DOI:
10.1039/D3RA01454A
(Review Article)
RSC Adv., 2023,
13, 12244-12269
Advances in organic photovoltaic cells: a comprehensive review of materials, technologies, and performance
Received
4th March 2023
, Accepted 26th March 2023
First published on 19th April 2023
Abstract
This paper provides a comprehensive overview of organic photovoltaic (OPV) cells, including their materials, technologies, and performance. In this context, the historical evolution of PV cell technology is explored, and the classification of PV production technologies is presented, along with a comparative analysis of first, second, and third-generation solar cells. A classification and comparison of PV cells based on materials used is also provided. The working principles and device structures of OPV cells are examined, and a brief comparison between device structures is made, highlighting their advantages, disadvantages, and key features. The various parts of OPV cells are discussed, and their performance, efficiency, and electrical characteristics are reviewed. A detailed SWOT analysis is conducted, identifying promising strengths and opportunities, as well as challenges and threats to the technology. The paper indicates that OPV cells have the potential to revolutionize the solar energy industry due to their low production costs, and ability to produce thin, flexible solar cells. However, challenges such as lower efficiency, durability, and technological limitations still exist. Despite these challenges, the tunability and versatility of organic materials offer promise for future success. The paper concludes by suggesting that future research should focus on addressing the identified challenges and developing new materials and technologies that can further improve the performance and efficiency of OPV cells.
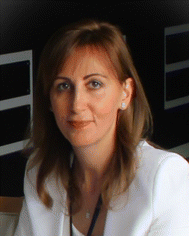 Ebru Kondolot Solak | Ebru Kondolot Solak graduated with a BSc degree from the Department of Chemistry from Erciyes University in Kayseri, Turkey in 1998. She obtained her MSc degree in Physical Chemistry from the same University in 2000 and PhD in Polymer Science from Gazi University, Turkey in 2005. Her research interests include polymer science, biopolymers, synthesis of graft copolymers, membrane processes, separation of volatile organic/water mixtures, and controlled release systems. She has authored or co-authored more than 100 peer-reviewed publications. Currently, she is a full-time Professor in the Department of Chemistry and Chemical Processing Technologies at Gazi University. |
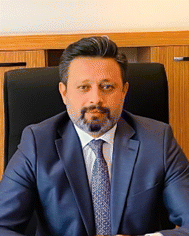 Erdal Irmak | Erdal Irmak received his BSc degree from the Department of Electrical Education of Gazi University in Ankara, Turkey in 1997. He obtained his MSc and PhD degrees from the Department of Electrical Education at the Institute of Science and Technology of Gazi University in 2001 and 2007, respectively. His main research areas include power system operation and control, voltage and frequency stability, and renewable energy systems. He has authored or co-authored more than 100 peer-reviewed publications. Currently, he is a full-time Professor in the Department of Electrical and Electronics Engineering at Gazi University. |
1. Introduction and motivation
The importance of renewable energy sources has become increasingly apparent in recent years as concerns about climate change, energy security, and the negative impacts of non-renewable sources continue to grow. Renewable energy offers a more sustainable and environmentally friendly alternative to non-renewable sources such as fossil fuels, and can help to reduce our dependence on foreign oil, increase energy security, and address the climate crisis.1,2
One of the main reasons for the importance of renewable energy is its potential to address climate change.3,4 Greenhouse gas emissions from the burning of fossil fuels are a major contributor to global warming and climate change, and the use of renewable energy can help to reduce these emissions. In addition to reducing emissions, renewable energy can also help to reduce air pollution, which has a number of negative health impacts. Another important reason for the need for renewable energy is energy security.5 By relying on domestic sources of energy, individuals and society can reduce their vulnerability to disruptions in the global energy market and ensure a stable and reliable supply of energy for the future. Renewable energy sources such as solar and wind are also less prone to supply disruptions than non-renewable sources, as they do not rely on finite resources that can be disrupted by geopolitical tensions or natural disasters.6,7
Despite the many benefits of renewable energy, there are also challenges associated with its deployment. One of the main challenges is the upfront cost of developing and installing renewable energy technologies, which can be higher than the cost of non-renewable sources in the short term.8 However, the long-term costs of renewable energy are often lower due to the fact that they do not require the constant purchase of fuel, and their costs are also likely to decrease as technology improves and economies of scale are achieved.9,10
Among renewable energy sources, solar energy is perhaps the most well-known. It is generated using photovoltaic panels, which convert sunlight into electricity. Solar energy is a clean, renewable source of energy that is widely available and can be used in a variety of applications, including electricity generation, heating, and lighting.11 One of the main benefits of solar energy is that it is relatively easy to install and maintain, and it can be used in a variety of locations, including urban, suburban, and rural areas.12
Solar cells, also known as photovoltaic cells, are a type of renewable energy source that converts sunlight into electricity through a process called the photovoltaic effect.13,14 They are made up of a semiconductor material that absorbs sunlight and releases electrons, which can be captured and used to generate electricity. There are several types of solar cells, including traditional inorganic cells made of silicon and newer organic cells made of polymers or small molecules.
Crystalline silicon cells are the most common type of solar cell and are made from a single crystal or polycrystalline silicon. They are efficient and durable, but can be expensive to produce. Organic solar cells, on the other hand, are made by depositing a thin layer of photovoltaic material onto a substrate, such as glass or polymeric material. They can also be made into a variety of shapes and sizes, making them more versatile. However, organic solar cells currently have lower efficiency rates and shorter lifetimes compared to traditional inorganic cells. Despite these limitations, research and development in the field of organic solar cells is ongoing, and there is potential for these materials to play a significant role in the future of solar energy.
As a result, there has been a growing interest in PV cell technology, which has the potential to provide clean, sustainable energy. In this context, this review paper aims to provide a comprehensive study of the evolution of PV cell technology, with a particular focus on OPV cells. The review makes several significant contributions to the existing literature on OPV cell, some of them are as follows:
• Providing a comprehensive overview of the evolution of photovoltaic cell technology and its historical context, including the classification of PV production technologies, comparison of PV cells based on the materials used, and a comparative analysis of first, second, and third-generation solar cells. This in-depth analysis provides valuable insight into the development of PV cells and the factors that have led to the emergence of OPV cells.
• Exploring the working principles and device structures of OPV cells, including a comparison of device structures. This provides a detailed analysis of the advantages and disadvantages of OPV cells, as well as their key features.
• Conducting a detailed SWOT analysis for OPV cells, revealing the strengths, weaknesses, opportunities, and threats associated with the technology. This analysis provides a comprehensive overview of the current state of the technology and the challenges and opportunities it faces.
• Analyzing the performance, efficiency, and electrical characteristics of photovoltaic cells, as well as the conversion efficiencies of OPV cells. By comparing the performance characteristics reported in recent literature on organic solar cells, the review highlights the latest trends and advancements in the field of OPV cells.
• Identifying several areas for future research and development, including improving efficiency and stability, developing new materials, and optimizing morphological characteristics for charge transport. These recommendations provide a valuable roadmap for future research and development in the field of OPV cells.
Overall, this review paper offers a detailed analysis and comprehensive perspective on the current state and future prospects of photovoltaic cell technology, with a specific focus on OPV cells. It is a valuable resource for researchers, engineers, and policymakers interested in the development of sustainable and efficient solar energy technologies.
2. PV generation technologies
Energy crises that have occurred at different times in the world have led to the search for renewable alternative energy sources and research in this direction has mainly focused on obtaining electrical energy from solar and wind energy.15 Photovoltaics have been a subject of intense interest during this period and significant resources have been allocated to research studies.16,17 Intensive efforts have been made to produce photovoltaic devices at lower costs and to increase their efficiency.
2.1. Historical overview of the evolution of PV cell technology
The history of PV cells can be traced back to the late 19th century, when the French physicist Alexandre-Edmond Becquerel discovered the phenomenon of the photovoltaic effect.18,19 He observed that certain materials, when exposed to light, produced a small electrical current. This was the first step in the development of PV technology.
In the early 20th century, researchers such as Albert Einstein and Charles Fritts continued to study the photovoltaic effect and improve upon the efficiency of PV cells. Fritts, for example, created the first working PV cell by layering selenium and gold onto glass, which had an efficiency of only 1%. In the 1950s and 60s, the space race between the United States and the Soviet Union led to significant advancements in PV technology.20,21 The US government invested heavily in the development of PV cells for use in space satellites. This led to the creation of more efficient and durable PV cells, with efficiencies reaching around 14%.22
In the 1970s, the oil crisis led to increased interest in renewable energy sources, including PV technology. This resulted in the development of new materials and manufacturing techniques that further improved the efficiency and cost-effectiveness of PV cells.23 In the 1980s and 90s, silicon-based PV cells became the dominant technology and were widely used in a variety of applications. This period also saw the development of thin-film PV cells, which used less silicon and were more cost-effective to produce.
In the 21st century, PV technology has continued to evolve and improve. The efficiency of PV cells has reached over 25%, and new materials such as perovskite and quantum dots have been developed, which have the potential to further increase efficiency. The perovskite material was found to have high light absorption, high charge-carrier mobility, and a suitable band gap for solar energy conversion.24,25 Since then, perovskite solar cells have attracted a lot of attention from the scientific community due to their high efficiency potential and low cost. The efficiency of perovskite solar cells has increased significantly over the years, with the current record efficiency at over 25%.
In parallel to the initial studies of PV cell technologies, the history of OPV cells can be traced back to the early 20th century when scientists first started to explore the potential of organic materials as a substitute for traditional inorganic materials in solar cells. The researchers in ref. 26 demonstrated that the polymer could be used as a photoconductive material, generating electrical power when exposed to light. This was a significant development in organic solar cell technology and led to the creation of new materials and device architectures. Since then, research in the field of organic solar cells has continued, resulting in the development of more efficient and stable organic solar cell technologies. However, it was only in the latter part of the 20th century that substantial progress was made in the advancement of OPV technology. In the 1970s and 80s, researchers delved into the utilization of polymers as active layers in solar cells. These early attempts yielded low efficiencies, usually below 1%. Nevertheless, they established the foundation for the creation of more sophisticated OPV cells in the following decades.
During the 1990s, the discovery of new conjugated polymers and small-molecule materials resulted in a marked improvement in the efficiency of OPV cells. Scientists were able to attain efficiencies of up to 4% using these new materials.27,28 Additionally, the creation of new fabrication techniques, such as solution processing, made it possible to produce OPV cells at a lower cost. In the early 21st century, the efficiency of OPV cells continued to advance, reaching around 18%.29 This was partly due to the development of new materials and device architectures, as well as advancements in fabrication techniques. Moreover, researchers started to examine the use of multiple layers in OPV cells, which led to further increases in efficiency.
In recent years, scientists have made notable progress in the development of OPV cells with improved efficiency, stability and cost-effectiveness. The efficiency of OPV cells has reached up to 20% which is relatively higher than the early stage.30,31 The development of new materials such as perovskite, fullerene derivatives, and new device architectures such as tandem cells have contributed to this improvement. Furthermore, advancements in manufacturing techniques have made OPV cells more affordable and accessible to a wider range of consumers. Briefly, the historical development of OPV cells has been marked by consistent progress in efficiency, stability, and cost-effectiveness. While OPV cells are still less efficient than traditional inorganic solar cells, they offer several advantages such as the potential for low-cost, large-scale production, and the ability to be flexible and transparent. As research continues to enhance the performance of OPV cells, it is likely that they will become an increasingly important part of the renewable energy mix in the future.
2.2. Generations of PV production technologies
As mentioned within the previous section, PV production technologies have evolved over time, and different types of technologies are available in the market. Therefore, understanding the classification of PV production technologies is important for policymakers, investors, and consumers to make informed decisions about the implementation of solar power systems. A general classification of PV production technologies is shown in Fig. 1.32,33
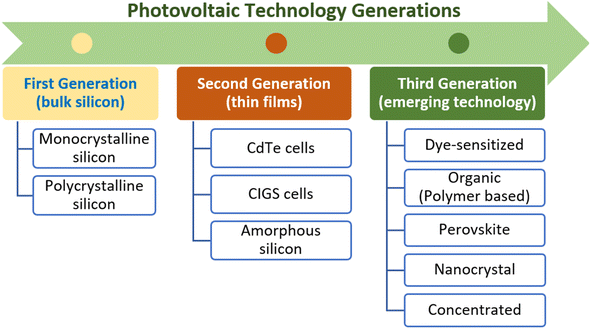 |
| Fig. 1 Three generations of PV cells. | |
First generation solar cells, also known as conventional or traditional solar cells, are made primarily of silicon.34 These cells were first developed in the 1950s and have been the most widely used type of solar cell to date.35,36 The efficiency of these cells ranges from 6–15%, but through continuous research and development, the efficiency of these cells has increased significantly over the years and now reaches levels of up to 25% as illustrated in Fig. 2.37
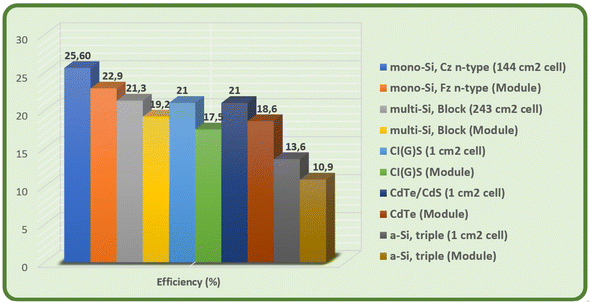 |
| Fig. 2 Efficiency of silicon-based technology in laboratories.37 | |
First generation solar cells have some limitations, such as a relatively low efficiency and a high cost of raw materials. Their efficiency drops significantly in high temperatures, which can cause power loss. Recent research has been focused on developing new materials and technologies to improve the efficiency and to reduce the cost of production.
Second generation solar cells, also known as thin-film solar cells, are made from materials like copper indium gallium selenide (CIGS), cadmium telluride (CdTe) and amorphous silicon (a-Si).37,38 They are thinner than traditional solar cells and have a higher tolerance to temperature changes, with an efficiency range of 10–15%. They use less material, are more flexible, lightweight, and can be manufactured using a roll-to-roll process which makes it more cost-effective. These cells are good for building-integrated photovoltaics (BIPV) and portable and lightweight solar panels for outdoor activities.39 They have lower efficiency and performance can degrade over time and their long-term stability and durability are not yet well understood. They are becoming more popular due to cost-effectiveness and versatility but research and development is ongoing to improve their efficiency, lifetime, and cost-effectiveness to make them more competitive with traditional solar cells.40
Third generation solar cells, some of which are highlighted in ref. 41–49, are important because they utilize materials that are cheaper than those used in first- and second-generation solar cells. These materials, such as perovskites, notable examples of which can be found in ref. 50–55, are abundant and can be processed using low-cost manufacturing techniques. As a result, third generation solar cells have the potential to significantly reduce the cost of solar energy,42 making it more accessible to people around the world.43 Additionally, third generation solar cells are capable of achieving higher efficiencies than previous generations, meaning that they can generate more electricity from the same amount of sunlight.44,56 This makes them an attractive option for both large-scale and small-scale solar energy applications.
2.3. Comparative analysis of first, second, and third generation solar cells
While the first-generation solar cells are a proven technology, the second-generation solar cells offer more flexibility and cost-effectiveness. The third-generation solar cells are the newest and most promising technology. Table 1 presents a detailed comparison table between the first, the second, and the third-generation solar cells. As shown, first generation cells use silicon and have an efficiency range of 6–25%. They are a proven technology but have some limitations such as a high cost of raw materials and performance drop in high temperatures. Second generation cells are made from materials like CIGS, CdTe, and a-Si and have an efficiency range of 10–15%. They are flexible, lightweight, cost-effective, and have roll-to-roll manufacturing. However, their lower efficiency, long-term stability, and durability are not yet well understood. Third generation cells are a newer technology that use materials like perovskites and have an efficiency range of over 25%. They have the potential to significantly reduce the cost of solar energy and can generate more electricity from the same amount of sunlight.
Table 1 Comparison between the first, the second, and the third-generation solar cells
|
First generation |
Second generation |
Third generation |
Materials |
Silicon |
CIGS, CdTe, a-Si |
Multi-junction cells, organic materials, perovskite materials, flexible substrates |
Efficiency range |
6–25% |
10–15% |
>25% |
Advantages |
Proven technology, increasing efficiency |
Flexible, lightweight, roll-to-roll production, cost-effective |
Cheaper materials, potential to significantly reduce the cost of solar energy, higher efficiencies |
Limitations |
High raw material cost, performance drops in high temperatures |
Lower efficiency, long-term stability and durability, not yet well understood |
Still in the research and development phase |
Manufacturing process |
Wafer-based |
Roll-to-roll |
Various, depending on material and design |
Applications |
Residential, commercial, utility-scale projects |
Building-integrated photovoltaics, portable and lightweight solar panels, small-scale projects |
Large-scale projects, consumer electronics, off-grid applications |
Durability |
Good |
Moderate |
Varies, depending on material and design |
Stability |
Good |
Moderate |
Varies, depending on material and design |
3. Advances in material preparation for PV cells
PV cells are made from various materials and technologies, which result in different types of photovoltaic cells. A general classification of them can be made as in the following section.
