DOI:
10.1039/D2RA08176H
(Paper)
RSC Adv., 2023,
13, 6459-6465
Design, synthesis and bioactivity of myricetin derivatives for control of fungal disease and tobacco mosaic virus disease†
Received
22nd December 2022
, Accepted 18th February 2023
First published on 23rd February 2023
Abstract
A series of myricetin derivatives containing isoxazole were designed and synthesized. All the synthesized compounds were characterized by NMR and HRMS. In terms of antifungal activity, Y3 had a good inhibitory effect on Sclerotinia sclerotiorum (Ss), and the median effective concentration (EC50) value was 13.24 μg mL−1, which was better than azoxystrobin (23.04 μg mL−1) and kresoxim-methyl (46.35 μg mL−1). Release of cellular contents and cell membrane permeability experiments further revealed that Y3 causes the destruction of the cell membrane of the hyphae, which in turn plays an inhibitory role. The anti-tobacco mosaic virus (TMV) activity in vivo showed that Y18 had the best curative and protective activities, with EC50 values of 286.6 and 210.1 μg mL−1 respectively, the effect was better than ningnanmycin. Microscale thermophoresis (MST) data showed that Y18 had a strong binding affinity with tobacco mosaic virus coat protein (TMV-CP), with a dissociation constant (Kd) value of 0.855 μM, which was better than ningnanmycin (2.244 μM). Further molecular docking revealed that Y18 interacts with multiple key amino acid residues of TMV-CP, which may hinder the self-assembly of TMV particles. Overall, after the introduction of isoxazole on the structure of myricetin, its anti-Ss and anti-TMV activities have been significantly improved, which can be further studied.
1. Introduction
Plant pathogenic fungi and viral infections are highly infective and have a wide range of parasitic hosts, which seriously affect the quality and yield of crops and cause huge economic losses.1–3 At present, the use of pesticides is still one of the most effective and economical means to control and treat plant diseases.4,5 However, due to long-term use or unscientific abuse of these fungicides and antiviral agents, such as azoxystrobin, kresoxim-methyl, and ningnanmycin, plant diseases have developed drug resistance.6,7 In addition, due to the high toxicity of these traditional pesticides, they are not easy to degrade, and they also cause certain damage to the ecological environment.8,9 Therefore, it is of great interest to develop drug molecules with novel structures, environmental friendliness and highly effective bioactivity.
Myricetin is a natural dietary flavonoid that can be extracted from plants such as grapes and vine tea.10 Relevant studies have shown that myricetin can regulate a variety of physiological abnormalities, such as anticancer,11 antiviral,12–14 antioxidant,15 analgesic16 and anti-allergic.17 In addition, our group found in previous studies that myricetin and its derivatives exhibit good biological activity in plant pathogenic bacteria and viruses,9,18 which provides new ideas for the creation of new pesticides.
Isoxazole is a class of five-membered heterocyclic compounds containing nitrogen and oxygen atoms, which has attracted widespread attention with its excellent biological activity and unique structural characteristics.19 Related studies have revealed that compounds containing isoxazole structures exhibit a wide range of biological functions. It not only has good anti-cancer20 bactericidal,21 antiviral22,23 and anti-inflammatory,24 biological activities in the field of medicine, but also shows excellent antifungal,25 insecticidal,26 herbicidal27 and other biological activities in the field of pesticides.
In this study, the isoxazole was introduced into the structure of myricetin through the principle of active splicing, and a series of myricetin derivatives containing isoxazole were designed and synthesized. The design idea is shown in Fig. 1. Subsequently, the antifungal and anti-TMV activities of the synthesized target compounds were evaluated, and the mechanism of action of the compounds with excellent activity were preliminarily studied.
 |
| Fig. 1 The design ideas of target compounds. | |
2. Experimental
2.1 Instruments and chemicals
The melting point of the compounds were determined by X-4B melting point instrument (Shanghai INESA Co., Ltd.), without the correction. The target compounds Y1–Y28 were characterized by nuclear magnetic resonance spectrometer with deuterated chloroform as solvent (Bruker, Germany) and Thermo Scientific Q Exactive Mass Spectrometer (Missouri, USA). The Kd values of compounds to TMV-CP were determined by using NanoTemper Monolith NT.115 microscale thermophoresis (NanoTemper, Germany). The chromatogram of Y1 was determined by Agilent 1100 high performance liquid chromatography (HPLC) (Agilent, USA). The microstructure of Y1 was observed by Olympus CX21 optical microscope (Olympus, Japan). All solvents and reagents were analytically or chemically pure, requiring no further purification, and the aldehyde containing compounds were purchased from the Shanghai Tansoole platform. Silica gel GF254 thin-layer chromatography was used to monitor the reaction progress.
