DOI:
10.1039/D3RA02054A
(Paper)
RSC Adv., 2023,
13, 26690-26699
A green and efficient two-step enzymatic esterification-hydrolysis method for enrichment of c9,t11-CLA isomer based on a three-liquid-phase system†
Received
29th March 2023
, Accepted 29th August 2023
First published on 6th September 2023
Abstract
A novel two-step enzymatic esterification-hydrolysis method that generates high-purity conjugated linoleic acid (CLA) isomers was developed. CLA was first partially purified by enzymatic esterification and then further purified by efficient, selective enzymatic hydrolysis in a three-liquid-phase system (TLPS). Compared with traditional two-step selective enzymatic esterification, this novel method produced highly pure cis-9, trans-11 (c9,t11)-CLA (96%) with high conversion (approx. 36%) and avoided complicated rehydrolysis and reesterification steps. The catalytic efficiency and selectivity of CLA ester enzymatic hydrolysis was greatly improved with TLPSs, as high-speed stirring provided a larger interface area for the reaction and product inhibition was effectively reduced by extraction of the product into other phases. Furthermore, the enzyme-enriched phase (liquid immobilization support) was effectively and economically reused more than 8 times because it contained more than 90% of the concentrated enzyme. Therefore, this novel enzymatic esterification-hydrolysis method can be considered ideal to produce high-purity fatty acid monomers.
1. Introduction
Conjugated linoleic acid (CLA) is a mixture of positional and geometric isomers (i.e., cis–trans, trans–cis, cis–cis, and trans–trans) of linoleic acid (C18:2, c9, c12) containing conjugated double bonds.1 CLA is an intermediate for the biohydrogenation of linoleic acid to stearic acid in the rumen of animals, but it can also be produced by alkaline catalyzed conjugation of oil rich in linoleic acid.2 Commonly, the most studied active isomers related to health are cis-9, trans-11 (c9,t11-CLA) and trans-10, cis-12 (t10,c12-CLA), as shown in Fig. 1. As a functional fatty acid, CLA has attracted attention due to its physiological effects, such as its ability to reduce cancer incidence and its antiatherosclerosis, antiobesity, antidiabetes and antihypertensive activities.3,4 Most studies on the physiological effects of CLA are conducted using mixed isomers of CLA. However, it has been reported that the biological effects of CLA isomers are different;5,6 the c9,t11 isomer has a positive anticancer effect, and the t10,c12 isomer is beneficial for reducing body fat.7,8 Therefore, it is strongly desirable to develop an effective and economic method to separate these CLA isomers.
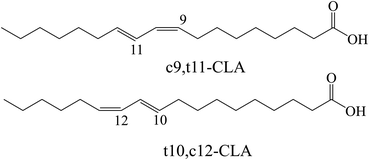 |
| Fig. 1 Structure of the common biologically active isomers of conjugated linoleic acid (CLA). | |
In recent decades, some methods, such as low-temperature crystallization, urea crystallization and microbial isomerization, have been applied in an attempt to separate the two abovementioned isomers.9–13 Due to the subtle differences in solubility values between these two isomers, there may be almost no difference in their crystallization behavior in the solution phase. Additional additives (such as medium chain fatty acids) are added for multiple crystallization to enrich a large number of target isomers, but the cumbersome operations and high energy consumption caused by low temperatures are hardly suitable for industrial applications.14 Urea and methanol are added to the CLA mixture to form urea–FFA complex crystals, making t10,c12-CLA more easily retained.15 However, due to low selectivity, it is often necessary to combine it with other methods such as enzyme catalysis and molecular distillation to obtain high-purity products, and urea cannot be used for food processing. Although high-purity target isomer can be obtained through microbial synthesis, low concentration production remains a challenge for large-scale production.16 Most of these processes have problems such as high energy consumption, complex operations, and environmental pollution, therefore a greener process is needed. Enzymatic purification of CLA isomers is considered a green and efficient method due to its high efficiency and the use of only mild reaction conditions.17 However, in most reaction systems, the purities of CLA isomers obtained by one-step enzymatic purification are too low to meet industrial requirements.17,18 Even if expensive immobilization techniques are used to improve enzyme selectivity, the purity of the c9,t11 isomer in the product is still lower than 75%.19 Therefore, it is necessary to develop a novel process to obtain products with higher purity.