3.1. Classification and comparison of PV cells based on materials used
While other types or variations exist, photovoltaic cells can generally be classified into groups based on material composition, fabrication technology, and application, as shown in Fig. 3. The figure also provides a timeline of when the technology was introduced.
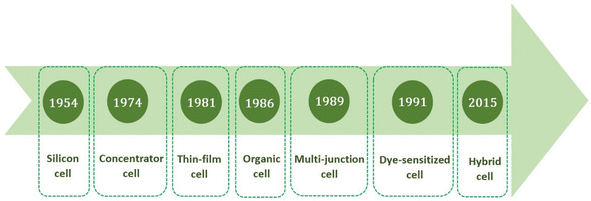 |
| Fig. 3 Types of PV cells on a timeline. | |
Silicon-based cells are the most common type of photovoltaic cells, comprising over 90% of the global PV market. They were first invented by Bell Labs in 1954 and are used in residential and commercial solar power systems.57,58 Crystalline silicon cells are made from silicon wafers, while amorphous silicon cells are made from a thin film of silicon deposited on a substrate. While crystalline silicon cells have high efficiency, durability, and reliability, amorphous silicon cells are less efficient but cheaper to produce and can be used in flexible and lightweight applications.
Thin-film cells are another type of photovoltaic cells made from materials like CdTe, CIGS, and amorphous silicon. The first thin-film solar cell, made from CdTe, was developed by the U.S. government's National Renewable Energy Laboratory in 1981.59 Thin-film cells are cheaper to produce and have a lower environmental impact than silicon-based cells. CdTe cells are widely used in utility-scale solar projects, while CIGS cells are used in residential and commercial applications due to their higher efficiency.60,61 Amorphous silicon cells are suitable for small-scale applications like pocket calculators and electronic watches.
Dye-sensitized cells (DSSC) are another type of photovoltaic cells that use a photosensitive dye to absorb light and generate electricity.62 Brian O'Regan and Michael Grätzel co-invented the contemporary edition of a DSSC in 1988, which they continued to refine until the release of the initial high-performance DSSC in 1991. These cells are less efficient than silicon-based cells but are cheaper to produce and can be made into flexible and transparent materials. DSSCs are used in portable devices, building-integrated photovoltaics, and other low-power applications.
Organic cells use organic materials such as polymers63–65 to generate electricity. The first organic solar cell was reported by researchers at the University of California, Santa Barbara in 1986. These cells are lightweight, flexible, and have a low environmental impact.66–68 However, their efficiency is lower than that of silicon-based cells, and they have a shorter lifespan.69 Organic cells are used in small-scale applications such as portable devices and flexible electronics.70,71
Multi-junction cells are photovoltaic cells made from layers of different materials that can absorb different wavelengths of light. The first multi-junction solar cell was made by the U.S. Air Force Research Laboratory in 1989.72,73 These cells are used in high-concentration photovoltaic systems and space applications. Multi-junction cells have the highest efficiency among all photovoltaic cells, with a record efficiency of 47.1% in the laboratory.
Concentrator cells use lenses or mirrors to focus sunlight onto a small area of a solar cell. This can significantly increase the efficiency by reducing the amount of material needed to generate electricity.74,75 The first concentrator solar cell was made by Boeing in 1974. Concentrator cells are used in utility-scale solar projects and high-concentration PV systems.76–78
Hybrid cells, some of which are highlighted in ref. 78–84, use a combination of different types of materials to achieve higher efficiencies. In 2015, the first-ever of perovskite/silicon tandem solar cell with two terminals was introduced.85 Perovskite cells have high efficiency but are not durable, while silicon solar cells have lower efficiency but are durable. By combining these two types, a hybrid cell can achieve both high efficiency and durability.86,87
It is important to note that the development of these photovoltaic cell technologies is ongoing, and new advancements and breakthroughs are constantly being made. However, relying on the above summarized descriptions, a general comparison for different types of PV cells can be made as in Table 2 that demonstrates that OPV cells have attracted significant attention among PV technologies due to their several benefits. Firstly, they offer the potential for low-cost, sustainable production due to their use of organic materials and low-energy manufacturing processes. Secondly, their flexibility and lightweight design make them ideal for use in a variety of applications, including indoor and portable settings where direct sunlight may not be available. Thirdly, their performance in low-light conditions is better than that of traditional silicon-based PV cells. Finally, because OPV technology is still in its early stages of development, there is considerable scope for innovation and discovery in the field, providing opportunities for cutting-edge research and new applications.
Table 2 A general comparison for different types of PV cells
Technology |
Advantages |
Disadvantages |
Silicon-based |
Most efficient technology with the longest track record |
Expensive to produce and manufacture |
|
High efficiency with a proven track record |
Limited flexibility |
|
Wide availability of raw materials |
Energy-intensive to produce |
|
|
Susceptible to shading, soiling, and temperature variations |
Thin-film |
Cheaper to produce than silicon-based cells |
Lower efficiency compared to silicon-based cells |
|
Flexible, lightweight, and adaptable to various surfaces |
Shorter lifespan than silicon-based cells |
|
Wide range of materials available for thin-film production |
Can be less stable under prolonged exposure to sunlight |
|
|
May contain toxic materials |
Concentrator |
Higher efficiency compared to other PV technologies |
Requires direct sunlight, making it less adaptable |
|
Uses less photovoltaic material, reducing cost |
Requires sophisticated tracking systems |
|
|
Requires specialized design, making it less accessible |
Multi-junction |
High efficiency for concentrated sunlight applications |
Expensive and complex to manufacture |
|
Ideal for space applications |
Less suitable for terrestrial applications |
|
Wide range of materials available for multi-junction cells |
Not yet cost-competitive for large-scale production |
Organic |
Cheaper and easier to produce than silicon-based cells |
Lower efficiency compared to other PV technologies |
|
Can be manufactured using low-cost printing methods |
Limited lifespan due to degradation of organic materials |
|
Flexible and lightweight, allowing for greater versatility |
Susceptible to temperature and moisture variations |
|
|
Less stable under prolonged exposure to sunlight |
Dye-sensitized |
Cheap to produce and can be manufactured using printing |
Low efficiency compared to other PV technologies |
|
Flexible and lightweight, allowing for greater versatility |
Susceptible to temperature and moisture variations |
|
Can be manufactured in different colors and transparency |
Shorter lifespan than other PV technologies |
|
|
Relatively new technology, still being developed |
Hybrid |
Potentially high efficiency, combining multiple technologies |
Limited lifespan due to degradation of organic materials |
|
Cheaper and easier to produce than some other technologies |
Still in development, not yet widely available |
|
Can be manufactured using low-cost printing methods |
Requires specialized design, making it less accessible |
|
More stable than some organic and dye-sensitized cells |
Requires further research and development |
4. Organic PV cells
Organic photovoltaic (OPV) cells, also known as organic solar cells, are a type of solar cell that converts sunlight into electricity using organic materials such as polymers and small molecules.83,84 These materials are carbon-based and can be synthesized in a laboratory, unlike inorganic materials like silicon that require extensive mining and processing.84,85 OPV cells work by absorbing photons of light and generating an electrical current through the flow of electrons in the organic material.86,87 The cells are typically made up of multiple layers, including a layer of organic material sandwiched between two electrodes.88 When light is absorbed, it creates a flow of electrons from one electrode to the other, producing a current.89–91
OPVs currently have lower efficiency levels, typically around 5–10%, compared to 15–20% for silicon-based cells.92–95 Despite this, research in the field is ongoing and scientists are working to improve the efficiency of polymer-based solar cells through various methods such as incorporating new materials and optimizing the cell structure.96,97 In recent years, the rapid increase in the power conversion efficiency of OPVs has led to increased scientific and economic interest.98–101
4.1. Working principles of OPV cells
4.1.1 Absorption of light. When light is incident on the active layer of the OPV cell, the organic materials absorb photons and generate electron–hole pairs. The process of light absorption in an OPV cell involves the interaction of photons with the organic semiconducting materials in the active layer of the cell. When a photon with energy equal to or greater than the bandgap energy of the material is absorbed, it generates electrical current. Therefore, the materials are designed to have strong absorption in this spectral range to maximize the conversion of sunlight into electrical power. The absorption of light in organic materials is influenced by various factors such as the chemical structure, molecular weight, and orientation of the materials.102 For example, the use of conjugated polymers, such as polythiophenes and polyfluorenes, has been shown to increase the absorption coefficient and extend the absorption range into the near-infrared region. Additionally, the morphology of the active layer plays a crucial role in light absorption. The active layer of an OPV cell is typically a blend of an electron-donor material and an electron-acceptor material, which form a heterojunction that facilitates efficient charge separation.103,104 The morphology of this blend is crucial to ensuring that the donor and acceptor materials are in intimate contact and that the excitons generated by light absorption are efficiently separated and transported to the respective electrodes. To increase the absorption of light in OPV cells, researchers have developed various strategies, including the use of light-trapping structures and the incorporation of plasmonic nanoparticles into the active layer. Light-trapping structures, such as textured substrates or photonic crystals, increase the path length of light in the active layer and enhance light absorption. Plasmonic nanoparticles, such as gold or silver, can be used to enhance the absorption of light through the excitation of surface plasmon resonance, which can confine and concentrate electromagnetic fields in the active layer.104,105 In conclusion, the absorption of light is a fundamental process in OPV cells, which is strongly influenced by the properties of the organic semiconducting materials and the morphology of the active layer. Researchers are continuing to develop new materials and strategies to improve light absorption and increase the efficiency of OPV cells.106,107
4.1.2 Charge separation. The electron–hole pairs are separated by a built-in electric field that exists within the active layer. This electric field is created by a difference in the energy levels of the donor and acceptor materials that make up the active layer. The process of charge separation involves the separation of the electron–hole pair generated by the absorption of light in the organic semiconducting material in the active layer of the cell.108 Efficient charge separation is essential to ensure that the generated charges can be collected at the respective electrodes to generate a current. The active layer of an OPV cell typically consists of a blend of an electron-donor material and an electron-acceptor material, which form a heterojunction that facilitates charge separation. The donor material, such as a conjugated polymer, is designed to have a high ionization potential, meaning that it has a strong tendency to donate an electron. The acceptor material, such as a fullerene or non-fullerene acceptor, is designed to have a low electron affinity, meaning that it has a strong tendency to accept an electron.109,110 When a photon is absorbed in the active layer, an exciton is generated, which is a bound state of an electron and a hole. The exciton is then dissociated into free charge carriers at the donor–acceptor interface, and the electron and hole are separated and transported to the respective electrodes. The morphology of the active layer plays a crucial role in charge separation. The donor and acceptor materials must be in intimate contact to ensure that the exciton can efficiently dissociate into free charge carriers. The morphology is typically optimized through the use of solvent processing or annealing to ensure that the donor and acceptor materials form a well-ordered and continuous heterojunction. The electronic properties of the donor and acceptor materials also play a significant role in charge separation. The energy levels of the donor and acceptor materials must be appropriately aligned to facilitate efficient charge separation.109,111 The energy offset between the donor and acceptor materials determines the driving force for charge separation, and an appropriate offset is required to ensure that the generated charges can be efficiently collected at the respective electrodes.112,113 The working principle of OPV is demonstrated in Fig. 4.114 To improve charge separation in OPV cells, researchers have developed various strategies, including the use of alternative acceptor materials, such as non-fullerene acceptors, and the development of tandem structures, which allow for multiple exciton dissociation and can enhance the overall efficiency of the device.
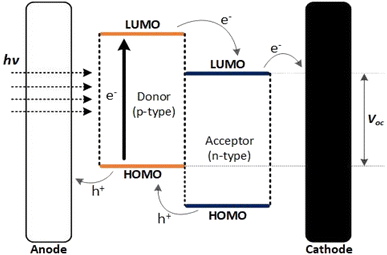 |
| Fig. 4 Working principles of the OPV.114 | |
4.1.3 Charge collection. The separated electrons and holes are collected by electrodes, which are typically made of transparent conductive materials that are coated onto the surface of the active layer. The process of charge collection in OPV cells involves the movement of separated charges to their respective electrodes to generate a current. Charge collection is a critical process and its efficiency directly affects the overall performance of the device.115 In OPV cells, the separated charges, which are generated by the process of charge separation, are transported to their respective electrodes through the organic semiconducting material in the active layer. The electrodes, typically made of conductive materials such as indium tin oxide (ITO), aluminum, or silver, are in contact with the active layer and collect the generated charges.116,117 The efficiency of charge collection is influenced by several factors, including the morphology of the active layer, the mobility of the charge carriers, and the alignment of the energy levels of the donor and acceptor materials.
4.1.4 Electrical output. The electrical output of an OPV cell is the result of the charge separation and collection processes. The efficiency of the electrical output is a key factor in determining the overall performance of the device. The electrical output of an OPV cell is characterized by its short-circuit current density (Jsc), open-circuit voltage (Voc), fill factor (FF), and power conversion efficiency (PCE).118,119 For a comprehensive understanding of these terms and their consequential effects on cell efficiency, Section 4.4 offers a detailed information.
4.2. Device structure of OPV cells
As shown in Fig. 5, recent research on OPV cells has revealed that various structures have been developed over time, each with its own unique advantages and disadvantages.117,120–122 These structures are composed of the materials described in the following sections.
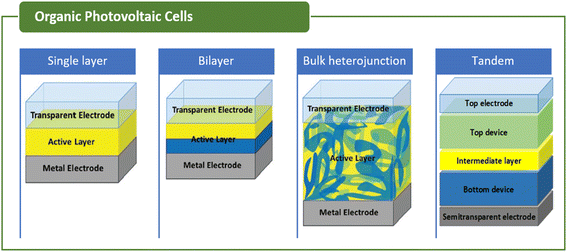 |
| Fig. 5 Device structure of OPV cells. | |
4.2.1 Single-layered OPV cells. These are the simplest type of OPV cells, consisting of only one layer of organic material that is designed to efficiently harvest light and generate electrical power. Single-layered OPV cells typically have lower efficiency compared to other types of OPV cells. In single-layer OPV cells, the donor and acceptor materials are mixed together in the same layer, which allows for a simpler device architecture and potentially lower production costs.116 When light is incident on the donor–acceptor layer, it generates electron–hole pairs that are separated by the built-in electric field. The separated electrons and holes are then collected by the electrodes to create a flow of electrical current, which can be used to power external devices or stored in a battery.The single-layer OPV cell typically includes the following layers.
Transparent electrode. This layer is typically made of a transparent conductive oxide material such as indium tin oxide (ITO) or zinc oxide (ZnO), and is used as the anode.
Donor–acceptor blend. This layer is a single layer that combines both the donor and acceptor materials. The donor material is typically a polymer or small molecule that absorbs light and generates electrons, while the acceptor material is typically a fullerene derivative that accepts electrons.
Metal electrode. This layer is typically made of a metal such as aluminum or silver, and is used as the cathode.
4.2.2 Bilayer OPV cells. Bilayer OPVs are a type of thin-film solar cell that consist of two organic semiconductor layers sandwiched between two electrodes, where the two layers are typically an electron-donating (or p-type) layer and an electron-accepting (or n-type) layer. In bilayer OPVs, the electron-donating layer is typically a conjugated polymer or a small molecule with a low ionization potential, while the electron-accepting layer is usually a fullerene derivative or a non-fullerene acceptor with a high electron affinity.119,120 The two layers are usually deposited using solution-based methods such as spin-coating. When light strikes the bilayer OPV, it creates an exciton in the electron-donating layer. The exciton is then separated into an electron and a hole, which are transported to the electron-accepting layer and the anode, respectively. The electron and hole then flow through their respective layers and the external circuit, creating a photocurrent that can be used to generate electricity.
4.2.3 Bulk heterojunction OPV cells. Bulk heterojunction OPV cells are a type of thin-film solar cell that consist of an interpenetrating network of electron-donating and electron-accepting materials.121 In a bulk heterojunction OPV cell, the electron-donating and electron-accepting materials are typically conjugated polymers or small molecules that are blended together in a solution and deposited onto a substrate using spin-coating or other solution-based methods. The blend forms an interpenetrating network of donor and acceptor materials, which allows for efficient charge separation and transport. The anode and cathode are the two electrodes that sandwich the blend of electron-donating and electron-accepting materials.122,123The anode is typically made of a transparent conductive oxide such as indium tin oxide or fluorine-doped tin oxide, while the cathode is typically made of a metal such as aluminum, silver, or gold. When sunlight strikes the bulk heterojunction OPV cell, it creates an exciton in the electron-donating material. The exciton is then separated into an electron and a hole, which are transported to the electron-accepting material and the anode, respectively. The electrons and holes then flow through their respective materials and the external circuit, creating a photocurrent that can be used to generate electricity.124
4.2.4 Tandem OPV cells. A tandem PV cell typically consists of two or more sub-cells that are connected in series to improve the efficiency of the device. The sub-cells can be made of different materials and have different band gaps to allow them to absorb different parts of the solar spectrum. In the tandem PV cell, each sub-cell is comprised of an absorber material and an electron transport material.122,125 The absorber material is responsible for absorbing the incoming photons and generating electron–hole pairs (excitons), while the electron transport material facilitates the separation and transport of the generated charge carriers.The junction between the two sub-cells allows for the efficient transfer of charge carriers between the two sub-cells. In a tandem PV cell, the bandgap of the first sub-cell is typically higher than the bandgap of the second sub-cell, which allows the first sub-cell to absorb the high-energy photons while the second sub-cell absorbs the low-energy photons.126,127 Overall, the use of tandem PV cells can improve the efficiency of a solar cell by allowing for a wider range of the solar spectrum to be absorbed and converted into electricity.