2.1.1 Detection conditions of HPLC. The chromatography was performed on Lichrospher C18 column (250 mm × 4.5 mm, 5 μm) with mobile phase consisted of water–methanol (30
:
70 vol) at the flow rate of 1.00 mL min−1. The detection wavelength was 280 nm and the sample size was 20 μL.
2.2 Synthesis
2.2.1 General procedures for preparing intermediates 1 to 4. The intermediates 1 to 4 were prepared according to the methods reported in the literature and slightly modified.28–31 Firstly, various aldehydes were used as starting materials to react with NH2OH·HCl to obtain intermediate 1; secondly, it reacted with N-chlorosuccinimide (NCS) to obtain nitrile oxide intermediate 2; then, the intermediate 3 was obtained by dipolar cycloaddition reaction with 3-bromopropyne; finally, methyl iodide was used as the methylation reagent to protect the hydroxyl group of myricitrin, and then deglycoside by concentrated hydrochloric acid to obtain intermediate 4.
2.2.2 General procedures for preparing target compounds Y1–Y28. With N,N-dimethylformamide (DMF) as solvent and anhydrous K2CO3 as acid binding agent, intermediate 3 reacted with intermediate 4 at 60 °C for 4 h, and then recrystallizes the target compounds Y1–Y28 by methanol. The specific synthesis process of the target compound Y1–Y28 can be obtained from the ESI.†
2.3 Biological activities
2.3.1 Antifungal activity in vitro. The antifungal effect of the target compounds Y1–Y28 in vitro was performed by the method reported in the literature.32 Commercial fungicides kresoxim-methyl and azoxystrobin were used as positive controls, and the experiment was repeated three times for each drug. The details are listed in the ESI.†
2.3.2 Antifungal activity in vivo. The antifungal effect of compound Y3 on Ss in vivo was studied in an artificial climate box (temperature: 20 °C, relative humidity: 80%, light: 14 h, dark: 10 h) through leaf separation experiment.33
2.3.3 Anti-TMV activity in vivo. The antiviral effect of the target compounds Y1–Y28 in vivo was evaluated by the method reported in the literature.34,35 The lead compound myricetin and commercial antiviral agent ningnanmycin were used as positive control, and the experiment was repeated three times for each drug. The details are listed in the ESI.†
2.4 Determination of release of cellular contents
Release of cellular contents test of Y3 against Ss was carried out by the method reported in the literature.33,36
2.5 Determination of cell membrane permeability
The determination experiment of Y3 on the cell membrane permeability of Ss was carried out by the method reported in the literature.33,36
2.6 Microscale thermophoresis
According to the methods reported in the literature,37 the binding affinity of Y18, myricetin and ningnanmycin to TMV-CP was determined by MST.
2.7 Molecular docking
The crystal structure of TMV-CP (PDB ID: 1EI7) was obtained from the protein database (PDB, https://www.rcsb.org).38 Discovery Studio software was used to simulate molecular docking, and minimization (QM-MM) module was used to optimize the molecular structure.39 Pymol software was used for visual analysis of molecular docking.