Recently, multistep enzyme-catalyzed reactions have been considered an effective method to obtain high-purity CLA isomers. In most cases, two-step enzymatic selective esterification has been widely used.10,15,20 However, using the CLA esters purified in the first esterification step directly in the second esterification step is difficult. Therefore, a series of complex steps (i.e., chemical hydrolysis, and molecular distillation) must be performed to convert the CLA esters into the partially purified CLA, which can then be secondly enzymatic selectively esterified. Furthermore, the final high-purity CLA ester can also be converted into highly pure CLA isomers by similar complex chemical hydrolysis and separation steps.10,20 As a result, this method is almost difficult to industrialize due to the complex process, high energy consumption and potential health hazards arising from high-temperature operation. In fact, it is also possible to obtain the high-purity CLA isomers by direct enzymatic hydrolysis of the partially purified CLA after enzymatic esterification. This process avoids a series of complicated rehydrolysis and reesterification steps. However, in a traditional oil/water (O/W) two-phase system, enzyme selectivity during the hydrolysis process is lower than that during the esterification process, resulting in its industrial applications being limited.13 Therefore, the development of an enzymatic hydrolysis system with high efficiency and recyclability is the key factor for industrialization. Thus, to solve the above problems, we considered using a three-liquid-phase system (TLPS) to develop a novel and efficient enzymatic hydrolysis process for the selective enrichment of CLA isomers.
The TLPS integrates an aqueous two-phase system (ATPS) and an O/W system. Classic TLPSs are composed of a water/salt phase, a nonpolar phase, and a polar phase provided by a polar-phase-forming component (PFC) (e.g., ionic liquids (ILs), water-soluble polymers and deep eutectic solvents (DES)). In recent years, the simplicity of the process and the low cost of phase forming materials have attracted considerable attention in the separation of multiple components, as it can extract products with different polarities into corresponding phases simultaneously.21,22 In our previous work, some TLPSs were shown to improve the efficiency and selectivity of enzymes for many applications, such as the selective hydrolysis of fish oil and phospholipids.23,24 Compared with the traditional O/W system, this novel system has several advantages, such as high catalytic efficiency, low energy consumption, and minimal health hazards, as well as facile recovery of the product and enzyme. Therefore, this enzymatic hydrolysis system is particularly suitable for replacing the traditional O/W method to purify CLA isomers.
In this study, a novel enzymatic esterification-hydrolysis method based on a TLPS was developed to purify CLA isomers (Fig. 2). First, a novel efficient enzymatic hydrolysis system was developed based on the TLPS. In the TLPS, CLA and the enzyme were enriched in the top phase and middle phase, respectively, and part of the byproduct (ethanol) was distributed to the bottom phase. As a result, the catalytic efficiency of the enzyme was greatly improved because product inhibition was considerably reduced. When this TLPS was used to hydrolyze the partially purified conjugated linoleic acid ethyl ester (CLA-EE) produced by enzymatic esterification, a high-purity CLA isomer was obtained and the complex processes of the traditional multistep enzyme-catalyzed reaction were greatly simplified.
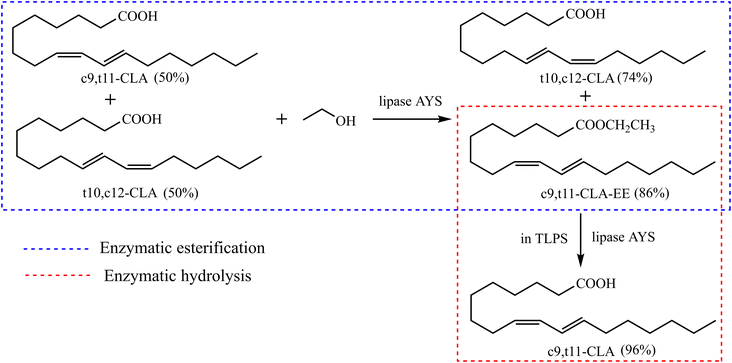 |
| Fig. 2 Two-step enzymatic esterification-hydrolysis reaction for enrichment of c9,t11-CLA isomer based on TLPS. | |
2. Materials and methods
2.1 Materials
All experiments were performed with deionized water. CLA and CLA-EE were purchased from Aohai Biological Co., Ltd (Qingdao, China), which contains almost equal amounts of c9,t11 and t10,c12 isomers. Lipase AYS (Candida rugosa lipases, spray-dried powder) was obtained from Amano (Nagoya, Japan). Methyl heptadecanoate (>97%) was purchased from Aladdin Reagent Co., Ltd (Shanghai, China). HPLC grade ethyl acetate was obtained from Thermo Fisher Scientific Co., Ltd (Shanghai, China). ILs (1-butyl-3-methylimidazolium hexafluorophosphate ([BMIM]PF6) and 1-butyl-3-methylimidazolium tetrafluoroborate ([BMIM]BF4)) were obtained from Lanzhou Institute of Chemical Physics (Lanzhou, China). PEG 400 was obtained from Aladdin Reagent Co., Ltd (Shanghai, China). All other reagents and chemicals were of analytical grade.