4.2.5 A brief comparison for device structures. As given within the previous sections, different types of OPV structures have been developed, each with unique advantages and disadvantages. Table 3 makes a brief comparison focusing on some key features. This comparison will provide insights into the performance and potential of these structures, and help identify the most promising candidates for future research and development.
Table 3 A brief comparison between device structures of OPV cells
Structure |
Advantages |
Disadvantages |
Key features |
Single-layered |
Simple device architecture and potentially lower production cost |
Lower efficiency compared to other OPV cells |
Single layer of organic material that combines both the donor and acceptor materials, three layers in total |
Bilayer |
Higher efficiency compared to single-layered OPV cells |
More complex device architecture than single-layered OPV cells |
Two layers of organic semiconductor sandwiched between two electrodes, solution-based methods such as spin-coating |
Bulk heterojunction |
High efficiency and excellent flexibility |
Complexity in blending donor and acceptor materials |
Interpenetrating network of electron-donating and electron-accepting materials, typically deposited by spin-coating |
Tandem |
Higher efficiency due to wider range of solar spectrum |
More complex device architecture than single-layered OPV cells |
Two or more sub-cells connected in series, with different band gaps to absorb different parts of the solar spectrum |
4.3. Parts of the OPV cells
The basic structure of an OPV cell involves the use of several materials, including organic semiconducting materials for the active layer, conductive materials for the electrodes, and encapsulation materials for protection.128,129 The performance of these materials is critical to the efficiency and durability of the OPV cell, and ongoing research is focused on improving their properties and developing new materials to enhance the performance and reduce the cost of OPV cells.
4.3.1 Organic semiconducting materials. The active layer of an OPV cell typically consists of a blend of electron-donor and electron-acceptor materials, which together form an organic semiconductor.130 These two materials function together to create a charge-separated state upon exposure to light, leading to the production of electrical energy. Without either of these components, the OPV would be unable to operate effectively. Therefore, the proper selection and pairing of these materials are critical to achieving high efficiency and stability in OPVs. Donor–acceptor molecules typically have a conjugated backbone consisting of alternating electron-rich (donor) and electron-deficient (acceptor) moieties. This allows for efficient charge transfer and separation when the material is exposed to sunlight.131 Some of the most frequently used donor–acceptor compounds in OPV cells are conjugated polymers and small molecules as electron donors illustrated in Fig. 6(a) and fullerene and non-fullerene derivatives as electron acceptors illustrated in Fig. 6(b).130–134
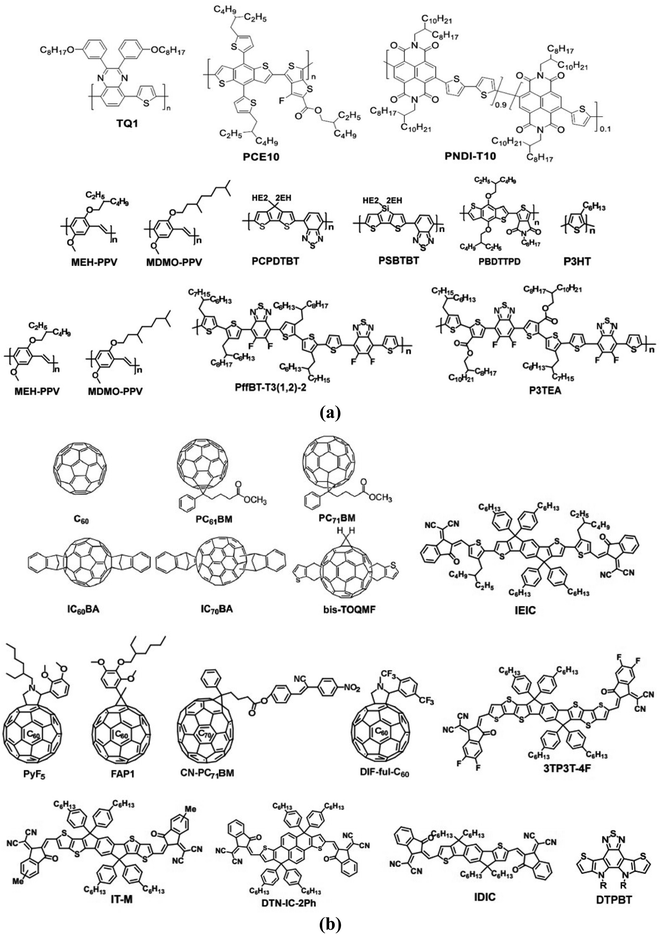 |
| Fig. 6 Recent advances on the chemical structures of (a) donor molecules (b) acceptor molecules.130–134 | |
The conjugated polymer can be designed with a low ionization energy, allowing it to donate electrons when excited by light. In contrast, the fullerene derivative has a high electron affinity, enabling it to accept electrons from the excited polymer. The resulting exciton dissociation leads to the generation of free electrons and holes, which can then be collected at the electrodes to produce an electric current. The selection of donor–acceptor compounds depends on factors such as their absorption spectra, energy levels, and solubility, as well as the desired efficiency and stability of the OPV cell.
In recent years, there has been a significant amount of research focused on the development of organic semiconductors for use in OPV cells.135,136 The performance of these devices is highly dependent on the electronic properties and structure–function relationships of the materials used.137,138 As a result, a deep understanding of these properties and relationships is essential for designing and developing high-performance organic semiconductors for use in OPV cells.139
4.3.2 Electrodes. OPV cells typically have two types of electrodes: the transparent conductive electrode (TCE) and the metallic electrode.140 The TCE is typically made of materials such as indium tin oxide (ITO) or fluorine doped tin oxide (FTO) and is placed on the side of the cell facing the incident light.141,142 This electrode is transparent so that the light can pass through it and reach the active layer, and it is electrically conductive so that it can collect the electrons produced by the active layer. The metallic electrode, on the other hand, is typically made of materials such as aluminum or silver and is placed on the opposite side of the cell, away from the incident light.143 This electrode is not transparent, but highly reflective in that it can reflect back from the cell any light that is not absorbed by the active layer to increase the chance of absorption. There are also different types of OPV cells that can use different materials and configurations for the electrodes, depending on the particular design and application.144,145
4.3.3 Encapsulation materials. Encapsulation is an important step in the manufacturing process of OPV cells as it protects the device from moisture, oxygen, and other environmental factors that can degrade the performance and lifetime of the device. The encapsulation materials used in OPV cells are typically selected based on their ability to provide a barrier against moisture and oxygen while still allowing light to pass through.146,147 Glass is a common encapsulation material for OPV cells, but it can be brittle and crack under stress. Polymer films, such as PET and PEN, are flexible and lightweight, making them a good choice for encapsulating OPV cells. Metal foils, such as aluminum and copper, provide a high barrier to moisture and oxygen but are less suitable for encapsulating flexible OPV cells.148,149 Thin film coatings like SiO2 and TiO2 are also used as encapsulation materials for OPV cells, but their application requires specialized equipment and processes. The choice of encapsulation material for OPV cells depends on the specific requirements of the device, such as flexibility, transparency, and barrier properties.150,151
4.4. Performance and efficiency of OPV cells
The performance and electrical characteristics of photovoltaic cells play a crucial role in determining the efficiency and effectiveness of photovoltaic systems. Performance of photovoltaic cells is measured by various parameters such as power output, efficiency, and fill factor.152,153 The power output of a photovoltaic cell is defined as the maximum power that it can generate under standard test conditions.154 The efficiency of a photovoltaic cell is expressed as:
where η is the efficiency, Pout is the output power and Pin is the incoming solar power. The fill factor (FF) of a photovoltaic cell is defined as the ratio of the maximum power to the product of the open-circuit voltage (VOC) and the short-circuit current (ISC). It is given by:
where Pmax is the maximum power output of the cell. The electrical characteristics of photovoltaic cells include the open-circuit voltage (VOC), short-circuit current (ISC), and maximum power point voltage (Vmp). The open-circuit voltage is the voltage across the cell when no current is flowing through it, and it is given by:
where n is ideality factor, k is Boltzmann's constant, T is the temperature in Kelvin, q is the charge of an electron, IL is the light-generated current and I0 is the reverse saturation current. The short-circuit current is the current that flows through the cell when the voltage across it is zero and it is given by:
where e is the base of the natural logarithm. It should be noted that the short circuit current (ISC) represents the maximum current at zero voltage. Ideally, if V = 0, ISC = IL.
The maximum power point voltage (Vmp) is the voltage at which the photovoltaic cell generates the maximum power and it is given by:
Power conversion efficiency (PCE) is one of the most important parameters of photovoltaic cells. PCE is a term used to express how effective the device is at converting solar energy into electrical energy.
In addition to the above-mentioned characteristics, the temperature coefficient of photovoltaic cells is also an important parameter that needs to be considered. This coefficient refers to the change in the performance of the cell with respect to temperature changes. Photovoltaic cells generally have a negative temperature coefficient, meaning that their performance decreases with an increase in temperature.155,156
The National Renewable Energy Laboratory (NREL) is a reputable research center that specializes in renewable energy and energy efficiency.157 They publish detailed reports on these topics and maintain a chart that tracks the highest confirmed conversion efficiencies for research cells across various photovoltaic technologies. This chart spans from 1976 to the present day and serves as a valuable tool for tracking the historical development of PV cell technology. NREL's data is particularly noteworthy, as it provides a comprehensive view of the progression of PV cell technology across different structures. Fig. 7 in their report illustrates this development over time. Additionally, the report includes a more detailed graph, shown in Fig. 8, which specifically focuses on OPVs.
 |
| Fig. 7 A chart of the highest confirmed conversion efficiencies for PV cells.157 | |
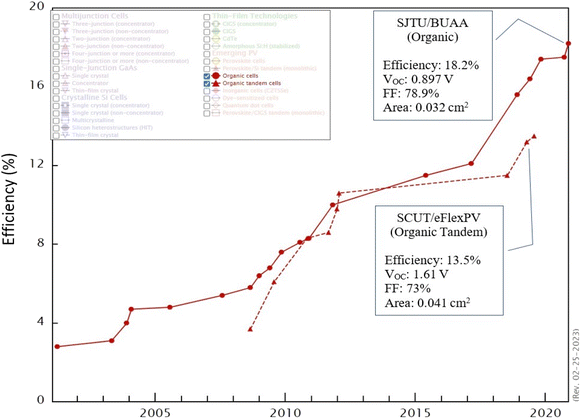 |
| Fig. 8 Conversion efficiencies for OPV cells from 2001 to 2021.157 | |
Fig. 7 and 8 demonstrate that current PV cell technologies are capable of achieving efficiencies greater than 40%. However, this level of efficiency is currently limited to multi-junction and concentrated cells, which are efficient but not yet widely used due to their high cost and limited economic feasibility. A similar scenario is also observed in cells based on inorganic materials. In the case of organic cell technology, current efficiencies can reach up to 18%. While this is not as high as multi-junction or concentrated cells, it is noteworthy given the lower cost and greater feasibility of organic cells. Continued research and development in all areas of PV cell technology will be necessary to further improve efficiency, reduce costs, and increase economic viability.
Recent literature on the OPV cell technology corroborates the efficiency rates reported by NREL. For example, recent review studies conducted by Li Y. et al.158 and H. Gao et al.159 reported that the latest developments in OPV cells have achieved a PCE% of up to 18.6% and an FF% of approximately 80%. These findings are consistent with the data presented in NREL's report. Fig. 9, which depicts reported PCE and FF rates of OPV cells in above references, provides advances achieved in recent studies. As seen, the FF% values range from 54% to 81.5%, while the PCE% values range from 5.72% to 18.6%. The highest FF% and PCE% values reported are 81.5% and 18.6%, respectively, while the lowest values reported are 54% and 5.72%, respectively.
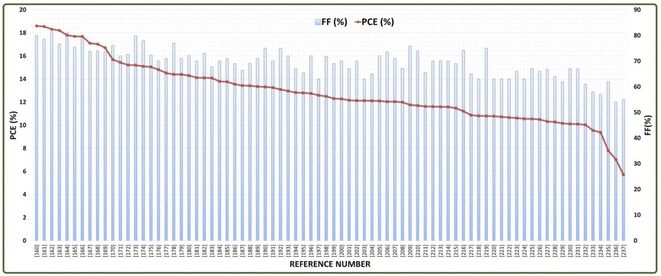 |
| Fig. 9 Performance of organic solar cells reported in recent literature.160–237 | |
Fill factor is an important parameter that measures how effectively a solar cell can convert incident light into electrical power. A higher FF% value indicates that the device can collect a larger fraction of the generated current, which results in higher output power. In Fig. 9, the average FF% value is 70.2%, which indicates that most of the devices have good fill factors.
Similarly, power conversion efficiency (PCE) is the most commonly used parameter to compare the performance of different solar cell technologies. It measures the percentage of incident solar energy that can be converted into electrical power. The highest PCE% value reported in Fig. 9 is 18.6%, which is close to the current record for OPV cells. However, the average PCE% value is only 13.7%, indicating that there is still significant room for improvement.
Fig. 9 also verifies that there exists a positive relationship between fill factor and power conversion efficiency in photovoltaic cells. Specifically, a higher fill factor typically results in a higher power conversion efficiency. This can be attributed to the fact that a higher fill factor indicates that a larger portion of the incident light is being converted into useable electrical power, which in turn leads to an increase in overall efficiency. However, there is typically a trade-off between fill factor and power conversion efficiency. This is because improving one parameter often comes at the expense of the other. For example, increasing the fill factor can be achieved by reducing the resistance of the solar cell, but this can also lead to an increase in the recombination rate of charge carriers, which can reduce the overall efficiency of the cell. Similarly, increasing the power conversion efficiency can be achieved by improving the collection of charge carriers, but this can also lead to an increase in the dark current, which can reduce the fill factor. Therefore, the optimal balance between fill factor and power conversion efficiency depends on the specific design and operating conditions of the solar cell, and requires careful optimization.
5. Evaluation and assessment
OPV cells have the potential to offer a sustainable and eco-friendly alternative to traditional solar cells, with low production costs and design flexibility. However, they also face challenges in terms of efficiency, durability, and competition from established renewable energy technologies. Table 4 presents a detailed SWOT analysis for OPV cells, highlighting the key strengths, weaknesses, opportunities, and threats facing this technology. The strengths of OPV cells, such as their low production costs and design flexibility, make them an attractive option for a range of applications, including consumer-facing products and emerging industries. The opportunities presented by growing demand for renewable energy sources, favorable government policies and incentives, and increasing consumer awareness of sustainability and environmental impact are all factors that could drive the growth and adoption of OPV cells in the future. However, the weaknesses and threats facing OPV cells cannot be ignored. The lower efficiency and durability of OPV cells compared to traditional solar cells present a challenge for their commercial viability, and competition from established renewable energy technologies may limit their market share. Additionally, the technological limitations and research challenges faced by OPV cells require significant investment and research, which may slow down their adoption and commercialization. Finally, the regulatory environment for renewable energy technologies is subject to change and may create uncertainty and risk for the adoption of OPV cells.