3. Results and discussion
3.1 Chemistry
The synthesis procedure of the target compounds Y1–Y28 is shown in Scheme 1, and their spectral data were characterized by NMR and HRMS. And the spectral data of the target compounds can be obtained from the ESI.†
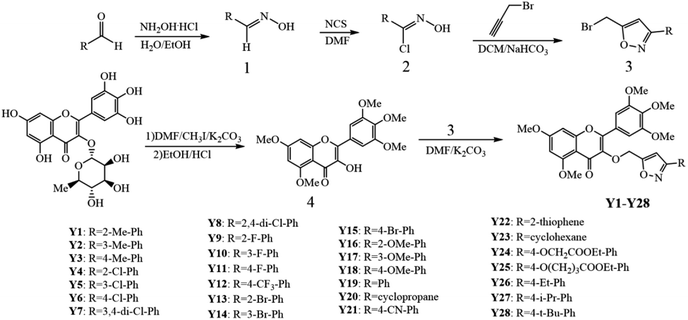 |
| Scheme 1 Synthetic route of the target compounds Y1–Y28. | |
3.2 Purity and state analysis of compound Y1
The purity of Y1 was analyzed by HPLC, as shown in Fig. S1.† The microstructure of Y1 was observed at 1500 times by optical microscope, Y1 was needle-like, and the appearance state of Y1 was observed indoors, as a white solid, as shown in Fig. S2.† These materials can be offered by ESI.†
3.3 Bioactivity and mechanism of action studies
3.3.1 Antifungal activity in vitro. As shown in Table S1†, the inhibitory effect of target compounds Y1–Y28 on 12 fungi were evaluated by in vitro antifungals, and some target compounds showed good antifungal activity. The inhibition rate of Y3 on Bc was 75.8% in vitro, which was better than that of the commercial antifungal agent kresoxim-methyl (69.3%). The inhibition rate of Y3 on Pc was 67.7% in vitro, which was better than that of kresoxim-methyl (61.1%) and azoxystrobin (63.6%). The inhibition rate of Y3 on Ss was 81.1% in vitro, which was better than that of kresoxim-methyl (62.8%) and azoxystrobin (70.3%). At this concentration, Y3 showed broad-spectrum antifungal activity.According to the preliminary biological activity test, the EC50 values of Y3 against three fungi were further tested (Table 1). Y3 had obvious inhibitory effect on Ss, EC50 value was 13.24 μg mL−1 exceeding kresoxim-methyl (46.35 μg mL−1) and azoxystrobin (23.04 μg mL−1). Y3 showed concentration dependence on Pc and Bc.
Table 1 EC50 value of target compound against 3 fungia
Pathogen |
Chemical |
Toxic regression equation |
r |
EC50 (μg mL−1) |
Repeat the experiment three times. Commercial antifungal agent kresoxim-methyl (Krm) and azoxystrobin (Azo) were used as positive control. |
Bc |
Y3 |
y = 1.1029x + 3.2374 |
0.9025 |
39.6 ± 1.8 |
Krmb |
y = 0.9655x + 3.6361 |
0.9874 |
25.9 ± 2.2 |
Pc1 |
Y3 |
y = 1.3297x + 2.7953 |
0.9759 |
45.5 ± 2.2 |
Krm |
y = 0.6592x + 4.3895 |
0.9867 |
8.4 ± 1.9 |
Azob |
y = 1.0909x + 3.5486 |
0.9729 |
21.4 ± 2.0 |
Ss |
Y3 |
y = 0.9234x + 3.9640 |
0.9867 |
13.2 ± 2.1 |
Krm |
y = 1.0383x + 3.2701 |
0.9907 |
46.4 ± 2.1 |
Azo |
y = 0.8806x + 3.8002 |
0.9849 |
23.0 ± 2.8 |
3.3.2 Antifungal activity in vivo. In vitro antifungal showed that Y3 had a good inhibitory effect on Ss, and the antifungal activity of Y3 against Ss in vivo was further evaluated. The experimental results are shown in Table 2and Fig. 2, Y3 had good protective activity in vivo against ex vivo leaves on the 4th day after inoculation, and the control effect on ex vivo leaves was 69.2% at 200 μg mL−1, which was lower than that of the commercialized fungicide azoxystrobin (83.3%).
Table 2 Protective activity of Y3 against Ss in ex vivo leaves of rapeseeda
Chemicals |
Treatment (μg mL−1) |
Lesion length (cm) |
Control efficacy (%) |
Average value of three experiments. The control azoxystrobin. |
Y3 |
200 |
1.30 ± 0.13 |
69.2 |
Azob |
200 |
0.93 ± 0.08 |
83.3 |
Blank control |
— |
3.10 ± 0.29 |
— |
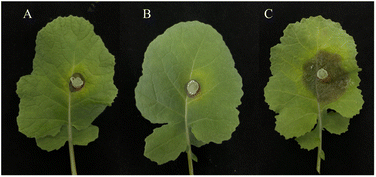 |
| Fig. 2 Anti-Ss protection efficacy of Y3 in ex vivo leaves of rapeseed. (A) Sprayed with Y3; (B) sprayed with azoxystrobin; (C) blank control. | |
3.3.3 Effects of Y3 on release of cellular contents. The changes of nucleic acid and protein concentrations in suspension of Ss mycelia were evaluated by measuring the absorbance at 260 and 280 nm. As shown in Fig. 3A and B, compared with the control group, the experimental group treated with Y3 had a significant increase in nucleic acid and protein release, and was positively correlated with the concentration of Y3.