2.2 Partition behaviour of lipase in various systems
The lipase was dissolved in water at room temperature with a solvent ratio of 1
:
100 (w
:
v). The synthesis method of deep eutectic solvents (DES) can be found in the ESI.† Various ATPSs consisting of 15–35% (wt%) salt (Na2SO4, (NH4)2SO4, K2HPO4) and PFC (polyethylene glycol, IL or DES) were prepared on a 1.5 g scale by adding a solid salt and a PFC to the enzyme solution. Afterward, 0.08 g of CLA-EE was added into the above ATPSs. The mixture was vortexed for 10 s to form various TLPSs, and the various phases were separated by centrifugation at 2800×g for 5 min. The lipase activities in the middle phase and bottom phase were analyzed by measuring the hydrolysis rate of p-nitrophenyl octanoate (PNPO).25 First, 80 μL of Tris–HCl buffer (25 mM) and 10 μL of PNPO ethanol solution (10 mM) were added to a 96-well plate and preheated at 37 °C for 5 min. The above mixed solution was added to 10 μL of the middle phase or bottom phase (diluted 10- to 50-fold in Tris–HCl buffer) to start the reaction. After 10 min of reaction, 100 μL of 1-propanol was added to stop the reaction, and the absorbance at 405 nm of each well was immediately measured. A unit of enzyme activity (U) was defined as the amount of enzyme required to hydrolyze 1 mmol of PNPO per minute at 37 °C. The partition coefficient of lipase (K) was defined as the ratio of the lipase activity of the middle phase to that in the bottom phase. The recovery (Y) was defined as the ratio of the lipase activity of the middle phase to the total lipase activity. The value of Y was determined from the following formula using the lipase partition coefficient and the volume ratio of the middle phase to the bottom phase.
where, EM and EB are the enzyme activities of the middle phase and the bottom phase respectively, and R is the ratio of the volume of the middle phase to the volume of the bottom phase.
2.3 Selective hydrolysis of CLA-EE in various TLPSs
The enzymatic hydrolysis of CLA-EE by lipase AYS in different TLPSs was investigated in 10 mL conical flasks with each flask, as described in Section 2.2. A control experiment was also carried out by mixing 0.08 g of commercial CLA-EE with 1.5 g of enzyme solution containing 9 mg of lipase. Each flask was agitated for 2 h at 200 rpm in a shaking bath at 40 °C. Finally, each reaction mixture was centrifuged at 1000×g for 3 min. The sample of the top phase was analyzed by GC as described below. All reactions were performed under atmospheric pressure, and all experiments were performed in triplicate.
2.4 Effects of PEG 400 and (NH4)2SO4 concentrations on CLA-EE hydrolysis in TLPS
The effects of the (NH4)2SO4 and PEG 400 concentrations were investigated by adding solid salts and PEG 400 to the enzyme solutions (1%, wt%) to form an ATPS consisting of 17–29% (wt%) (NH4)2SO4 and 17–29% (wt%) PEG 400, and then, 0.08 g of CLA-EE was added to the system. The reaction system was agitated in a shaking bath set at 200 rpm for 2 h and then centrifuged at 1000×g for 3 min. Samples from the various TLPSs were collected and analyzed. Furthermore, the effect of pH on the hydrolysis of CLA-EE in the PEG 400/(NH4)2SO4 system was studied.
2.5 Esterification
Twenty grams of commercial CLA mixtures and 4 mL of ethanol were added to a 100 mL conical flask. Then, 0.4 g of enzyme was dissolved in 6 mL of water and added to the above solution. The reaction was performed at 40 °C in a shaking bath at 150 rpm. Samples from the mixtures in each flask were withdrawn at different times. The esterification degree of total fatty acids was determined by titrating with a 0.05 N NaOH solution.26 The degree of esterification of the c9,t11 isomer was calculated with the following formula from the ratio of the amount of c9,t11 isomer in the ethyl esters to the total amount of corresponding isomer in the reaction mixture.
After the reaction was completed and the mixture was centrifuged, the oil phase was removed into a conical flask, and an appropriate amount of n-hexane was added. Then, a 0.5 N NaOH solution in 30% ethanol was used to remove the unreacted CLA. Three washes were performed with deionized water. The organic solvent was evaporated with a rotary evaporator to obtain CLA-EE (enriched c9,t11 isomer).