Table 4 SWOT analysis for OPV cells
Strengths |
Flexible and lightweight: OPV cells are thin and flexible, which makes them suitable for a range of applications including wearable technology, building-integrated photovoltaics, and solar-powered fabrics. They are also less susceptible to damage from wind and impact compared to rigid solar panels |
Low-cost production through printing techniques: OPV cells can be produced using roll-to-roll printing or other low-cost printing techniques, which reduces manufacturing costs and enables scalability. The use of printing techniques also allows for customization and the creation of unique shapes and designs |
Eco-friendly and easy to dispose of: OPV cells are made from non-toxic materials, such as carbon-based polymers, and are free of rare and expensive materials like silicon and metals. This makes them environmentally friendly and easy to dispose of at the end of their lifespan. OPV cells can also be recycled into new cells or other products |
Modular and scalable: OPV cells can be produced in different shapes and sizes, and can be interconnected to form modules or arrays. This allows for scalability and the customization of solar panels to fit specific applications and installation sites |
Low-light performance: OPV cells have a higher efficiency under low-light conditions compared to traditional silicon-based solar cells. This makes them suitable for use in regions with less sunlight, or for indoor applications such as powering smart buildings and IoT devices |
Weaknesses |
Limited efficiency compared to traditional silicon-based solar cells: OPV cells have a lower conversion efficiency compared to traditional silicon-based solar cells. They typically have an efficiency of 10–20%, while silicon-based solar cells are higher than this rate. This limits the power output of OPV cells, which may not be sufficient for certain applications |
Durability and stability issues: OPV cells are less durable and stable compared to traditional solar cells, and their performance may degrade over time due to exposure to UV light, moisture, and other environmental factors. The encapsulation and protection of OPV cells is a key challenge that needs to be addressed to improve their durability |
Low production volume compared to traditional solar cells: OPV cells are produced in much smaller quantities compared to traditional silicon-based solar cells, which makes them less commercially viable at large-scale production. This also limits the availability of OPV cells and may increase their cost |
Sensitivity to temperature: OPV cells have a lower operating temperature range compared to silicon-based solar cells. They may degrade or malfunction in high temperatures, reducing their efficiency and lifespan |
Limited lifespan: OPV cells have a shorter lifespan compared to traditional silicon-based solar cells, typically around 10–15 years. This may make them less suitable for certain applications that require long-term reliability and durability |
Opportunities |
Development of new materials: advances in the development of new materials for OPV cells may lead to improved performance and stability |
Growing demand for renewable energy sources: the increasing focus on reducing carbon emissions and transitioning to renewable energy sources creates a significant opportunity for OPV cells, as they offer a sustainable and eco-friendly alternative to traditional solar cells |
Favorable government policies and incentives: governments offer subsidies, tax incentives, and other financial incentives for the adoption of renewable energy technologies. These policies create a favorable environment for the development and deployment of OPV cells |
Growing consumer awareness and demand for sustainable and environmentally friendly products: consumers are becoming more aware of the environmental impact of their choices and are seeking out more sustainable and eco-friendly products. This trend creates an opportunity for OPV cells to gain market share and be adopted in a range of consumer-facing applications |
Emerging markets and industries for OPV cells: OPV cells offer a range of new opportunities in industries such as agriculture, transportation, and architecture. In agriculture, they can be used to power irrigation systems and crop monitoring systems, while in transportation, they can be used to power electric vehicles, boats, and drones. In architecture, they can be integrated into building facades, windows, and roofs to provide solar power and reduce energy consumption |
Collaboration and investment from major companies: many companies are investing in the development and production of OPV cells, which provides an opportunity for collaboration and knowledge sharing to further improve the technology and increase its adoption. This may make them less suitable for certain applications that require long-term reliability and durability |
Threats |
Competition from traditional solar cells and other renewable energy technologies: traditional silicon-based solar cells and other renewable energy technologies, such as wind and hydropower, are well-established and offer higher efficiency and reliability compared to OPV cells. This creates a threat to the adoption and commercial viability of OPV cells |
Technological limitations and research challenges: OPV cells face a range of technological limitations and research challenges, such as improving their efficiency, durability, and stability. Addressing these challenges requires significant investment and research, which may slow down the adoption and commercialization of OPV cells |
Regulatory and policy risks: the regulatory environment for renewable energy technologies is subject to change and may create uncertainty and risk for the adoption of OPV cells. Changes in government policies and regulations could limit the development of the OPV cell industry |
Supply chain risks: OPV cells' complex supply chain involves material production, manufacturing, and distribution. Any disruption, such as material or component shortages, could affect their availability and cost |
Economic risks: the commercial success of OPV cells depends on production costs, market demand, and competition. Any changes to these economic factors can threaten the industry's adoption and commercialization |
As clearly seen in Table 4, organic PV cells have a natural advantage over other types of PV cells due to their transparent characteristics, which make them ideal for integration with building-integrated photovoltaics, such as windows. However, a critical challenge for efficient organic PV cells is the trade-off between average visible light transmittance (AVT) and power conversion efficiency (PCE). The recent development of materials that yield simultaneously high levels of efficiency and transparency brings the opportunity to enter important niche markets. Researchers have successfully designed and constructed a superior transparent rear electrode for efficient transparent organic photovoltaics via integrating an aperiodic band-pass filter (ABPF). The integrated rear electrode exhibits an AVT of up to 78.69%, a color-rendering index (CRI) of 97.54, and a total reflection in the near-infrared region (700–900 nm). As a result, ABPF-integrated transparent organic photovoltaics demonstrate a record-breaking light utilization efficiency (LUE) of 5.35%, accompanied with an AVT of 46.79% and CRI of 85.39. Reinforcing research efforts on topics such as improvement in device lifetime, color portfolio, and module design and efficiency will help in making a significant effect on changing the status of organic photovoltaics from a novel technology to a mature industry.238–240
Similar to their transparent characteristics, one of the key features of OPV cells is their tunability, which refers to their ability to be easily modified to meet specific application requirements. Organic materials used in PV cells can be easily synthesized and modified, allowing for a high degree of control over the cell's optical and electrical properties. This means that the cells can be designed to absorb specific wavelengths of light, making them suitable for a range of applications, from powering small electronic devices to generating electricity on a larger scale. Another important feature of OPV cells is their versatility. Unlike traditional silicon-based solar cells, OPV cells can be manufactured using low-cost, flexible substrates, such as plastic or metal foils. This flexibility allows for the cells to be integrated into a variety of surfaces, including curved or irregular shapes, making them ideal for use in portable and wearable electronics, as well as building-integrated photovoltaic systems. Additionally, OPV cells have the potential to be produced in large quantities using roll-to-roll printing techniques, which could significantly reduce production costs and increase their accessibility to a wider range of applications.
On the other hand, to overcome the challenges emphasized in Table 4, it will be important for the OPV cell industry to focus on research and development to improve the efficiency, durability, and stability of the technology.241–246 Addressing these technological limitations will help to increase the commercial viability of OPV cells and enable them to compete with traditional solar cells and other renewable energy technologies. Additionally, collaboration with governments, policymakers, and stakeholders can help to create a more favorable regulatory environment for OPV cells and facilitate their adoption.
In conclusion, while OPV cells face significant challenges, their potential for sustainable and eco-friendly energy production is promising. The OPV cell industry should work towards addressing the weaknesses and threats identified in the SWOT analysis, while continuing to build on their strengths and opportunities, to enable the widespread adoption of this technology in the future.
6. Conclusion
This state-of-the-art review provides a comprehensive overview of the evolution of photovoltaic (PV) cell technology. It includes the classification of PV production technologies and a comparative analysis of first, second, and third-generation solar cells. The working principles and device structures of OPV cells are also explored and compared focusing on their advantages, disadvantages, and key features. Furthermore, the paper discusses the various parts of OPV cells and analyzes the performance, efficiency, and electrical characteristics of photovoltaic cells. The conversion efficiencies for OPV cells are presented, and a detailed SWOT analysis is conducted for OPV cells.
The SWOT analysis reveals a promising future for the technology, but also highlights several challenges and areas for improvement. Despite the strengths of OPV cells, the technology still faces weaknesses such as limited efficiency, durability and stability issues, sensitivity to temperature, and limited lifespan. However, there are opportunities to improve the technology, including the development of new materials, growing demand for renewable energy sources, favorable government policies and incentives, growing consumer awareness and demand for sustainable and environmentally friendly products, emerging markets and industries, and collaboration and investment from major companies. The review also identifies potential threats to the adoption and commercial viability of OPV cells, including competition from traditional solar cells and other renewable energy technologies, technological limitations and research challenges, regulatory and policy risks, supply chain risks, and economic risks.
The review indicates that OPV cells have the potential to revolutionize the solar energy industry due to their compatibility with printing technologies and the ability to produce thin, flexible solar cells. However, challenges such as preventing recombination, improving absorption in the visible to near-infrared part of the solar spectrum, and optimizing morphological characteristics for charge transport still exist. Despite these challenges, researchers are making steady progress, and the tunability and versatility of organic materials offer promise for future success. The potential applications for thin, flexible OPV cells are exciting, including powering remote or underdeveloped areas and charging internal devices.
Overall, this review highlights the significant advancements and challenges in the development of OPV cells. It is hoped that this paper will serve as a valuable resource for researchers, policymakers, and stakeholders interested in the development of sustainable and efficient solar energy technologies. Future research should focus on addressing the challenges identified in this paper and developing new materials and technologies that can further improve the performance and efficiency of OPV cells.
Conflicts of interest
There are no conflicts to declare.
Acknowledgements
Authors would like to thank to the Gazi University Scientific Research Projects Coordination Unit for their support of this study under project number FCD-2023-8308.
References
- A. G. Olabi and M. A. Abdelkareem, Renewable energy and climate change, Renewable Sustainable Energy Rev., 2022, 158, 1–7, DOI:10.1016/j.rser.2022.112111.
- L. Qiu, L. He, H. Lu and D. Liang, Systematic potential analysis on renewable energy centralized co-development at high altitude: A case study in Qinghai-Tibet plateau, Energy Convers. Manage., 2022, 267, 1–12, DOI:10.1016/j.enconman.2022.115879.
- A. Dutta, E. Bouri, T. Rothovius and G. S. Uddin, Climate risk and green investments: New evidence, Energy, 2023, 265, 1–13, DOI:10.1016/j.energy.2022.126376.
- K. A. Tahir, M. Zamorano and J. O. García, Scientific mapping of optimisation applied to microgrids integrated with renewable energy systems, Int. J. Electr. Power Energy Syst., 2023, 145, 1–18, DOI:10.1016/j.ijepes.2022.108698.
- M. Mišík, The EU needs to improve its external energy security, Energy Policy, 2022, 165, 1–5, DOI:10.1016/j.enpol.2022.112930.
- L. Li, W. Li and X. Li, A Power Grid Planning Method Considering Dynamic Limit of Renewable Energy Security Constraints, 2021 IEEE 5th Conference on Energy Internet and Energy System Integration (EI2), Taiyuan, China, 2021, pp. 1101–1105, DOI:10.1109/EI252483.2021.9713073.
- M. F. Rabbi, J. Popp, D. Máté and S. Kovács, Energy Security and Energy Transition to Achieve Carbon Neutrality, Energies, 2022, 15, 8126, DOI:10.3390/en15218126.
- C. A. Hossain, N. Chowdhury, M. Longo and W. Yaïci, System and Cost Analysis of Stand-Alone Solar Home System Applied to a Developing Country, Sustainability, 2019, 11(5), 1403, DOI:10.3390/su11051403.
- G. E. Halkos and E.-C. Gkampoura, Reviewing Usage, Potentials, and Limitations of Renewable Energy Sources, Energies, 2020, 13(11), 2906, DOI:10.3390/en13112906.
- N. S. M. N. Izam, Z. Itam, W. L. Sing and A. Syamsir, Sustainable Development Perspectives of Solar Energy Technologies with Focus on Solar Photovoltaic—A Review, Energies, 2022, 15(8), 2790, DOI:10.3390/en15082790.
- G. Li, M. Li, R. Taylor, Y. Hao, G. Besagni and C. N. Markides, Solar energy utilisation: Current status and roll-out potential, Appl. Therm. Eng., 2022, 209, 118285, DOI:10.1016/j.applthermaleng.2022.118285.
- H. S. Hussein, The state of the art of nanomaterials and its applications in energy saving, Bull. Natl. Res. Cent., 2023, 47, 7, DOI:10.1186/s42269-023-00984-4.
- B. Kumar, D. Garg, K. Swamy and P. Kumar, Clean Energy Production Using Solar Energy Resources, in Sustainable and Clean Energy Production Technologies. Clean Energy Production Technologies, ed. Pal, D. B. and Jha, J. M., Springer, Singapore, 2022, DOI:10.1007/978-981-16-9135-5_12.
- A. A. Chand, P. Lal, K. A. Prasad and N. M. Kumar, Economics and environmental impacts of solar energy technologies, Solar Energy Advancements in Agriculture and Food Production Systems, Academic Press, 2022, pp. 391–423, DOI:10.1016/B978-0-323-89866-9.00006-7.
- C. L. Crago and E. Koegler, Drivers of growth in commercial-scale solar PV capacity, Energy Policy, 2018, 120, 481–491, DOI:10.1016/j.enpol.2018.05.047.
- P. K. S. Rathore, D. S. Chauhan and R. P. Singh, Decentralized solar rooftop photovoltaic in India: On the path of sustainable energy security, Renewable Energy, 2019, 131, 297–307, DOI:10.1016/j.renene.2018.07.049.
- M. Ramadan, A review on coupling Green sources to Green storage (G2G): Case study on solar-hydrogen coupling, Int. J. Hydrogen Energy, 2021, 46(59), 30547–30558, DOI:10.1016/j.ijhydene.2020.12.165.J.
- N. A. F. M. N. Ong, M. Z. M. Tohir, M. S. M. Said, M. S. Nasif, A. H. Alias and M. R. Ramali, Development of fire safety best practices for rooftops grid-connected photovoltaic (PV) systems installation using systematic review methodology, Sustain. Cities Soc., 2022, 78, 103637, DOI:10.1016/j.scs.2021.103637.
- E. Barruna, A. Saniah, R. Bhaskara, S. F. Rahman, A. Zulfia and N. R. Poespawati, Carbon Nanotubes, Graphite, and Reduced Graphene Oxide in CuSCN incorporated Carbon Electrodes in Perovskite Solar Cells, 2022, 11th Electrical Power, Electronics, Communications, Controls and Informatics Seminar, Malang, Indonesia, 2022, pp. 119–122, DOI:10.1109/EECCIS54468.2022.9902945.
- R. Verduci, V. Romano, G. Brunetti, N. Y. Nia, A. Di Carlo, G. D'Angelo and C. Ciminelli, Solar Energy in Space Applications: Review and Technology Perspectives, Adv. Energy Mater., 2022, 12, 2200125, DOI:10.1002/aenm.202200125.
- L. Thomas, C. H. Don and J. D. Major, An investigation into the optimal device design for selenium solar cells, Energy Rep., 2022, 8(11), 14–22, DOI:10.1016/j.egyr.2022.05.045.
- L. M. Fraas, History of Solar Cell Development, Low Cost Solar Electric Power, Springer, Cham, 1st edn, 2014, ch. 1, DOI:10.1007/978-3-319-07530-3-1.
- D. E. Carlson and C. R. Wronski, Amorphous silicon solar cell, Appl. Phys. Lett., 1976, 28, 671, DOI:10.1063/1.88617.
- A. Kojima, K. Teshima, Y. Shirai and T. Miyasaka, Organometal halide perovskites as visible-light sensitizers for photovoltaic cells, J. Am. Chem. Soc., 2009, 131(17), 6050–6051, DOI:10.1021/ja809598r.
- S. B. Jathar, S. R. Rondiya, B. R. Bade, M. P. Nasane, S. V. Barma, Y. A. Jadhav, A. V. Rokade, K. B. Kore, D. S. Nilegave, P. U. Tandale, S. R. Jadkar and A. M. Funde, Facile Method for Synthesis of CsPbBr3 Perovskite at Room Temperature for Solar Cell Applications, ES Mater. Manuf., 2021, 12, 72–77, DOI:10.30919/esmm5f1036.
- R. S. Potember, R. C. Hoffman, H. S. Hu, J. E. Cocchiaro, C. A. Viands and T. O. Poehler, Electronic Devices from Conducting Organics and Polymers, Polym. J., 1987, 19, 147–156, DOI:10.1295/polymj.19.147.
- C. J. Brabec, N. S. Sariciftci and C. Hummelen, Plastic Solar Cells, Adv. Funct. Mater., 2001, 11, 15–26, DOI:10.1002/1616-3028(200102)11:1%3C15::AID-ADFM15%3E3.0.CO;2-A.
- R. Mathur and P. B. O'Connor, Design and implementation of a high power rf oscillator on a printed circuit board for multipole ion guides, Rev. Sci. Instrum., 2006, 77, 114101, DOI:10.1063/1.2387881.
- N. Nakao, M. Saito, T. Mikie, T. Ishikawa, J. Jeon, H. D. Kim, H. Ohkita, A. Saeki and I. Osaka, Halogen-Free π-Conjugated Polymers Based on Thienobenzobisthiazole for Efficient Nonfullerene Organic Solar Cells: Rational Design for Achieving High Backbone Order and High Solubility, Adv. Sci., 2023, 10, 2205682, DOI:10.1002/advs.202205682.
- Y. Li, X. Huang, K. Ding, H. K. M. Sheriff, L. Ye, H. Liu, C. Z. Li, H. Ade and S. R. Forrest, Non-fullerene acceptor organic photovoltaics with intrinsic operational lifetimes over 30 years, Nat. Commun., 2021, 12, 5419, DOI:10.1038/s41467-021-25718-w.
- Y. Cui, H. Yao, J. Zhang, T. Zhang, Y. Wang, L. Hong, K. Xian, B. Xu, S. Zhang, J. Peng, Z. Wei, F. Gao and J. Hou, Over 16% efficiency organic photovoltaic cells enabled by a chlorinated acceptor with increased open-circuit voltages, Nat. Commun., 2019, 10, 2515, DOI:10.1038/s41467-019-10351-5.
- R. A. M. Lameirinhas, J. P. N. Torres and C. J. P. de Melo, A Photovoltaic Technology Review: History, Fundamentals and Applications, Energies, 2022, 15, 1823, DOI:10.3390/en15051823.
- A. H. Alami, M. Ramadan, M. A. Abdelkareem, J. J. Alghawi, N. T. Alhattawi, H. A. Mohamad and A. G. Olabi, Novel and practical photovoltaic applications, Therm. Sci. Eng. Prog., 2022, 29, 101208, DOI:10.1016/j.tsep.2022.101208.
- S. Reber, W. Zimmermann and T. Kieliba, Zone melting recrystallization of silicon films for crystalline silicon thin-film solar cells, Sol. Energy Mater. Sol. Cells, 2001, 65, 409–416, DOI:10.1016/S0927-0248(00)00120-3.