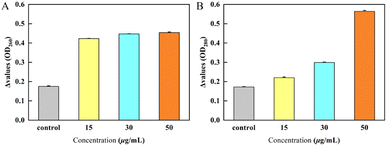 |
| Fig. 3 Release of cellular contents from Ss after treatment with Y3. ((A) leakages of nucleic acids; (B) soluble proteins). | |
3.3.4 Effect of Y3 on cell membrane permeability. Cell membranes are important for maintaining the integrity of cell structures and normal life activities. The permeability of cell membrane can be evaluated by relative conductivity. As shown in Fig. 4, the relative conductivity of mycelium in the experimental group treated with Y3 increased significantly over 7 h. Over time, the relative conductivity was much higher than in the control group. In addition, the increase in drug concentration makes the increase in conductivity more pronounced. This result shows that Y3 can cause the destruction of the hyphal cell membrane, which in turn leads to the death of the hyphae.
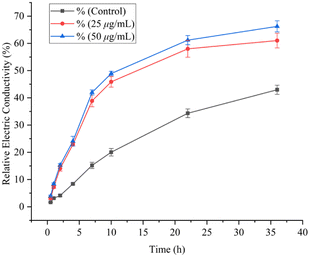 |
| Fig. 4 Determination of cell membrane permeability of Y3 anti-Ss. | |
3.3.5 Anti-TMV activity in vivo. The anti-TMV activity of the target compounds Y1–Y28 was evaluated by antiviral in vivo as shown in Table 3. The curative, protective and inactivating activities of Y1–Y28 were 29.1–60.6%, 31.9–67.7% and 34.8–62.6%, respectively. Among them, Y18 had the best curative and protective activity, 60.6 and 67.5%, respectively, which was superior to ningnanmycin (57.7 and 65.3%). The in vitro effects of Y18 and ningnanmycin on TMV are shown in Fig. 5.
Table 3 Anti-TMV activity of target compounds in vivoa
Compd |
R |
Curation |
Protection |
Inactivation |
Average value of three experiments. The control ningnanmycin. |
Y1 |
2-Me-Ph |
52.8 ± 3.2 |
40.9 ± 6.3 |
44.5 ± 1.4 |
Y2 |
3-Me-Ph |
46.2 ± 6.1 |
49.3 ± 3.3 |
46.7 ± 2.5 |
Y3 |
4-Me-Ph |
44.0 ± 6.9 |
43.8 ± 0.8 |
56.6 ± 7.6 |
Y4 |
2-Cl-Ph |
55.7 ± 3.1 |
52.2 ± 3.5 |
54.0 ± 3.0 |
Y5 |
3-Cl-Ph |
30.8 ± 6.5 |
46.0 ± 5.8 |
50.7 ± 5.6 |
Y6 |
4-Cl-Ph |
53.5 ± 1.6 |
55.8 ± 1.6 |
53.5 ± 1.4 |
Y7 |
3,4-Di-Cl-Ph |
47.1 ± 5.0 |
59.7 ± 6.6 |
41.9 ± 2.7 |
Y8 |
2,4-Di-Cl-Ph |
36.9 ± 0.6 |
56.5 ± 7.7 |
58.2 ± 5.6 |
Y9 |
2-F-Ph |
50.0 ± 6.1 |
50.3 ± 8.7 |
53.0 ± 3.2 |
Y10 |
3-F-Ph |
40.3 ± 6.8 |
52.5 ± 6.6 |
34.8 ± 6.2 |
Y11 |
4-F-Ph |
48.4 ± 1.7 |
39.8 ± 2.6 |
48.9 ± 5.3 |
Y12 |
4-CF3-Ph |
38.5 ± 5.5 |
46.3 ± 3.6 |
53.3 ± 4.1 |
Y13 |
2-Br-Ph |
33.8 ± 3.5 |
59.8 ± 5.4 |
42.2 ± 3.2 |
Y14 |
3-Br-Ph |
56.2 ± 1.6 |
65.0 ± 0.8 |
56.2 ± 5.5 |
Y15 |
4-Br-Ph |
