC;
(d) L. H. T. Nguyen, T. T. T. Nguyen, Y. T. Dang, P. H. Tran and T. Le Hoang Doan, Asian J. Org. Chem., 2019, 8, 2276–2281 CrossRef CAS;
(e) L. H. T. Nguyen, T. T. T. Nguyen, M. H. D. Dang, P. H. Tran and T. L. H. Doan, Mol. Catal., 2021, 499, 111291–111299 CrossRef CAS.
- R. Noel, N. Gupta, V. Pons, A. Goudet, M. D. Garcia-Castillo, A. Michau, J. Martinez, D. A. Buisson, L. Johannes, D. Gillet and J. Barbier, J. Med. Chem., 2013, 56, 3404–3413 CrossRef CAS PubMed.
- R. Williams, C. M. Niswender, Q. Luo, U. Le, P. J. Conn and C. W. Lindsley, Bioorg. Med. Chem. Lett., 2009, 19, 962–966 CrossRef CAS PubMed.
- U. A. Kshirsagar, Org. Biomol. Chem., 2015, 36, 9336–9352 RSC.
- D. Rambabu, S. K. Kumar, B. Y. Sreenivas, S. Sandra, A. Kandale, P. Misra, B. Rao and M. V. Manojit Pal, Tetrahedron Lett., 2013, 54, 495–501 CrossRef CAS.
- H. Li, H. Guo, Y. Su, Y. Hiraga, Z. Fang, E. J. Hensen, M. Watanabe and R. L. Smith, Nat. Commun., 2019, 10, 1–13 CrossRef PubMed.
- W. Su and B. Yang, Aust. J. Chem., 2002, 55, 695–697 CrossRef CAS.
- S. W. Li, M. G. Nair, D. M. Edwards, R. L. Kisliuk, Y. Gaumont, I. K. Dev, D. S. Duch, J. Humphreys, G. K. Smith and R. J. Ferone, Med. Chem., 1991, 34, 2746 CrossRef CAS PubMed.
- J. A. Moore, G. J. Sutherlarnod, R. Sowerbey, E. G. Kelly, S. Palermo and W. J. Webster, Org. Chem., 1969, 34, 887 CrossRef CAS.
- J. M. Khurana and G. Kukreja, J. Heterocycl. Chem., 2003, 40, 677 CrossRef CAS.
- B. S. Reddy, A. Venkateswarlu, C. Madan and A. Vinu, Tetrahedron Lett., 2011, 52, 1891–1894 CrossRef.
- M. Wang, J. J. Gao, Z. G. Song and L. Wang, Chem. Heterocycl. Compd., 2011, 47, 851–855 CrossRef CAS.
- G. Yashwantrao, V. P. Jejurkar, R. Kshatriya and S. Saha, ACS Sustainable Chem. Eng., 2019, 7, 13551–13558 CrossRef CAS.
- A. A. Khan, K. Mitra, A. Mandal, N. Baildya and M. A. Mondal, Heteroat. Chem., 2017, 28, 21379 CrossRef.
- M. Ghashang, S. S. Mansoor and K. Aswin, Res. Chem. Intermed., 2015, 41, 3447–3460 CrossRef CAS.
- M. Abdollahi-Alibeik and E. Shabani, Chin. Chem. Lett., 2011, 22, 1163–1166 CAS.
- S. Kohli, G. Rathee, S. Hooda and R. Chandra, Dalton Trans., 2020, 50, 7750–7758 RSC.
- G. Rathee, S. Kohli, N. Singh, A. Awasthi and R. Chandra, ACS Omega, 2020, 5, 15673–15680 CrossRef CAS PubMed.
- G. Rathee, S. Kohli, S. Panchal, N. Singh, A. Awasthi, S. Singh, A. Singh, S. Hooda and R. Chandra, ACS Omega, 2020, 5, 23967–23974 CrossRef CAS PubMed.
- Y. Liu, M. Chen and H. Yongmei, Chem. Eng. J., 2013, 218, 46–54 CrossRef CAS.
- R. Mostafalu, B. Kaboudin, F. Kazemi and T. Yokomatsu, RSC Adv., 2014, 4, 49273–49279 RSC.
- X. Wang, Y. Shen, A. Xie, L. Qiu, S. Li and Y. Wang, J. Mater. Chem., 2011, 21, 9641–964643 RSC.
- G. Tong, Y. Liu, T. Wu, Y. Ye and C. Tong, Nanoscale, 2015, 7, 16493–16503 RSC.
- C. Jin, Q. Zhang, W. B. Mi, E. Y. Jiang and H. L. Bai, J. Phys. D: Appl. Phys., 2010, 43, 385001 CrossRef.
- W. Yang, B. Vogler, Y. Lei and T. Wu, Environ. Sci.: Water Res. Technol., 2017, 3, 1143–1151 RSC.
- M. Hajjami, F. Ghorbani and Z. Yousofvand, Appl. Organomet. Chem., 2017, 31, 3843 CrossRef.
- S. Gajare, M. Jagadale, A. Naikwade, P. Bansode, P. Patil and G. Rashinkar, J. Heterocycl. Chem., 2020, 57, 89–102 CrossRef CAS.
- R. Katla, R. Chowrasia, C. D. da Silva, A. R. de Oliveira, B. F. dos Santos and N. L. Domingues, Synthesis, 2017, 49, 5143–5148 CrossRef CAS.
- N. Azizi and M. Edrisi, J. Mol. Liq., 2020, 300, 112263 CrossRef CAS.
Footnote |
† Electronic supplementary information (ESI) available: SEM and XRD of recycled catalyst; green chemistry metric calculations, spectral data of compounds, 1H NMR and 13C NMR spectra of all compounds. See DOI: https://doi.org/10.1039/d2ra07496f |
|
This journal is © The Royal Society of Chemistry 2023 |
Click here to see how this site uses Cookies. View our privacy policy here.