- J. Pastuszak and P. Węgierek, Photovoltaic Cell Generations and Current Research Directions for Their Development, Materials, 2022, 15(16), 5542, DOI:10.3390/ma15165542.
- J. Zhao, Recent advances of high-efficiency single crystalline silicon solar cells in processing Technologies and substrate materials, Sol. Energy Mater. Sol. Cells, 2004, 82, 53–64, DOI:10.1016/j.solmat.2004.01.005.
- P. G. V. Sampaio and M. O. A. González, Photovoltaic solar energy: Conceptual framework, Renewable Sustainable Energy Rev., 2017, 74, 590–601, DOI:10.1016/j.rser.2017.02.081.
- M. A. Green, Y. Hishikawa, W. Warta, E. D. Dunlop, D. H. Levi, J. Hohl-Ebinger and A. W. H. Ho-Baillie, Solar cell efficiency tables (version 50), Prog. Photovoltaics, 2017, 25(7), 668–676, DOI:10.1002/pip.2909.
- C. B. Isabela, R. A. A. M. Lameirinhas, J. P. N. Torres and C. A. F. Fernandes, Comparative study of the copper indium gallium selenide (CIGS) solar cell with other solar technologies, Sustainable Energy Fuels, 2021, 5, 2273–2283, 10.1039/D0SE01717e.
- M. A. Green, Crystalline and thin-film silicon solar cells: state of the art and future potential, Sol. Energy, 2003, 74(3), 181–192, DOI:10.1016/S0038-092X(03)00187-7.
- N. Y. Doumon, L. Yang and F. Rosei, Ternary organic solar cells: A review of the role of the third element, Nano Energy, 2022, 94, 106915, DOI:10.1016/j.nanoen.2021.106915.
- E. Arici, H. Hoppe, F. Schäffler, D. Meissner, M. A. Malik and N. S. Sariciftci, Hybrid solar cells based on inorganic nanoclusters and conjugated polymers, Thin Solid Films, 2004, 451–452, 612–618, DOI:10.1016/j.tsf.2003.11.026.
- F. Kessler and D. Rudmann, Technological aspects of flexible CIGS solar cells and modules, Sol. Energy, 2004, 77, 685–695, DOI:10.1016/j.solener.2004.04.010.
- S. G. Hashmi, J. Halme, Y. Ma, T. Saukkonen and P. A Lund, Single-Walled Carbon Nanotube Coated Flexible PVC Counter Electrode for Dye-Sensitized Solar Cells, Adv. Mater. Interfaces, 2014, 1, 1300055, DOI:10.1002/admi.201300055.
- G. Michael, Dye-sensitized solar cells, J. Photochem. Photobiol., C, 2003, 4(2), 145–153, DOI:10.1016/S1389-5567(03)00026-1.
- S. Anandan, Recent improvements and arising challenges in dye-sensitized solar cells, Sol. Energy Mater. Sol. Cells, 2007, 91, 843–846, DOI:10.1016/j.solmat.2006.11.017.
- Z. W. Gao, Y. Wang and W. C. H. Choy, Buried Interface Modification in Perovskite Solar Cells: A Materials Perspective, Adv. Energy Mater., 2022, 12, 2104030, DOI:10.1002/aenm.202104030.
- C. J. Brabec, S. Gowrisanker, J. J. M. Halls, D. Laird, S. Jia and S. P. Williams, Polymer–Fullerene Bulk-Heterojunction Solar Cells, Adv. Mater., 2010, 22(4), 3839–3856, DOI:10.1002/adma.200903697.
- J. Nelson, Polymer: fullerene bulk heterojunction solar cells, Mater. Today, 2011, 14(10), 462–470, DOI:10.1016/S1369-7021(11)70210-3.
- W. S. Yang, J. H. Noh, N. J. Jeon, Y. C. Kim, S. Ryu, J. Seo and S. I. Seok, High-performance photovoltaic perovskite layers fabricated through intramolecular exchange, Science, 2015, 348(6240), 1234–1237, DOI:10.1126/science.aaa9272.
- Z. Li, Z. Wang, C. Jia, Z. Wan, C. Zhi, C. Li, M. Zhang, C. Zhang and Z. Li, Annealing
free tin oxide electron transport layers for flexible perovskite solar cells, Nano Energy, 2022, 94, 106919, DOI:10.1016/j.nanoen.2022.106919.
- Z. Wang, X. Zhu, J. Feng, C. Wang, C. Zhang, X. Ren, S. Priya, S. Frank and D. Yang, Antisolvent- and Annealing-Free Deposition for Highly Stable Efficient Perovskite Solar Cells via Modified ZnO, Adv. Sci., 2021, 8, 2002860, DOI:10.1002/advs.202002860.
- W. Hu, T. Liu, X. Yin, H. Liu, X. Zhao, S. Luo, Y. Guo, Z. Yao, J. Wang, N. Wang, H. Lin and Z. Guo, Hematite electron-transporting layers for environmentally stable planar perovskite solar cells with enhanced energy conversion and lower hysteresis, J. Mater. Chem. A, 2017, 5, 1434–1441, 10.1039/C6TA09174A.
- C. Chen, Y. Jiang, Y. Wu, J. Guo, X. Kong, X. Wu, Y. Li, D. Zheng, S. Wu, X. Gao, Z. Hou, G. Zhou, Y. Chen, J. Liu, K. Kempa and J. Gao, Low-Temperature-Processed WOx as Electron Transfer Layer for Planar Perovskite Solar Cells Exceeding 20% Efficiency, Sol. RRL, 2020, 4, 1900499, DOI:10.1002/solr.201900499.
- B. Gu, Y. Zhu, H. Lu, W. Tian and L. Li, Efficient planar perovskite solar cells based on low-cost spin-coated ultrathin Nb2O5 films, Sol. Energy, 2018, 166, 187–194, DOI:10.1016/j.solener.2018.03.054.
- M. Zhong, D. Yang, J. Zhang, J. Shi, X. Wang and C. Li, Improving the performance of CdS/P3HT hybrid inverted solar cells by interfacial modification, Sol. Energy Mater. Sol. Cells, 2012, 96, 160–165, DOI:10.1016/j.solmat.2011.09.041.
- M. A. Green, Estimates of Te and in prices from direct mining of known ores, Prog. Photovoltaics, 2009, 17, 347–359, DOI:10.1002/pip.899.
- D. Chapin, C. Fuller and G. Pearson, A new silicon p–n junction photocell for converting solar radiation into electrical power, J. Appl. Phys., 1954, 25, 676–677, DOI:10.1063/1.1721711.
- Y. S. Tyan and E. A. Albuerne, Efficient thin film CdS/CdTe solar cells, Proceedings of the 16th IEEE Photovoltaic Specialist Conference, New York, NY, USA, 27–30 September 1982 Search PubMed.
- J. Britt and C. Ferekides, Thin-film CdS/CdTe solar cell with 15.8% efficiency, Appl. Phys. Lett., 1993, 62, 2851–2852, DOI:10.1063/1.109629.
- A. Bosio, S. Pasini and N. Romeo, The History of Photovoltaics with Emphasis on CdTe Solar Cells and Modules, Coatings, 2020, 10, 344, DOI:10.3390/coatings10040344.
- B. C. Oregan and M. Grätzel, A low-cost, high-efficiency solar cell based on dye-sensitized colloidal TiO2 films, Nature, 1991, 353, 737–740, DOI:10.1038/353737a0.
- U. Farva, H. W. Lee, R. N. Kim, D. G. Lee, D. W. Kang and J. Kim, Growth temperature influence on atomic-layer-deposited In2O3 thin films and their application in inorganic perovskite solar cells, Nanomaterials, 2021, 11, 2047, DOI:10.3390/nano11082047.
- H. Fu, Y. Li, J. Yu, Z. Wu, Q. Fan, F. Lin, H. Y. Woo, F. Gao, Z. Zhu and K. Y. Alex, High Efficiency (15.8%) All-Polymer Solar Cells Enabled by a Regioregular Narrow Bandgap Polymer Acceptor Jen, J. Am. Chem. Soc., 2021, 143(7), 2665–2670, DOI:10.1021/jacs.0c12527.
- L. Lu, T. Zheng, Q. Wu, A. M. Schneider, D. Zhao and L. Yu, Recent Advances in Bulk Heterojunction Polymer Solar Cells, Chem. Rev., 2015, 115(23), 12666–12731, DOI:10.1021/acs.chemrev.5b00098.
- Y. Guo, Y. Li, O. Awartani, J. Zhao, H. Han, H. A. D. Zhao and H. Yan, A Vinylene-Bridged Perylenediimide-Based Polymeric Acceptor Enabling Efficient All-Polymer Solar Cells Processed under Ambient Conditions, Adv. Mater., 2016, 28(38), 8483–8489, DOI:10.1002/adma.201602387.
- G. J. Zhang, X. P. Xu, Y. W. Lee, H. Y. Woo, Y. Li and Q. Peng, Achieving a High Fill Factor and Stability in Perylene Diimide–Based Polymer Solar Cells Using the Molecular Lock Effect between 4,4′-Bipyridine and a Tri (8-hydroxyquinoline) aluminium (III) Core, Adv. Funct. Mater., 2019, 29, 1902079, DOI:10.1002/adfm.201902079.
- S. Dowland, T. Lutz, A. Ward, S. P. King, A. Sudlow, M. S. Hill, K. C. Molloy and S. A. Haque, Direct Growth of Metal Sulfide Nanoparticle Networks in Solid-State Polymer Films for Hybrid Inorganic–Organic Solar Cells, Adv. Mater., 2011, 23, 2739–2744, DOI:10.1002/adma.201100625.
- C. W. Tang, Two-Layer Organic Photovoltaic Cell, Appl. Phys. Lett., 1986, 48(2), 183–185, DOI:10.1063/1.96937.
- H. Spanggaard and F. C. Krebs, A brief history of the development of organic and polymeric photovoltaics, Sol. Energy Mater. Sol. Cells, 2004, 83(2–3), 125–146, DOI:10.1016/j.solmat.2004.02.021.
- A. Rafique, I. Ferreira, G. Abbas and A. Baptista, Recent Advances and Challenges Toward Application of Fibers and Textiles in Integrated Photovoltaic Energy Storage Devices, Nano-Micro Lett., 2023, 15, 40, DOI:10.1007/s40820-022-01008-y.
- J. M. Olson, S. R. Kurtz, N. H. Karam and C. Y. Tabery, AlGaAs/GaAs tandem solar cells with quantum well intermediate layers, Appl. Phys. Lett., 1990, 56(7), 655–657, DOI:10.1063/1.102730.
- S. R. Kurtz, Multi-junction solar cells for space power, Sol. Cells, 1992, 32(1–2), 15–22, DOI:10.1016/0379-6787(92)90242-X.
- L. L. Kazmerski, H. J. Hovel and G. A. Antypas, Concentrator solar cell development at Boeing, IEEE Trans. Electron Devices, 1976, 23(7), 671–679, DOI:10.1109/T-ED.1976.18517.
- L. L. Kazmerski, Forty years of concentrator photovoltaics research, Sol. Energy, 1999, 65(1), 75–78, DOI:10.1016/S0038-092X(98)00120-1.
- J. M. Saura, D. Chemisana, P. M. Rodrigo, F. M. Almonacid and E. F. Fernández, Effect of non-uniformity on concentrator multi-junction solar cells equipped with refractive secondary optics under shading conditions, Energy, 2022, 238, 122044, DOI:10.1016/j.energy.2021.122044.
- S. Alwin, V. Ramasubbu and X. S. Shajan, TiO2 aerogel–metal organic framework nanocomposite: a new class of photoanode material for dye-sensitized solar cell applications, Bull. Mater. Sci., 2018, 41(27), 2018, DOI:10.1007/s12034-017-1532-8.
- B. Chen, Z. Yang, Q. Jia, R. J. Ball, Y. Zhu and Y. Xia, Emerging applications of metal-organic frameworks and derivatives in solar cells: Recent advances and challenges, Mater. Sci. Eng., R, 2023, 152, 100714, DOI:10.1016/j.mser.2022.100714.
- P. R. Kumar, V. Ramasubbu, X. Sahaya Shajan and E. M. Mothi, Porphyrin-sensitized quasi-solid solar cells with MOF composited titania aerogel photoanodes, Mater. Today Energy, 2020, 18, 100511–100517, DOI:10.1016/j.mtener.2020.100511.
- W. Moloto, P. Mbule, E. Nxumalo and B. Ntsendwana, Stabilizing effects of zinc(II)-benzene-1,3,5-tricarboxylate metal organic frameworks on the performance of TiO2 photoanodes for use in dye-sensitized solar cells, J. Photochem. Photobiol., A, 2021, 407, 113063, DOI:10.1016/j.jphotochem.2020.113063.
- L. G. da Trindade, K. M. N. Borba, A. B. Trench, L. Zanchet, V. Teodoro, F. M. L. Pontes, E. Longo and T. M. Mazzo, Effective strategy to coupling Zr-MOF/ZnO: Synthesis, morphology and photoelectrochemical properties evaluation, J. Solid State Chem., 2021, 293, 121794, DOI:10.1016/j.jssc.2020.121794.
- H. S. Vogelbaum and G. Sauvé, Recently developed high-efficiency organic photoactive materials
for printable photovoltaic cells: a mini review, Synth. Met., 2017, 223(107–121), 0379–6779, DOI:10.1016/j.synthmet.2016.12.011.
- J. Ackermann, C. Videlot and A. El Kassmi, Growth of organic semiconductors for hybrid solar cell application, Thin Solid Films, 2002, 403–404, 157–161, DOI:10.1016/S0040-6090(01)01578-4.
- M. S. A. Kamel, A. Al-jumaili, M. Oelgemöller and M. V. Jacob, Inorganic nanoparticles to overcome efficiency inhibitors of organic photovoltaics: An in-depth review, Renewable Sustainable Energy Rev., 2022, 166, 112661, DOI:10.1016/j.rser.2022.112661.
- J. P. Mailoa, C. D. Bailie, E. C. Johlin, E. T. Hoke, A. J. Akey, W. H. Nguyen, M. D. McGehee and T. Buonassisi, A 2-terminal perovskite/silicon multijunction solar cell enabled by a silicon tunnel junction, Appl. Phys. Lett., 2015, 106, 121105, DOI:10.1063/1.4914179.
- T. P. White, N. Lal and K. R. Catchpole, Tandem Solar Cells Based on High-Efficiency c-Si Bottom Cells: Top Cell Requirements for >30% Efficiency, IEEE J. Photovolt., 2014, 4(1), 208–214, DOI:10.1109/JPHOTOV.2013.2283342.
- N. N. Lal, T. White and K. R. Catchpole, Optics and Light Trapping for Tandem Solar Cells on Silicon, IEEE J. Photovolt., 2014, 4(6), 1380–1386, DOI:10.1109/JPHOTOV.2014.2342491.
- C. Deibel, T. Strobel and V. Dyakonov, Role of the Charge Transfer State in Organic Donor–Acceptor Solar Cells, Adv. Mater., 2010, 22, 4097–4111, DOI:10.1002/adma.201000376.
- H. Haloui, K. Touafek and A. Khelifa, Modeling of a hybrid thermal photovoltaic (PVT) collector based on thin film organic solar cells, Electr. Power Syst. Res., 2022, 210, 108131, DOI:10.1016/j.epsr.2022.108131.
- Y. K. Kim, T. H. Lee, J. Yeop, W. J. Byun, J. H. Kim, J. Y. Kim and J. S. Lee, Hetero-tandem organic solar cells drive water electrolysis with a solar-to-hydrogen conversion efficiency up to 10, Appl. Catal., B, 2022, 309, 121237, DOI:10.1016/j.apcatb.2022.121237.
- V. M. Moorthy and V. M. Srivastava, Device Modeling of Organic Photovoltaic Cells with Traditional and Inverted Cells Using s-SWCNT:C60 as Active Layer, Nanomaterials, 2022, 12, 2844, DOI:10.3390/nano12162844.
- J. Wang, Y. Cui, Y. Xu, K. Xian, P. Bi, Z. Chen, K. Zhou, L. Ma, T. Zhang, Y. Yang, Y. Zu, H. Yao, X. Hao, L. Ye and J. Hou, A New Polymer Donor Enables Binary All-Polymer Organic Photovoltaic Cells with 18% Efficiency and Excellent Mechanical Robustness, Adv. Mater., 2022, 34, 2205009, DOI:10.1002/adma.202205009.
- T. Zhang, C. An, Y. Cui, J. Zhang, P. Bi, C. Yang, S. Zhang and J. Hou, A Universal Nonhalogenated Polymer Donor for High-Performance Organic Photovoltaic Cells, Adv. Mater., 2022, 34, 2105803, DOI:10.1002/adma.202105803.
- Z. Wang, A. Tang, H. Wang, Q. Guo, Q. Guo, X. Sun, Z. Xiao, L. Ding and E. Zhou, Organic photovoltaic cells offer ultrahigh VOC of ∼ 1.2 V under AM 1.5G light and a high efficiency of 21.2% under indoor light, Chem. Eng. J., 2023, 451(4), 1–8, DOI:10.1016/j.cej.2022.139080.