34.3 ± 8.2 |
64.1 ± 3.8 |
41.8 ± 3.4 |
Y16 |
2-OMe-Ph |
48.6 ± 3.2 |
62.8 ± 2.4 |
62.6 ± 4.0 |
Y17 |
3-OMe-Ph |
36.0 ± 6.9 |
43.2 ± 6.0 |
56.0 ± 6.8 |
Y18 |
4-OMe-Ph |
60.6 ± 1.6 |
67.5 ± 5.5 |
60.4 ± 4.6 |
Y19 |
Ph |
33.5 ± 7.8 |
55.8 ± 8.2 |
57.2 ± 3.8 |
Y20 |
Cyclopropane |
49.5 ± 3.4 |
62.2 ± 5.7 |
53.4 ± 2.8 |
Y21 |
4-CN-Ph |
53.6 ± 2.6 |
48.0 ± 0.3 |
52.6 ± 5.4 |
Y22 |
2-Thiophene |
48.6 ± 7.8 |
41.2 ± 2.4 |
47.9 ± 5.3 |
Y23 |
Cyclohexane |
32.5 ± 5.5 |
42.5 ± 3.8 |
38.1 ± 8.3 |
Y24 |
4-OCH2COOEt-Ph |
29.1 ± 3.1 |
46.9 ± 2.5 |
45.0 ± 1.3 |
Y25 |
4-O(CH2)3COOEt-Ph |
41.5 ± 3.8 |
55.7 ± 4.8 |
39.7 ± 3.0 |
Y26 |
4-Et-Ph |
53.2 ± 4.4 |
54.0 ± 4.9 |
49.9 ± 4.3 |
Y27 |
4-i-Pr-Ph |
49.1 ± 7.3 |
31.9 ± 2.5 |
55.8 ± 3.2 |
Y28 |
4-t-Bu-Ph |
40.8 ± 6.5 |
44.1 ± 2.3 |
43.7 ± 4.8 |
Myricetin |
|
41.4 ± 3.2 |
43.6 ± 4.1 |
44.9 ± 3.6 |
NNMb |
|
55.7 ± 1.0 |
65.3 ± 2.7 |
92.4 ± 1.8 |
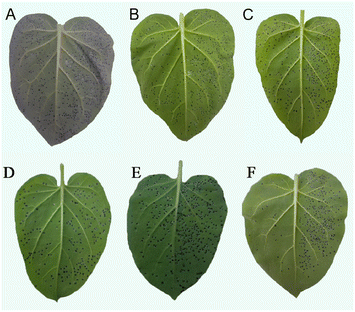 |
| Fig. 5 Morphological effects of Y18 and ningnanmycin on TMV. Y18 ((A) Curation, (B) protection, (C) inactivation); ningnanmycin ((D) curation, (E) protection, (F) inactivation)) (left lobe: drug treated; right lobe: blank control). | |
In addition, the EC50 values of Y14 and Y18 with good antiviral activity were further evaluated, as shown in Table 4. The EC50 values for the curative activity of Y14 and Y18 anti-TMV were 320.1 and 286.7 μg mL−1, respectively, superior to ningnanmycin (329.1 μg mL−1). The EC50 value of Y18 anti-TMV protective activity was 210.1 μg mL−1, which was better than ningnanmycin (230.3 μg mL−1).
Table 4 EC50 of anti-TMV with some target compounds
Activity |
Compd |
Regression equation |
r |
EC50 (μg mL−1) |
Curative activity |
Y14 |
y = 1.0549x + 2.3572 |
0.9952 |
320.1 ± 2.1 |
Y18 |
y = 1.1377x + 2.2042 |
0.9986 |
286.7 ± 3.0 |
NNM |
y = 0.9046x + 2.7228 |
0.9898 |
329.1 ± 3.2 |
Protective activity |
Y18 |
y = 1.4022x + 1.7434 |
0.9909 |
210.1 ± 4.5 |
NNM |
y = 1.2083x + 2.1456 |
0.9834 |
230.3 ± 3.7 |
3.3.6 Microscale thermophoresis. Fig. 6 shows the MST results of Y18, myricetin, ningnanmycin and TMV-CP. The binding affinity can be evaluated by Kd value. The Kd values of the three combined with TMV-CP were 0.855 ± 0.343, 60.677 ± 24.495 and 2.244 ± 0.780 μM, respectively. Y18 showed strong binding ability with TMV-CP, which was much stronger than the lead compound myricetin and the control drug ningnanmycin.