- R. Ilmi, A. Haque and M. S. Khan, High efficiency small molecule-based donor materials for organic solar cells, Org. Electron., 2018, 58, 53–62, DOI:10.1016/j.orgel.2018.03.048.
- M. Zhu, K. Meng, C. Xu, J. Zhang and G. Ni, Lateral photovoltaic effect in ITO/PEDOT:PSS/MEH-PPV:PCBM/Al organic photovoltaic cells, Org. Electron., 2020, 78, 1–5, DOI:10.1016/j.orgel.2019.105585.
- Md. H. Rahaman, B. Sang, Md. A. Hossain, B. Hoex, P. M. Santiago, V. D. Mitchell, A. Uddin and J. A. Stride, Impact of the bilayer electron transport layer in the donor acceptor bulk heterojunctions for improved inverted organic photovoltaic performance, Appl. Surf. Sci., 2023, 612, 1–12, DOI:10.1016/j.apsusc.2022.155669.
- L. Ma, Y. Cui, J. Zhang, K. Xian, Z. Chen, K. Zhou, T. Zhang, W. Wang, H. Yao, S. Zhang, X. Hao, L. Ye and J. Hou, High-Efficiency and Mechanically Robust All-Polymer Organic Photovoltaic Cells Enabled by Optimized Fibril Network Morphology, Adv. Mater., 2022, 2208926, DOI:10.1002/adma.202208926.
- M. Jahandar, A. Prasetio, C. Lee, H. Kim, A. R. Kim, J. Heo, Y. Kim, S. Kim and D. C. Lim, Highly efficient flexible organic photovoltaic modules for sustainable energy harvesting under low-light condition via suppressing voltage-drop by metal-mediated cross-linkable polymer interfacial layer, Chem. Eng. J., 2022, 448, 1–12, DOI:10.1016/j.cej.2022.137555.
- G. Bianchi, C. Carbonera, L. Ciammaruchi, N. Camaioni, N. Negarville, F. Tinti, G. Forti, A. Nitti, D. Pasini, A. Facchetti, R. M. Pankow, T. J. Marks and R. Po, An Anthradithiophene Donor Polymer for Organic Solar Cells with a Good Balance between Efficiency and Synthetic Accessibility, Sol. RRL, 2022, 6, 2200643, DOI:10.1002/solr.202200643.
- K. Y. Ryu, S. Shafian, J. Shin, Y. J. Lee, M. Lee and K. Kim, Linear polyurethane ionenes for stable interlayer of organic photovoltaics, J. Power Sources, 2022, 542, 1–6, DOI:10.1016/j.jpowsour.2022.231772.
- C. Y. Chan, Y. C. Wong, M. Y. Chan, S. H. Cheung, S. K. So and V. W. W. Yam, Hole-Transporting Spirothioxanthene Derivatives as Donor Materials for Efficient Small-Molecule-Based Organic Photovoltaic Devices, Chem. Mater., 2014, 26(22), 6585–6594, DOI:10.1021/cm5033699.
- J. Gong, J. Liang and K. Sumathy, Review on dye-sensitized solar cells (DSSCs): fundamental concepts and novel materials, Renewable Sustainable Energy Rev., 2012, 16, 5848–5860, DOI:10.1016/j.rser.2012.04.044.
- W. Zhang, Y. Xu, H. Wang, C. Xu and S. Yang, Fe3O4 nanoparticles induced magnetic field effect on efficiency enhancement of P3HT:PCBM bulk heterojunction polymer solar cells, Sol. Energy Mater. Sol. Cells, 2011, 95, 2880–2885, DOI:10.1016/j.solmat.2011.06.005.
- M. H. Rahaman, J. Holland, M. A. Hossain, L. Duan, B. Hoex, P. M. Santiago, V. D. Mitchell, A. Uddin and J. A. Stride, Increased Efficiency of Organic Solar Cells by Seeded Control of the Molecular Morphology in the Active Layer, Sol. RRL, 2022, 6, 2200184, DOI:10.1002/solr.202200184.
- L. Timotijevic, M. Vujisic and K. Stankovic, Simulation of radiation effects in ultra-thin insulating layers, Nucl. Technol. Radiat. Prot., 2013, 28, 308–315, DOI:10.2298/NTRP1303308T.
- M. Vujisić, K. Stanković, E. Dolićanin and P. Osmokrović, Radiation hardness of COTS EPROMs and E2 PROMs, Radiat. Eff. Defects Solids, 2010, 165, 362–369, DOI:10.1080/10420151003664747.
- S. K. M. Haque, J. A. Rey, Y. Umar, H. Rahman, A. Mas’ud, F. Sukki and R. Albarracín, Polymeric Materials for Conversion of Electromagnetic Waves from the Sun to Electric Power, Polymers, 2018, 10, 307, DOI:10.3390/polym10030307.
- K. Ohta, K. Tominaga, T. Ikoma, Y. Kobori and H. Yamada, Microscopic Structures, Dynamics, and Spin Configuration of the Charge Carriers in Organic Photovoltaic Solar Cells Studied by Advanced Time-Resolved Spectroscopic Methods, Langmuir, 2022, 38(24), 7365–7382, DOI:10.1021/acs.langmuir.2c00290.
- J. Nelson, Organic photovoltaic films, Curr. Opin. Solid State Mater. Sci., 2002, 6, 87–95, DOI:10.1016/S1359-0286(02)00006-2.
- D. M. Gonzalez, V. Körstgens, Y. Yao, L. Song, G. Santoro, S. V. Roth and P. M. Buschbaum, Improved Power Conversion Efficiency of P3HT:PCBM Organic Solar Cells by Strong Spin–Orbit Coupling-Induced Delayed Fluorescence, Adv. Energy Mater., 2015, 5, 1401770, DOI:10.1002/aenm.201401770.
- G. Dennler, M. C. Scharber and C. J. Brabec, Polymer-Fullerene Bulk Heterojunction Solar Cells, Adv. Mater., 2010, 21, 1323–1338, DOI:10.1002/adma.200801283.
- M. Hiramoto, M. Suezaki and M. Yokoyama, Effect of Thin Gold Interstitial-Layer on The Photovoltaic Properties of Tandem Organic Solar Cell, Chem. Lett., 1990, 19(3), 327–330, DOI:10.1246/CL.1990.327.
- H. Tian and L. Sun, 8.16 - Organic Photovoltaics and Dye-Sensitized Solar Cells, Comprehensive Inorganic Chemistry II, ed. Jan Reedijk and Kenneth Poeppelmeier, Elsevier, 2nd edn, 2013, pp. 567–605, DOI:10.1016/B978-0-08-097774-4.00809-3.
- R. O. Kesinro, A. O. Boyo, M. L. Akinyemi, M. E. Emetere and A. P. Aizebeokhai, Progress on Organic Solar Cells: A Short Review, International Conference on Energy and Sustainable Environment, IOP Conf. Ser.: Earth Environ. Sci., 2021, 665, 012036, DOI:10.1088/1755-1315/665/1/012036.
- Y. S. Li, C. H. Tsai, S. H. Kao, I. W. Wu, J. Z. Chen, C. I. Wu, C. F. Lin and I. C. Cheng, Single-layer organic–inorganic-hybrid thin-film encapsulation for organic solar cells, J. Phys. D: Appl. Phys., 2013, 46, 435502, DOI:10.1088/0022-3727/46/43/435502.
- A. Wadsworth, Z. Hamid, J. Kosco, N. Gasparini and I. McCulloch, The Bulk Heterojunction in Organic Photovoltaic, Photodetector, and Photocatalytic Applications, Adv. Mater., 2020, 32, 2001763, DOI:10.1002/adma.202001763.
- H. Kim, S. Nam, J. Jeong, S. Lee, J. Seo, H. Han and Y. Kim, Organic solar cells based on conjugated polymers: History and recent advances, Korean J. Chem. Eng., 2014, 31, 1095–1104, DOI:10.1007/s11814-014-0154-8.
- J. Lee, T. Sagawa, M. Takafuji and H. Ihara, Modeling of optimum size and shape for high photovoltaic performance of poly(3-hexylthiophene) nanopore in interdigitated bilayer organic solar cells, Org. Electron., 2016, 28, 59–66, DOI:10.1016/j.orgel.2015.09.026.
- H. Gommans, T. Aernouts, B. Verreet, P. Heremans, A. Medina, C. G. Claessens and T. Torres, Perfluorinated subphthalocyanine as a new acceptor material in a small-molecule bilayer organic solar cell, Adv. Funct. Mater., 2009, 19, 3435–3439, DOI:10.1002/adfm.200900524.
- S. Ahn, W. Jang, J. H. Park and D. H. Wang, Enhanced performance of layer-evolved bulk-heterojunction solar cells with Ag nanoparticles by sequential deposition, Org. Electron., 2015, 24, 325–329, DOI:10.1016/j.orgel.2015.05.008.
- H. Hoppe and N. S. Sariciftci, Organic solar cells: An overview, J. Mater. Res., 2004, 19, 1924–1945, DOI:10.1557/JMR.2004.0252.
- H. Shirakawa, E. J. Louis, A. G. MacDiarmid, C. K. Chiang and A. J. Heeger, Synthesis of electrically conducting organic polymers: halogen derivatives of polyacetylene,(CH)x, J. Chem. Soc., Chem. Commun., 1977, 16, 578–580, 10.1039/C39770000578.
- P. W. M. Blom, V. D. Mihailetchi, L. J. A. Koster and D. E. Markov, Device physics of polymer: fullerene bulk heterojunction solar cells, Adv. Mater., 2007, 19(12), 1551–1566, DOI:10.1002/adma.200601093.
- Y. Lee, S. Biswas, H. Choi and H. Kim, Record High Efficiency Achievement under LED Light in Low Bandgap Donor-Based Organic Solar Cell through Optimal Design, Int. J. Energy Res., 2023, 3823460, DOI:10.1155/2023/3823460.
- C. M. Amb, S. Chen, K. R. Graham, J. Subbiah, C. E. Small, F. So and J. R. Reynolds, Dithienogermole as a fused electron donor in bulk heterojunction solar cells, J. Am. Chem. Soc., 2011, 133, 10062–10065, DOI:10.1021/ja204056m.
- R. F. Service, Outlook brightens for plastic solar cells, Science, 2011, 332, 293, DOI:10.1126/science.332.6027.293.
- G. Li, R. Zhu and Y. Yang, Polymer solar cells, Nat. Photonics, 2012, 6, 153–161, DOI:10.1038/nphoton.2012.11.
- W. Ma, C. Yang, X. Gong, K. Lee and A. J. Heeger, Thermally stable, efficient polymer solar cells with nanoscale control of the interpenetrating network morphology, Adv. Funct. Mater., 2005, 15, 1617–1622, DOI:10.1002/adfm.200500211.
- J. Song and Z. Bo, Asymmetric molecular engineering in recent nonfullerene acceptors for efficient organic solar cells, Chin. Chem. Lett., 2023, 108163, DOI:10.1016/j.cclet.2023.108163.
- X. Liang, J. Wang, R. Miao, Q. Zhao, L. Huang, S. Wen and J. Tang, The evolution of small molecular acceptors for organic solar cells: Advances, challenges and prospects, Dyes Pigm., 2022, 198, 109963, DOI:10.1016/j.dyepig.2021.109963.
- K. Zhou, X. Zhou, X. Xu, C. Musumeci, C. Wang, W. Xu, X. Meng, W. Ma and O. Inganäs, π–π Stacking Distance and Phase Separation Controlled Efficiency in Stable All-Polymer Solar Cells, Polymers, 2019, 11(10), 1665, DOI:10.3390/polym11101665.
- A. G. S. Al-Azzawi, S. B. Aziz, E. M. A. Dannoun, A. Iraqi, M. M. Nofal, A. R. Murad and M. Hussein, A Mini Review on the Development of Conjugated Polymers: Steps towards the Commercialization of Organic Solar Cells, Polymers, 2023, 15(1), 164, DOI:10.3390/polym15010164.
- N. Camaioni, C. Carbonera, L. Ciammaruchi, G. Corso, J. Mwaura, R. Po and F. Tinti, Polymer Solar Cells with Active Layer Thickness Compatible with Scalable Fabrication Processes: A Meta-Analysis, Adv. Mater., 2023, 35, 2210146, DOI:10.1002/adma.202210146.
- I. G. Hill, A. Kahn, Z. G. Soos and R. A. Pascal, Charge-separation energy in films of π-conjugated organic molecules, Chem. Phys. Lett., 2000, 327, 181–188, DOI:10.1016/S0009-2614(00)00882-4.
- S. Alvarado, P. Seidler, D. Lidzey and D. Bradley, Direct determination of the exciton binding energy of conjugated polymers using a scanning tunneling microscope, Phys. Rev. Lett., 1998, 81, 1082–1085, DOI:10.1103/PhysRevLett.81.1082.
- S. Calnan, Applications of Oxide Coatings in Photovoltaic Devices, Coatings, 2014, 4, 162–202, DOI:10.3390/coatings4010162.
- N. Marjanović, M. Vujisić, K. Stanković and P. Osmokrović, Effects of heavy ion bombardment on TiO2 memristor operation, Radiat. Eff. Defects Solids, 2011, 166, 1–7, DOI:10.1080/10420150.2010.533673.
- D. Lazarevic, M. Vujisic, K. Stankovic, E. Dolicanin and P. Osmokrovic, Radiation hardness of indium oxide films in the Cooper-pair insulator state, Nucl. Technol. Radiat. Prot., 2012, 27, 40–43, DOI:10.2298/NTRP1201040L.
- J. Saleh, S. Haider, M. S. Akhtar, M. Saqib, M. Javed, S. Elshahat and G. M. Kamal, Energy Level Prediction of Organic Semiconductors for Photodetectors and Mining of a Photovoltaic Database to Search for New Building Units, Molecules, 2023, 28(3), 1240, DOI:10.3390/molecules28031240.
- F. Khan, B. D. Rezgui and J. H. Kim, Analysis of PV cell parameters of solution processed Cu-doped nickel oxide hole transporting layer-based organic-inorganic perovskite solar cells, Sol. Energy, 2020, 209, 226–234, DOI:10.1016/j.solener.2020.09.007.
- E. Raza, Z. Ahmad, F. Aziz, M. Asif, M. Q. Mehmood, J. Bhadra and N. J. Al-Thani, Design and optimization of four-terminal mechanically stacked and optically coupled silicon/perovskite tandem solar cells with over 28% efficiency, Heliyon, 2023, 9, 2, DOI:10.1016/j.heliyon.2023.e13477.
- J. C. Yu, J. A. Hong, E. D. Jung, D. B. Kim, S. M. Baek, S. Lee, S. Cho, S. S. Park, K. J. Choi and M. H. Song, Highly efficient and stable inverted perovskite solar cell employing PEDOT: GO composite layer as a hole transport layer, Sci. Rep., 2018, 8, 1070, DOI:10.1038/s41598-018-19612-7.
- O. Ostroverkhova, Organic Optoelectronic Materials: Mechanisms and Applications, Chem. Rev., 2016, 116(22), 13279–13412, DOI:10.1021/acs.chemrev.6b00127.
- M. L. Tang, T. Okamoto and Z. Bao, High-Performance Organic Semiconductors: Asymmetric Linear Acenes Containing Sulphur, J. Am. Chem. Soc., 2006, 128(50), 16002–16003, DOI:10.1021/ja066824j.
- K. Bagchi and M. D. Ediger, Controlling Structure and Properties of Vapor-Deposited Glasses of Organic Semiconductors: Recent Advances and Challenges, J. Phys. Chem. Lett., 2020, 11(17), 6935–6945, DOI:10.1021/acs.jpclett.0c01682.
- Y. Deng, Y. Chen, X. Zhang, H. Tian, C. Bao, D. Yan, Y. Geng and F. Wan, Donor–Acceptor Conjugated Polymers with Dithienocarbazoles as Donor Units: Effect of Structure on Semiconducting Properties, Macromolecules, 2012, 45(21), 8621–8627, DOI:10.1021/ma301864f.
- S. Günes, H. Neugebauer and N. S. Sariciftci, Conjugated Polymer-Based Organic Solar Cells, Chem. Rev., 2007, 107(4), 1324–1338, DOI:10.1021/cr050149z.
- A. Phengdaam, S. Nootchanat, R. Ishikawa, C. Lertvachirapaiboon, K. Shinbo, K. Kato, S. Ekgasit and A. Baba, Improvement of organic solar cell performance by multiple plasmonic excitations using mixed-silver nanoprisms, J. Sci.: Adv. Mater. Devices, 2021, 6(2), 264–270, DOI:10.1016/j.jsamd.2021.02.007.
- Z. Jiang, B. Gholamkhass and P. Servati, Effects of interlayer properties on the performance of tandem organic solar cells with low and high band gap polymers, J. Mater. Res., 2019, 34, 2407–2415, DOI:10.1557/jmr.2019.168.
- J. S. Park, G. U. Kim, S. Lee, J. W. Lee, S. Li, J. Y. Lee and B. J. Kim, Material Design and Device Fabrication Strategies for Stretchable Organic Solar Cells, Adv. Mater., 2022, 34, 2201623, DOI:10.1002/adma.202201623.
- J. H. Kim, S. Wood, J. B. Park, J. Wade, M. Song, S. C. Yoon, I. H. Jung, J. S. Kim and D. H. Hwang, Optimization and Analysis of Conjugated Polymer Side Chains for High-Performance Organic Photovoltaic Cells, Adv. Funct. Mater., 2016, 26, 1517–1525, DOI:10.1002/adfm.201504093.
- M. Wang, P. Baek, A. Akbarinejad, D. Barker and J. Travas-Sejdic, Conjugated polymers and composites for stretchable organic electronics, J. Mater. Chem. C, 2019, 7, 5534–5552, 10.1039/c9tc00709a.
- Q. Lu, Z. Yang, X. Meng, Y. Yue, M. A. Ahmad, W. Zhang, S. Zhang, Y. Zhang, Z. Liu and W. A. Chen, A Review on Encapsulation Technology from Organic Light Emitting Diodes to Organic and Perovskite Solar Cells, Adv. Funct. Mater., 2021, 31, 2100151, DOI:10.1002/adfm.202100151.
- A. Uddin, M. B. Upama, H. Yi and L. Duan, Encapsulation of Organic and Perovskite Solar Cells: A Review, Coatings, 2019, 9(2), 65, DOI:10.3390/coatings9020065.
- M. S. A. Kamel, M. Oelgemöller and M. V. Jacob, Sustainable plasma polymer encapsulation materials for organic solar cells, J. Mater. Chem. A, 2022, 10, 4683–4694, 10.1039/D1TA10608B.
- National Renewable Energy Laboratory, Best research-cell efficiency charts, online source at https://www.nrel.gov/pv/cell-efficiency.html, last accessed on Feb 25, 2023.
- Y. Li, W. Huang, D. Zhao, L. Wang, Z. Jiao, Q. Huang, P. Wang, M. Sun and G. Yuan, Recent Progress in Organic Solar Cells: A Review on Materials from Acceptor to Donor, Molecules, 2022, 27(6), 1800, DOI:10.3390/molecules27061800.
- H. Gao, C. Han, X. Wan and Y. Chen, Recent progress in non-fused ring electron acceptors for high performance organic solar cells, Ind. Chem. Mater.Industrial Chemistry & Materials, 2023, 1, 60–78, 10.1039/D2IM00037G.
- J. Song, L. Zhu, C. Li, J. Xu, H. Wu, X. Zhang, Y. Zhang, Z. Tang, F. Liu and Y. Sun, High-efficiency organic solar cells with low voltage loss induced by solvent additive strategy, Matter, 2021, 4, 2542–2552, DOI:10.1016/j.matt.2021.06.010.
- K. Jin, Z. Xiao and L. Ding, D18, an eximious solar polymer, J. Semicond., 2021, 42(1), 1–4, DOI:10.1088/1674-4926/42/1/010502.
- C. Li, J. Zhou, J. Song, J. Xu, H. Zhang, X. Zhang, J. Guo, L. Zhu, D. Wei, G. Han, J. Min, Y. Zhang, Z. Xie, Y. Yi, H. Yan, F. Gao, F. Liu and Y. Sun, Non-fullerene acceptors with branched side chains and improved molecular packing to exceed 18% efficiency in organic solar cells, Nat. Energy, 2021, 6, 605–613, DOI:10.1038/s41560-021-00820-x.
- Q. Liu, Y. Jiang, K. Jin, J. Qin, J. Xu, W. Li, J. Xiong, J. Liu, Z. Xiao, K. Sun, S. Yang, X. Zhang and L. Ding, 18% Efficiency organic solar cells, Sci. Bull., 2020, 65, 272–275, DOI:10.1016/j.scib.2020.01.001.
- Y. Cui, H. Yao, J. Zhang, K. Xian, T. Zhang, L. Hong, Y. Wang, Y. Xu, K. Ma, C. An, C. He, Z. Wei, F. Gao and J. Hou, Single-Junction Organic Photovoltaic Cells with Approaching 18% Efficiency, Adv. Mater., 2020, 32, 1908205, DOI:10.1002/adma.201908205.
- Z. Zhang, Y. Li, G. Cai, Y. Zhang, X. Lu and Y. Lin, Selenium heterocyclic electron acceptor with small urbach energy for as-cast high-performance organic solar cells, J. Am. Chem. Soc., 2020, 142, 18741–18745, DOI:10.1021/jacs.0c08557.
- G. Chai, Y. Chang, J. Zhang, X. Xu, L. Yu, X. Zou, X. Li, Y. Chen, S. Luo, B. Liu, F. Bai, Z. Luo, H. Yu, J. Liang, T. Liu, K. S. Wong, H. Zhou, Q. Peng and H. Yan, Fine-tuning of side-chain orientations on nonfullerene acceptors enables organic solar cells with 17.7% efficiency, Energy Environ. Sci., 2021, 14, 3469–3479, 10.1039/D0EE03506H.
- H. N. Tran, S. Park, F. T. A. Wibowo, N. V. Krishna, J. H. Kang, J. H. Seo, H. Nguyen-Phu, S. Y. Jang and S. Cho, 17% Non-fullerene organic solar cells with annealing-free aqueous MoOx, Adv. Sci., 2020, 7, 2002395, DOI:10.1002/advs.20200239.
- R. Ma, T. Liu, Z. Luo, Q. Guo, Y. Xiao, Y. Chen, X. Li, S. Luo, X. Lu, M. Zhang, Y. Li and H. Yan, Improving open-circuit voltage by a chlorinated polymer donor endows binary organic solar cells efficiencies over 17%, Sci. China: Chem., 2020, 63, 325–330, DOI:10.1007/s11426-019-9669-3.
- J. Xiong, K. Jin, Y. Jiang, J. Qin, T. Wang, J. Liu, Q. Liu, H. Peng, X. Li, A. Sun, X. Meng, L. Zhang, L. Liu, W. Li, Z. Fang, X. Jia, Z. Xiao, Y. Feng, X. Zhang, K. Sun, S. Yang, S. Shi and L. Ding, Thiolactone copolymer donor gifts organic solar cells a 16.72% efficiency, Sci. Bull., 2019, 64, 1573–1576, DOI:10.1016/j.scib.2019.10.002.
- J. Yuan, Y. Zhang, L. Zhou, G. Zhang, H. L. Yip, T. K. Lau, X. Lu, C. Zhu, H. Peng, P. A. Johnson, M. Leclerc, Y. Cao, J. Ulanski, Y. Li and Y. Zou, Single-junction organic solar cell with over 15% efficiency using fused-ring acceptor with electron-deficient core, Joule, 2019, 3, 1140–1151, DOI:10.1016/j.joule.2019.01.004.
- X. Wang, H. Lu, Y. Liu, A. Zhang, N. Yu, H. Wang, S. Li, Y. Zhou, X. Xu, Z. Tang and Z. Bo, Simple Nonfused Ring Electron Acceptors with 3D Network Packing Structure Boosting the Efficiency of Organic Solar Cells to 15.44%, Adv. Energy Mater., 2021, 11, 2102591, DOI:10.1002/aenm.202102591.
- H. Yu, S. Luo, R. Sun, I. Angunawela, Z. Qi, Z. Peng, W. Zhou, H. Han, R. Wei, M. Pan, A. M. H. Cheung, D. Zhao, J. Zhang, H. Ade, J. Min and H. Yan, A difluoro-monobromo end group enables high-performance polymer acceptor and efficient all-polymer solar cells processable with green solvent under ambient condition, Adv. Funct. Mater., 2021, 31, 2100791, DOI:10.1002/adfm.202100791.
- L. Ma, S. Zhang, J. Zhu, J. Wang, J. Ren, J. Zhang and J. Hou, Completely non-fused electron acceptor with 3D-interpenetrated crystalline structure enables efficient and stable organic solar cell, Nat. Commun., 2021, 12, 5093, DOI:10.1038/s41467-021-25394-w.
- Y. Cui, H. Yao, L. Hong, T. Zhang, Y. Xu, K. Xian, B. Gao, J. Qin, J. Zhang, Z. Wei and J. Hou, Achieving over 15% efficiency in organic photovoltaic cells via copolymer design, Adv. Mater., 2019, 31, 1808356, DOI:10.1002/adma.201808356.
- Z. Luo, T. Liu, R. Ma, Y. Xiao, L. Zhan, G. Zhang, H. Sun, F. Ni, G. Chai, J. Wang, C. Zhong, Y. Zou, X. Guo, X. Lu, H. Chen, H. Yan and C. Yang, Precisely controlling the position of bromine on the end group enables well-regular polymer acceptors for all-polymer solar cells with efficiencies over 15, Adv. Mater., 2020, 32, 2005942, DOI:10.1002/adma.202005942.
- X. Zhang, C. Li, L. Qin, H. Chen, J. Yu, Y. Wei, X. Liu, J. Zhang, Z. Wei, F. Gao, Q. Peng and H. Huang, Side-Chain Engineering for Enhancing the Molecular Rigidity and Photovoltaic Performance of Noncovalently Fused-Ring Electron Acceptors, Angew. Chem., Int. Ed., 2021, 60, 17720, DOI:10.1002/anie.202106753.
- X. Zhang, L. Qin, J. Yu, Y. Li, Y. Wei, X. Liu, X. Lu, F. Gao and H. Huang, High-Performance Noncovalently Fused-Ring Electron Acceptors for Organic Solar Cells Enabled by Noncovalent Intramolecular Interactions and End-Group Engineering, Angew. Chem., Int. Ed., 2021, 60, 12475, DOI:10.1002/anie.202100390.
- S. Zhang, Y. Qin, J. Zhu and J. Hou, Over 14% efficiency in polymer solar cells enabled by a chlorinated polymer donor, Adv. Mater., 2018, 30, 1800868, DOI:10.1002/adma.201800868.
- T. Jia, J. Zhang, W. Zhong, Y. Liang, K. Zhang, S. Dong, L. Ying, F. Liu, X. Wang, F. Huang and Y. Cao, 14.4% efficiency all-polymer solar cell with broad absorption and low energy loss enabled by a novel polymer acceptor, Nano Energy, 2020, 72, 104718, DOI:10.1016/j.nanoen.2020.104718.
- H. Sun, H. Yu, Y. Shi, J. Yu, Z. Peng, X. Zhang, B. Liu, J. Wang, R. Singh, J. Lee, Y. Li, Z. Wei, Q. Liao, Z. Kan, L. Ye, H. Yan, F. Gao and X. Guo, A narrow-bandgap n-type polymer with an acceptor–acceptor backbone enabling efficient all-polymer solar cells, Adv. Mater., 2020, 32, 2004183, DOI:10.1002/adma.202004183.
- X. Wang, H. Lu, A. Zhang, N. Yu, G. Ran, Z. Bi, X. Yu, X. Xu, Y. Liu, Z. Tang, W. Zhang, W. Ma and Z. Bo, Molecular-Shape-Controlled Nonfused Ring Electron Acceptors for High-Performance Organic Solar Cells with Tunable Phase Morphology, ACS Appl. Mater. Interfaces, 2022, 14(25), 28807–28815, DOI:10.1021/acsami.2c04530.
- D. Luo, Z. Jiang, C. Shan, L. Li, C. Duan, Q. Liu, Z. Wang, K. Wang, B. Xu and A. K. K. Kyaw, Simultaneous Tuning of Alkyl Chains and End Groups in Non-fused Ring Electron Acceptors for Efficient and Stable Organic Solar Cells, ACS Appl. Mater. Interfaces, 2022, 14(21), 24374–24385, DOI:10.1021/acsami.2c03723.
- H. Yu, Z. Qi, J. Yu, Y. Xiao, R. Sun, Z. Luo, A. M. H. Cheung, J. Zhang, H. Sun, W. Zhou, S. Chen, X. Guo, X. Lu, F. Gao, J. Min and H. Yan, Fluorinated end group enables high-performance all-polymer solar cells with near-infrared absorption and enhanced device efficiency over 14, Adv. Energy Mater., 2021, 11, 2003171, DOI:10.1002/aenm.202003171.
- X. Liu, Y. Wei, X. Zhang, L. Qin, Z. Wei and H. Huang, An A-D-A′-D-A type unfused nonfullerene acceptor for organic solar cells with approaching 14% efficiency, Sci. China: Chem., 2021, 64, 228–231, DOI:10.1007/s11426-020-9868-8.
- C. Li, X. Zhang, N. Yu, X. Gu, L. Qin, Y. Wei, X. Liu, J. Zhang, Z. Wei, Z. Tang, Q. Shi and H. Huang, Simple Nonfused-Ring Electron Acceptors with Noncovalently Conformational Locks for Low-Cost and High-Performance Organic Solar Cells Enabled by End-Group Engineering, Adv. Funct. Mater., 2022, 32, 2108861, DOI:10.1002/adfm.202108861.
- M. Chang, L. Meng, Y. Wang, X. Ke, Y. Q. Q. Yi, N. Zheng, W. Zheng, Z. Xie, M. Zhang, Y. Yi, H. Zhang, X. Wan, C. Li and Y. Chen, Achieving an Efficient and Stable Morphology in Organic Solar Cells Via Fine-Tuning the Side Chains of Small-Molecule Acceptors, Chem. Mater., 2020, 32(6), 2593–2604, DOI:10.1021/acs.chemmater.0c00097.
- W. Wang, Q. Wu, R. Sun, J. Guo, Y. Wu, M. Shi, W. Yang, H. Li and J. Min, Controlling molecular mass of low-band-gap polymer acceptors for high-performance all-polymer solar cells, Joule, 2020, 4, 1070–1086, DOI:10.1016/j.joule.2020.03.019.
- J. Yuan, T. Huang, P. Cheng, Y. Zou, H. Zhang, J. L. Yang, S. Y. Chang, Z. Zhang, W. Huang, R. Wang, D. Meng, F. Gao and Y. Yang, Enabling low voltage losses and high photocurrent in fullerene-free organic photovoltaics, Nat. Commun., 2019, 10, 570, DOI:10.1038/s41467-019-08386-9.
- D. Luo, L. Li, Y. Shi, J. Zhang, K. Wang, X. Guo and A. K. K. Kyaw, Electron-deficient diketone unit engineering for non-fused ring acceptors enabling over 13% efficiency in organic solar cells, J. Mater. Chem. A, 2021, 9, 14948–14957, 10.1039/D1TA03643B.
- J. L. Wang, K. K. Liu, L. Hong, G. Y. Ge, C. Zhang and J. Hou, Selenopheno[3,2-b]thiophene-based narrow-bandgap nonfullerene acceptor enabling 13.3% efficiency for organic solar cells with thickness-insensitive feature, ACS Energy Lett., 2018, 3, 2967–2976, DOI:10.1021/acsenergylett.8b01808.
- Y. Zhou, M. Li, N. Yu, S. Shen, J. Song, Z. Ma and Z. Bo, Simple Tricyclic-Based A-π-D-π-A-Type Nonfullerene Acceptors for High-Efficiency Organic Solar Cells, ACS Appl. Mater. Interfaces, 2022, 14(4), 6039–6047, DOI:10.1021/acsami.1c22520.
- J. Zhu, Y. Xiao, J. Wang, K. Liu, H. Jiang, Y. Lin, X. Lu and X. Zhan, Alkoxy-induced near-infrared sensitive electron acceptor for high-performance organic solar cells, Chem. Mater., 2018, 30, 4150–4156, DOI:10.1021/acs.chemmater.8b01677.
- W. Zhao, S. Li, H. Yao, S. Zhang, Y. Zhang, B. Yang and J. Hou, Molecular optimization enables over 13% efficiency in organic solar cells, J. Am. Chem. Soc., 2017, 139, 7148–7151, DOI:10.1021/jacs.7b02677.
- H. Lu, X. Wang, S. Li, D. Li, N. Yu, Z. Tang, Y. Liu, X. Xu and Z. Bo, Diphenylamine Substituted High-performance Fully Nonfused Ring Electron Acceptors: The Effect of Isomerism, Chem. Eng. J., 2022, 435(2), 1–7, DOI:10.1016/j.cej.2022.134987.
- X. Xu, T. Yu, Z. Bi, W. Ma, Y. Li and Q. Peng, Realizing over 13% efficiency in green-solvent-processed nonfullerene organic solar cells enabled by 1,3,4-thiadiazole-based wide-bandgap copolymers, Adv. Mater., 2018, 30, 1703973, DOI:10.1002/adma.201703973.
- T.-J. Wen, Z.-X. Liu, Z. Chen, J. Zhou, Z. Shen, Y. Xiao, X. Lu, Z. Xie, H. Zhu, C.-Z. Li and H. Chen, Simple Non-Fused Electron Acceptors Leading to Efficient Organic Photovoltaics, Angew. Chem., Int. Ed., 2021, 60, 12964, DOI:10.1002/anie.202101867.
- Z. Wu, Y. Chen, L. Zhang, D. Yuan, R. Qiu, S. Deng, H. Liu, Z. Zhangab and J. Chen, A ligand-free direct heteroarylation approach for benzodithiophenedione-based simple small molecular acceptors toward high efficiency polymer solar cells, J. Mater. Chem. A, 2021, 9, 3314–3321, 10.1039/D0TA12288B.