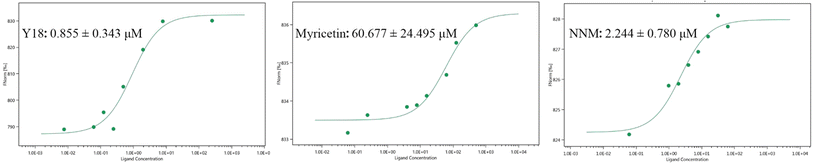 |
| Fig. 6 The MST results of Y18, myricetin and ningnanmycin to TMV-CP. | |
The results showed that the binding affinity of myricetin to TMV-CP was significantly enhanced after heterocyclic modification with isoxazole.
3.3.7 Molecular docking. Molecular docking was used to identify the identification sites of Y18, myricetin and ningnanmycin with TMV-CP. The results are shown in Fig. 7, and the three were well embedded in the active pocket of TMV-CP. Y18 had 7 conventional hydrogen bonds with TMV-CP, in which the oxygen atom on the isoxazole fragment and the oxygen atom on the methoxy group interact firmly with the amino acid residues ASN73 and LYS268 through two conventional hydrogen bonds (1.96 Å, 1.66 Å), respectively. There were 3 conventional hydrogen bonds and 6 conventional hydrogen bonds in myricetin and ningnanmycin and TMV-CP, respectively, and the number of hydrogen bonds was lower than that of Y18. In addition, Y18 binds well to amino acid residues ALA74, TYR139, GLU131 and VAL75 by conventional hydrogen bonding, pi-lone pair, carbon–hydrogen bonding, and pi-alkyl, respectively. Previous studies have reported that such amino acid residues play a key role in the self-assembly of TMV particles,40,41 and Y18 may hinder the self-assembly of TMV particles. The molecular docking results were consistent with the bioassay activity. Based on the molecular docking results of Y18, myricetin and ningnanmycin well verified the good antiviral activity of Y18.
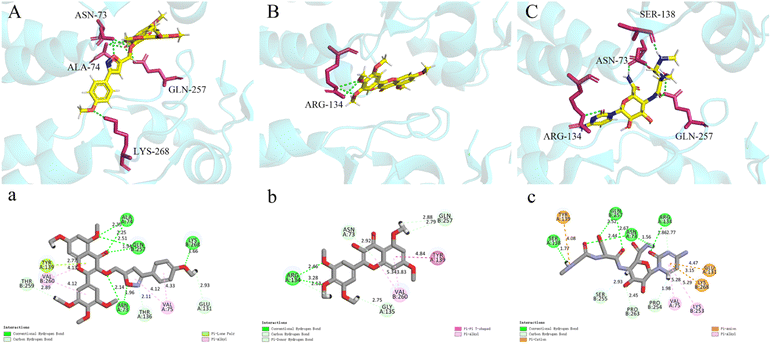 |
| Fig. 7 Molecular docking of Y18, myricetin, and ningnanmycin to TMV-CP. Y18 (A, a), myricetin (B, b), ningnanmycin (C, c). | |
4. Conclusion
A series of myricetin derivatives containing isoxazole were designed and synthesized, and the activity of all synthetic compounds against 12 plant-derived fungi and anti-TMV was evaluated. The antifungal activity showed that the EC50 value of Y3 for Ss was 13.24 μg mL−1 was superior to azoxystrobin and kresoxim-methyl. Release of cellular contents and cell membrane permeability experiments further revealed that Y3 causes destruction of the cell membrane of hyphae. The results of in vivo antiviral activity test showed that Y18 had the best curative and protective activity, and the EC50 values were 286.6 and 210.1 μg mL−1, respectively, which were better than ningnanmycin (329.1 and 230.3 μg mL−1). The MST results showed that Y18 had a strong binding affinity with TMV-CP. The molecular docking results further revealed that Y18 may hinder the self-assembly of TMV particles. In summary, myricetin derivatives containing isoxazole backbones can serve as potential antifungal, antiviral drug molecules.
Conflicts of interest
The authors declare no competing financial interest.
Acknowledgements
This research was completed with the support of the National Natural Science Foundation of China (No. 21867003), the Science Foundation of Guizhou Province (No. 20192452), Key Laboratory of Institute of Environment and Plant Protection (No. HZSKFKT202208).
References
- M. V. Nguyen, J. W. Han, H. Kim and G. J. Choi, ACS Omega, 2022, 7, 33273–33279 CrossRef CAS PubMed.
- Z. W. Wang, Q. Peng, X. Gao, S. Zhong, Y. Fang, X. L. Yang, Y. Ling and X. Liu, J. Agric. Food Chem., 2020, 68, 5318–5326 CrossRef CAS PubMed.