- Y. Lin, F. Zhao, S. K. K. Prasad, J. D. Chen, W. Cai, Q. Zhang, K. Chen, Y. Wu, W. Ma, F. Gao, J. X. Tang, C. Wang, W. You, J. M. Hodgkiss and X. Zhan, Balanced partnership between donor and acceptor components in nonfullerene organic solar cells with >12% efficiency, Adv. Mater., 2018, 30, 1706363, DOI:10.1002/adma.201706363.
- J. Huang, S. Li, J. Qin, L. Xu, X. Zhu and L. M. Yang, Facile Modification of a Noncovalently Fused-Ring Electron Acceptor Enables Efficient Organic Solar Cells, ACS Appl. Mater. Interfaces, 2021, 13(38), 45806–45814, DOI:10.1021/acsami.1c11412.
- X. Wang, H. Lu, J. Zhou, X. Xu, C. Zhang, H. Huang, J. Song, Y. Liu, X. Xu, Z. Xie, Z. Tang and Z. Bo, High-Performance Simple Nonfused Ring Electron Acceptors with Diphenylamino Flanking Groups, ACS Appl. Mater. Interfaces, 2021, 13(33), 39652–39659, DOI:10.1021/acsami.1c09597.
- X. Ding, X. Chen, Y. Xu, Z. Ni, T. He, H. Qiu, C. Z. Li and Q. Zhang, A selenophene-containing near-infrared unfused acceptor for efficient organic solar cells, Chem. Eng. J., 2022, 429, 1–8, DOI:10.1016/j.cej.2021.132298.
- J. Cao, S. Qu, L. Yang, H. Wang, F. Du, J. Yu and W. Tang, Asymmetric simple unfused acceptor enabling over 12% efficiency organic solar cells, Chem. Eng. J., 2021, 412, 1–8, DOI:10.1016/j.cej.2021.128770.
- D. Luo, Z. Jiang, W. Yang, X. Guo, X. Li, E. Zhou, G. Li, L. Li, C. Duan, C. Shan, Z. Wang, Y. Li, B. Xu and A. K. K. Kyaw, Dual-functional ambipolar non-fused ring electron acceptor as third component and designing similar molecular structure between two acceptors for high-performance ternary organic solar cells, Nano Energy, 2022, 98, 1–13, DOI:10.1016/j.nanoen.2022.107186.
- X. Zheng, W. Liu, H. Lu, N. Yu, Y. Wang, H. Huang, S. Li, X. Wang, H. Wang, Y. Liu, X. Xu, Z. Tang and Z. Bo, A simple high-performance fully nonfused ring electron acceptor with a planar molecular backbone, Chem. Eng. J., 2022, 444, 136472, DOI:10.1016/j.cej.2022.136472.
- J. Li, H. Li, L. Ma, Y. Xu, Y. Cui, J. Wang, J. Ren, J. Zhu, S. Zhang and J. Hou, Influence of Large Steric Hindrance Substituent Position on Conformation and Charge Transfer Process for Non-Fused Ring Acceptors, Small Methods, 2022, 6, 2200007, DOI:10.1002/smtd.202200007.
- S. Li, L. Ye, W. Zhao, S. Zhang, S. Mukherjee, H. Ade and J. Hou, Energy-level modulation of small-molecule electron acceptors to achieve over 12% efficiency in polymer solar cells, Adv. Mater., 2016, 28, 9423–9429, DOI:10.1002/adma.201602776.
- Y. Zhou, M. Li, H. Lu, H. Jin, X. Wang, Y. Zhang, S. Shen, Z. Ma, J. Song and Z. Bo, High-Efficiency Organic Solar Cells Based on a Low-Cost Fully Non-Fused Electron Acceptor, Adv. Funct. Mater., 2021, 31, 2101742, DOI:10.1002/adfm.202101742.
- T. Li, S. Dai, Z. Ke, L. Yang, J. Wang, C. Yan, W. Ma and X. Zhan, Fused tris(thienothiophene)-based electron acceptor with strong near-infrared absorption for high-performance as-cast solar cells, Adv. Mater., 2018, 30, 1705969, DOI:10.1002/adma.201705969.
- L. Zhu, W. Zhong, C. Qiu, B. Lyu, Z. Zhou, M. Zhang, J. Song, J. Xu, J. Wang, J. Ali, W. Feng, Z. Shi, X. Gu, L. Ying, Y. Zhang and F. Liu, Aggregation-induced multilength scaled morphology enabling 11.76% efficiency in all-polymer solar cells using printing fabrication, Adv. Mater., 2019, 31, 1902899, DOI:10.1002/adma.201902899.
- J. Zhao, Y. Li, G. Yang, K. Jiang, H. Lin, H. Ade, W. Ma and H. Yan, Efficient organic solar cells processed from hydrocarbon solvents, Nat. Energy, 2016, 1, 15027, DOI:10.1038/nenergy.2015.27.
- L. Xue, Y. Yang, J. Xu, C. Zhang, H. Bin, Z. G. Zhang, B. Qiu, X. Li, C. Sun, L. Gao, J. Yao, X. Chen, Y. Yang, M. Xiao and Y. Li, Side chain engineering on medium bandgap copolymers to suppress triplet formation for high-efficiency polymer solar cells, Adv. Mater., 2017, 29, 1703344, DOI:10.1002/adma.201703344.
- P. Bi, S. Zhang, J. Ren, Z. Chen, Z. Zheng, Y. Cui, J. Wang, S. Wang, T. Zhang, J. Li, Y. Xu, J. Qin, C. An, W. Ma, X. Hao and J. Hou, A High-Performance Nonfused Wide-Bandgap Acceptor for Versatile Photovoltaic Applications, Adv. Mater., 2022, 34, 2108090, DOI:10.1002/adma.202108090.
- S. Ma, Q. Huang, Y. Liang, H. Tang, Y. Chen, J. Zhang, K. Zhang, F. Huang and Y. Cao, Non-fullerene electron acceptors with benzotrithiophene with π-extension terminal groups for the development of high-efficiency organic solar cells, J. Mater. Chem. C, 2021, 9, 13896–13903, 10.1039/D1TC03259C.
- X. Zhang, Y. Wei, X. Liu, L. Qin, N. Yu, Z. Tang, Z. Wei, Q. Shi, A. Peng and H. Huang, Enhancing Photovoltaic Performances of Naphthalene-Based Unfused-Ring Electron Acceptors upon Regioisomerization, Sol. RRL, 2021, 5, 2100094, DOI:10.1002/solr.202100094.
- Y. Wang, Z. Liu, X. Cui, C. Wang, H. Lu, Y. Liu, Z. Fei, Z. Ma and Z. Bo, Small molecule acceptors with a ladder-like core for high-performance organic solar cells with low non-radiative energy losses, J. Mater. Chem. A, 2020, 8, 12495–12501, 10.1039/D0TA03683H.
- W. Zhao, D. Qian, S. Zhang, S. Li, O. Inganäs, F. Gao and J. Hou, Fullerene-free polymer solar cells with over 11% efficiency and excellent thermal stability, Adv. Mater., 2016, 28, 4734–4739, DOI:10.1002/adma.201600281.
- Y. Wang, S. Liu, H. Gao, L. Wang, W. Wang, Y. Zhou, B. Zhao, H. Wu and C. Gao, Multiple chlorinations to improve the performance of unfused electron-acceptor based organic photovoltaic cells, Surf. Interfaces, 2022, 32, 102185, DOI:10.1016/j.surfin.2022.102185.
- M. Chang, Y. Zhang, B. S. Lu, D. Sui, F. Wang, J. Wang, Y. Yang and B. Kan, The design of quinoxaline based unfused non-fullerene acceptors for high performance and stable organic solar cells, Chem. Eng. J., 2022, 427, 131473, DOI:10.1016/j.cej.2021.131473.
- Y. Liu, J. Zhao, Z. Li, C. Mu, W. Ma, H. Hu, K. Jiang, H. Lin, H. Ade and H. Yan, Aggregation and morphology control enables multiple cases of high-efficiency polymer solar cells, Nat. Commun., 2014, 5, 5293, DOI:10.1038/ncomms6293.
- C. Wang, B. Wang, Y. Wu, S. Liang, L. Yuan, D. Xia, C. Zhao, F. Liu and W. Li, Naphthobistriazole based non-fused electron acceptors for organic solar cells, J. Mater. Chem. C, 2022, 10, 8070–8076, 10.1039/D2TC01077A.
- S. He, Z. Lin, F. Du, X. Wang, Y. Liu and W. Tang, Simple unfused acceptors with optimal naphthalene isomerization enabling 10.72% as-cast organic solar cells, Chem. Eng. J., 2022, 441, 135973, DOI:10.1016/j.cej.2022.135973.
- J. Zhao, X. Xu, L. Yu, R. Li, Y. Li and Q. Peng, Highly Efficient Non-Fused-Ring Electron Acceptors Enabled by the Conformational Lock and Structural Isomerization Effects, ACS Appl. Mater. Interfaces, 2021, 13(21), 25214–25223, DOI:10.1021/acsami.1c06299.
- R. Zheng, Q. Guo, D. Hao, C. Zhang, W. Xue, H. Huang, C. Li, W. Ma and Z. Bo, Naphthalene core-based noncovalently fused-ring electron acceptors: effects of linkage positions on photovoltaic performances, J. Mater. Chem. C, 2019, 7, 15141–15147, 10.1039/C9TC05013B.
- X. Zhou, S. Pang, B. Wu, J. Zhou, H. Tang, K. Lin, Z. Xie, C. Duan, F. Huang and Y. Cao, Noncovalent Interactions Induced by Fluorination of the Central Core Improve the Photovoltaic Performance of A-D-A′-D-A-Type Nonfused Ring Acceptors, ACS Appl. Energy Mater., 2022, 5(6), 7710–7718, DOI:10.1021/acsaem.2c01179.
- S. Ye, S. Chen, S. Li, Y. Pan, X. Xia, W. Fu, L. Zuo, X. Lu, M. Shi and H. Chen, Synergistic Effects of Chlorination and Branched Alkyl Side Chain on the Photovoltaic Properties of Simple Non-Fullerene Acceptors with Quinoxaline as the Core, ChemSusChem, 2021, 14, 3599, DOI:10.1002/cssc.202100689.
- S. Pang, X. Zhou, S. Zhang, H. Tang, S. Dhakal, X. Gu, C. Duan, F. Huang and Y. Cao, Nonfused Nonfullerene Acceptors with an A–D–A′–D–A Framework and a Benzothiadiazole Core for High-Performance Organic Solar Cells, ACS Appl. Mater. Interfaces, 2020, 12(14), 16531–16540, DOI:10.1021/acsami.0c01850.
- J. Gao, Y. Li, S. Li, X. Xia, X. Lu, M. Shi and H. Chen, Non-fullerene acceptors with nitrogen-containing six-membered heterocycle cores for the applications in organic solar cells, Sol. Energy Mater. Sol. Cells, 2021, 225, 111046, DOI:10.1016/j.solmat.2021.111046.
- Y. Wang, S. Liu, H. Gao, L. Wang, W. Wang, B. Zhao, H. Wu and C. Gao, Synergistic halogenation of backbone and end group for high-performance non-fused acceptors based organic solar cells, Dyes Pigm., 2022, 200, 110178, DOI:10.1016/j.dyepig.2022.110178.
- T. J. Wen, J. Xiang, N. Jain, Z. X. Liu, Z. Chen, X. Xia, X. Lu, H. Zhu, F. Gao and C. Z. Li, Non-fused medium bandgap electron acceptors for efficient organic photovoltaics, J. Energy Chem., 2022, 70, 576–582, DOI:10.1016/j.jechem.2022.03.030.
- S. Z. Geng, W. T. Yang, J. Gao, S. X. Li, M. M. Shi, T. K. Lau, X. H. Lu, C. Z. Li and H. Z. Chen, Non-fullerene Acceptors with a Thieno[3,4-c]pyrrole-4,6-dione (TPD) Core for Efficient Organic Solar Cells, Chin. J. Polym. Sci., 2019, 37, 1005–1014, DOI:10.1007/s10118-019-2309-x.
- J. D. Chen, C. Cui, Y. Q. Li, L. Zhou, Q. D. Ou, C. Li, Y. Li and J. X. Tang, Single-junction polymer solar cells exceeding 10% power conversion efficiency, Adv. Mater., 2015, 27, 1035–1041, DOI:10.1002/adma.201404535.
- J. Zhong, Y. Cui, P. Zhu, M. Zhang, W. Xie, H. Liu, Q. Xie, F. Liu, X. Liao and Y. Chen, Nonfused Ring Electron Acceptors for Efficient Organic Solar Cells Enabled by Multiple Intramolecular Conformational Locks, ACS Appl. Energy Mater., 2022, 5(4), 5136–5145, DOI:10.1021/acsaem.2c00475.
- F. Du, H. Wang, Z. Zhang, L. Yang, J. Cao, J. Yu and W. Tang, An unfused-ring acceptor with high side-chain economy enabling 11.17% as-cast organic solar cells, Mater. Horiz., 2021, 8, 1008–1016, 10.1039/D0MH01585G.
- R. Lv, S. Geng, S. Li, F. Wu, Y. Li, T. R. Andersen, Y. Li, X. Lu, M. Shi and H. Chen, Influences of Quinoid Structures on Stability and Photovoltaic Performance of Nonfullerene Acceptors, Sol. RRL, 2020, 4, 2000286, DOI:10.1002/solr.202000286.
- Z. Liang, X. Cheng, Y. Jiang, J. Yu, X. Xu, Z. Peng, L. Bu, Y. Zhang, Z. Tang, M. Li, L. Ye and Y. Geng, P3HT-Based Organic Solar Cells with a Photoresponse to 1000 nm Enabled by Narrow Band Gap Nonfullerene Acceptors with High HOMO Levels, ACS Appl. Mater. Interfaces, 2021, 13(51), 61487–61495, DOI:10.1021/acsami.1c21089.
- J. Zhu, C. Yang, L. Ma, T. Zhang, S. Li, S. Zhang, H. Fan and J. Hou, Terthiophene based non-fused electron acceptors for efficient organic solar cells, Org. Electron., 2022, 105, 106512, DOI:10.1016/j.orgel.2022.106512.
- J. Guo, W. Tang, Y. Zhang, C. Qian, J. Wang, H. Tan and W. Zhu, Simple non-fused small-molecule acceptors with bithiazole core: synthesis, crystallinity and photovoltaic properties, Mater. Adv., 2022, 3, 554–561, 10.1039/D1MA00954K.
- C. Yang, D. Liu, M. Bates, M. C. Barr and R. R. Lunt, How to Accurately Report Transparent Solar Cells, Joule, 2019, 3(Issue 8), 1803–1809, DOI:10.1016/j.joule.2019.06.005.
- I. Burgués-Ceballos, L. Lucera, P. Tiwana, K. Ocytko, L. W. Tan, S. Kowalski, J. Snow, A. Pron, H. Bürckstümmer, N. Blouin and G. Morse, Transparent organic photovoltaics: A strategic niche to advance commercialization, Joule, 2021, 5(Issue 9), 2261–2272, DOI:10.1016/j.joule.2021.07.004.
- X. Liu, Z. Zhong, R. Zhu, J. Yu and G. Li, Aperiodic band-pass electrode enables record-performance transparent organic photovoltaics, Joule, 2022, 6(Issue 8), 1918–1930, DOI:10.1016/j.joule.2022.06.009.
- A. T. Hamada, O. Z. Sharaf and M. F. Orhan, A novel photovoltaic/thermal (PV/T) solar collector based on a multi-functional nano-encapsulated phase-change material (nano-ePCM) dispersion, Energy Convers. Manage., 2023, 280, 116797, DOI:10.1016/j.enconman.2023.116797.
- N. Kant and P. Singh, Review of next generation photovoltaic solar cell technology and comparative materialistic development, Mater. Today: Proc., 2022, 56(6), 3460–3470, DOI:10.1016/j.matpr.2021.11.116.
- J. S. Cho, W. Jang, K. H. Park and D. H. Wang, Amorphous metal thin film electrode for mechanically robust charge collection of organic photovoltaics, J. Alloys Compd., 2023, 944, 169219, DOI:10.1016/j.jallcom.2023.169219.
- X. Qian, L. She, Z. Li, X. Kang and L. Ying, High-performance indoor organic photovoltaics enabled by screening multiple cases of electron acceptors, Org. Electron., 2023, 113, 106721, DOI:10.1016/j.orgel.2022.106721.
- M. Sandrini, J. C. Gemelli, M. S. Gibin, V. S. Zanuto, R. F. Muniz, F. S. de Vicente and M. P. Belançon, Synthesis and properties of Cerium-doped organic/silica xerogels: A potential UV filter for photovoltaic panels, J. Non-Cryst. Solids, 2023, 600, 122033, DOI:10.1016/j.jnoncrysol.2022.122033.
- Z. Golshani, F. Arjmand, S. Maghsoudi and S. M. A. Hosseini, Fe2O3–NiO doped carbon counter electrode for high-performance and long-term stable photovoltaic perovskite solar cells, J. Mater. Res. Technol., 2023, 23, 2612–2625, DOI:10.1016/j.jmrt.2023.01.178.
|
This journal is © The Royal Society of Chemistry 2023 |
Click here to see how this site uses Cookies. View our privacy policy here.