- R. N. Silva, V. N. Monteiro, A. S. Steindorff, E. V. Gomes, E. F. Noronha and C. J. Ulhoa, Fungal Biol., 2019, 123, 565–583 CrossRef PubMed.
- P. Y. Wang, M. W. Wang, D. Zeng, M. Xiang, J. R. Rao, Q. Q. Liu, L. W. Liu, Z. B. Wu, Z. Li, B. A. Song and S. Yang, J. Agric. Food Chem., 2019, 67, 3535–3545 CrossRef CAS PubMed.
- X. Zhou, Y. Q. Ye, S. S. Liu, W. B. Shao, L. W. Liu, S. Yang and Z. B. Wu, Pestic. Biochem. Physiol., 2021, 172, 104749 CrossRef CAS PubMed.
- C. Buttimer, O. McAuliffe, R. P. Ross, C. Hill, J. O'Mahony and A. Coffey, Front. Microbiol., 2017, 8, 34 Search PubMed.
- S. K. Wu, J. Shi, J. X. Chen, D. Y. Hu, L. S Zang and B. A. Song, J. Agric. Food Chem., 2021, 69, 4645–4654 CrossRef CAS PubMed.
- X. Tang, C. Zhang, M. Chen, Y. N. Xue, T. T. Liu and W. Xue, New J. Chem., 2020, 44, 2374–2379 RSC.
- T. T. Liu, F. Peng, X. Cao, F. Liu, Q. F. Wang, L. W. Liu and W. Xue, ACS Omega, 2021, 6, 30826–30833 CrossRef CAS PubMed.
- Z. Javed, K. Khan, J. Herrera-Bravo, S. Naeem, M. J. Iqbal, Q. Raza, H. Sadia, S. Raza, M. Bhinder, D. Calina, J. Sharifi-Rad and W. C. Cho, Cancer Cell Int., 2022, 22, 239 CrossRef CAS PubMed.
- S. C. Iyer, A. Gopal and D. Halagowder, Mol. Cell. Biochem., 2015, 407, 223–237 CrossRef CAS PubMed.
- S. Pasetto, V. Pardi and R. M. Murata, PLoS One, 2014, 9, e115323 CrossRef PubMed.
- M. S. Yu, J. Lee, J. M. Lee, Y. Kim, Y. W. Chin, J. G. Jee, Y. S. Keum and Y. J. Jeong, Bioorg. Med. Chem. Lett., 2012, 22, 4049–4054 CrossRef CAS PubMed.
- X. W. Su and D. H. D'Souza, Food Environ. Virol., 2013, 5, 97–102 CrossRef CAS PubMed.
- R. Bertin, Z. Chen, R. Marin, M. Donati, A. Feltrinelli, M. Montopoli, S. Zambon, E. Manzato and G. Froldi, Biomed. Pharmacother., 2016, 82, 472–478 CrossRef CAS PubMed.
- Y. Tong, X. M. Zhou, S. J. Wang, Y. Yang and Y. L. Cao, Arch. Pharmacal Res., 2009, 32, 527–533 CrossRef CAS PubMed.
- K. C. Medeiros, C. A. Figueiredo, T. B. Figueredo, K. R. Freire, F. A. Santos, N. M. Alcantara-Neves, T. M. Silva and M. R. Piuvezam, J. Ethnopharmacol., 2008, 119, 41–46 CrossRef CAS PubMed.
- S. C. Jiang, S. J. Su, M. Chen, F. Peng, Q. Zhou, T. T. Liu, L. W. Liu and W. Xue, J. Agric. Food Chem., 2020, 68, 5641–5647 CrossRef CAS PubMed.
- V. Basavanna, S. Doddamani, M. Chandramouli, U. K. Bhadraiah and S. Ningaiah, J. Iran. Chem. Soc., 2022, 19, 3249–3283 CrossRef CAS.
- G. C. Arya, K. Kaur and V. Jaitak, Eur. J. Med. Chem., 2021, 221, 113511 CrossRef CAS PubMed.
- M. B. Bommagani, J. R. Yerrabelly, M. Chitneni, G. Thalari, N. R. Vadiyala, S. K. Boda and P. R. Chitneni, Chem. Data Collect., 2021, 31, 100629 CrossRef CAS.
- A. Egorova, E. Kazakova, B. Jahn, S. Ekins, V. Makarov and M. Schmidtke, Eur. J. Med. Chem., 2020, 188, 112007 CrossRef CAS PubMed.
- P. Kumar Kushwaha, K. Saurabh Srivastava, N. Kumari, R. Kumar, D. Mitra and A. Sharon, Bioorg. Med. Chem., 2022, 56, 116612 CrossRef CAS PubMed.
- G. Saravanan, V. Alagarsamy and P. Dineshkumar, Arch. Pharmacal Res., 2021, 44, 1–11 CrossRef PubMed.
- F. Liu, M. Y. Wang, X. H. Teng, P. Z. Zhang and L. Jiang, Res. Chem. Intermed., 2013, 40, 1575–1581 CrossRef.
- R. F. Sun, Y. Q. Li, L. S. Xiong, Y. X. Liu and Q. M. Wang, J. Agric. Food Chem., 2011, 59, 4851–4859 CrossRef CAS PubMed.
- X. L. Sun, Z. M. Ji, S. P. Wei and Z. Q. Ji, J. Agric. Food Chem., 2020, 68, 15107–15114 CrossRef CAS PubMed.
- L. Schwarz, U. Girreser and B. Clement, Eur. J. Org. Chem., 2014, 2014, 1961–1975 CrossRef CAS.
- H. Huang, P. Si, L. Wang, Y. Xu, X. Xu, J. Zhu, H. L. Jiang, W. H. Li, L. L. Chen and J. Li, ChemMedChem, 2015, 10, 1184–1199 CrossRef CAS PubMed.
- A. V. Serebryannikova, E. E. Galenko, M. S. Novikov and A. F. Khlebnikov, Tetrahedron, 2021, 88, 132153 CrossRef CAS.
- W. Xue, B. A. Song, H. J. Zhao, X. B. Qi, Y. J. Huang and X. H. Liu, Eur. J. Med. Chem., 2015, 97, 155–163 CrossRef CAS PubMed.
- X. M. Tang, Q. Zhou, W. L. Zhan, D. Hu, R. Zhou, N. Sun, S. Chen, W. N. Wu and W. Xue, RSC Adv., 2022, 12, 2399–2407 RSC.
- X. D. Yin, K. Y. Ma, Y. L. Wang, Y. Sun, X. F. Shang, Z. M. Zhao, R. X. Wang, Y. J. Chen, J. K. Zhu and Y. Q. Liu, J. Agric. Food Chem., 2020, 68, 11096–11104 CrossRef CAS PubMed.
- T. T. Liu, F. Peng, Y. Y. Zhu, X. Cao, Q. F. Wang, F. Liu, L. W. Liu and W. Xue, Arabian J. Chem., 2022, 15, 104019 CrossRef CAS.
- X. H. Gan, D. Y. Hu, Y. J Wang, L. Yu and B. A. Song, J. Agric. Food Chem., 2017, 65, 4367–4377 CrossRef CAS PubMed.
- D. Y. Ma, D. C. Ji, Z. Q. Zhang, B. Q. Li, G. Z. Qin, Y. Xu, T. Chen and S. P. Tian, Postharvest Biol. Technol., 2019, 150, 158–165 CrossRef CAS.
- T. Guo, R. J Xia, M. Chen, J. He, S. J. Su, L. W. Liu, X. Y. Li and W. Xue, RSC Adv., 2019, 9, 24942–24950 RSC.
- Y. Chen, P. Li, S. J. Su, M. Chen, J. He, L. W. Liu, M. He, H. Wang and W. Xue, RSC Adv., 2019, 9, 23045–23052 RSC.
- D. X. Luo, S. X. Guo, F. He, S. H. Chen, A. L. Dai, R. F. Zhang and J. Wu, J. Agric. Food Chem., 2020, 68, 7226–7234 CrossRef CAS PubMed.
- A. C. Bloomer, J. N. Champness, G. Bricogne, R. Staden and A. Klug, Nature, 1978, 276, 362–368 CrossRef CAS PubMed.
- Y. Y. Wang, F. Z. Xu, Y. Y. Zhu, B. A. Song, D. X. Luo, G. Yu, S. H. Chen, W. Xue and J. Wu, Bioorg. Med. Chem. Lett., 2018, 28, 2979–2984 CrossRef CAS PubMed.
|
This journal is © The Royal Society of Chemistry 2023 |
Click here to see how this site uses Cookies. View our privacy policy here.