2.6 Reuse of the middle enzyme-rich phase
First, 5 g of solid salt, 5 g of PEG 400 and 1.2 g of synthesized CLA-EE were added to 15 g of enzyme solution (5%, wt%) to form the TLPS. The substrate was hydrolyzed at 40 °C for 1 h. After the reaction, the middle phase was collected by centrifugation and used in the next reaction, mixing with the original top and salt-enriched phases to form a new TLPS and continuing the reaction to generate the next batch.
2.7 Analytical methods
Confocal microscopy. Fluorescence images of the TLPS microstructures were acquired by laser scanning confocal microscopy (LSCM; Leica, Germany).23 For fluorescence imaging, the oil phase was labeled with Nile red (excited at 553 nm) and the middle phase was labeled with fluorescein (excited at 460 nm). After mixing the TLPS or O/W system for at least 5 min, a drop of the stained sample was placed on a confocal microscope slide to observe its microstructure.
Droplet size analysis. The droplet size was analyzed using a Mastersizer 2000 laser diffractometer equipped with a Hydrosizer 2000S module (Malvern Instruments, UK). The sizes of the CLA-EE droplets in different continuous phases (purified water and the middle and bottom phases of the TLPS) were measured. First, CLA-EE was dropped into the continuous phase and stirred at 2700 rpm. When the shading rate of the sample reaches 10–20%, the size of the droplet was measured after stirring for 5 minutes. The size of the middle phase droplet in the bottom phase was also measured. Each sample was measured three times.
Determination of the hydrolysis degree of the c9,t11 isomer. The hydrolysates were analyzed by means of a GC instrument (Agilent 7890A) equipped with a hydrogen flame ionization detector (GC-FID).27 Modified polyethylene glycol polar columns (OV-351, 60 m × 0.32 mm i.d., 0.25 μm film thickness, Ohio Valley) were used to analyze the samples. The carrier gas was N2, and the flow rate was 3.0 mL min−1. The injector and detector temperatures were both 250 °C. The column temperature was initially held at 150 °C for 4 min, increased to 230 °C at a rate of 4 °C min−1, and then held at 230 °C for 20 min. The compounds observed by means of GC were identified by comparison of their retention times with those of known standards. Methyl heptadecanoate (C17:0) was used as the internal standard for quantitative analysis. The hydrolysis degree of CLA-EE was defined as the hydrolysis degree of the c9,t11 isomer, which was calculated as follows.
where, [CLAEE]0 and [CLAEE]t are the contents of the c9,t11 isomer in the ethyl ester fraction before and after hydrolysis, respectively.
Fatty acid composition analysis. The ester and free fatty acid (FFA) in the reaction products were separated by TLC.28 The developing agent was n-hexane
:
ether
:
glacial acetic acid (80
:
20
:
1 v/v). The composition of CLA isomers in the FFA fraction was determined by the method of Kim et al.17 The sample was scraped into a test tube, and 2 mL of a 0.5 N KOH/methanol solution was added before heating for 15 min at 70 °C. Then, the flask was cooled for 5 min, 2 mL of BF3-diethyl ether/methanol solution was added to the mixed solution and heated in a water bath for 5 min at 70 °C. Next, 1 mL of a hexane and aqueous NaCl mixture was added to the reaction mixture to extract the fatty acid methyl esters (FAMEs) for GC analysis as previously described. The purity of the c9,t11 isomer was calculated according to the following formula.
where, c9,t11 and t10,c12 are the contents of the c9,t11 isomer and the t10,c12 isomer in the FAME, respectively.
3. Results and discussion
3.1 Selective hydrolysis of CLA-EE in various TLPSs
To successfully develop a two-step enzymatic esterification-hydrolysis method for CLA isomers separation, the key factor is the effective improvement of the selectivity and efficiency of the enzyme in the traditional hydrolysis system.13 Because of the substrate specificity of the enzyme, it is critical to screen an enzyme with high selectivity for a single isomer. However, we found that the selectivity of the enzyme was low on the traditional O/W system (Table S1 in ESI†). The highest purity of the c9,t11 isomer in the product was only 86% with a low conversion (24%). To solve this problem, we attempted to use a TLPS to develop a novel and highly efficient enzymatic hydrolysis system to purify CLA isomers.
Different salts (Na2SO4, (NH4)2SO4, and K2HPO4) and PFCs (water-soluble PEG, ILs and DESs) and the lipase AYS solution were mixed, and the CLA-EE was added to form TLPSs (Table S2 in ESI†). In general, the influences of the PFC and salt type on TLPS formation were similar to those observed in our previous research.29,30 These data indicate that the miscibility of the other two phases was only slightly affected by the small amount of CLA-EE present, possibly because in the polarity of the top phase (CLA-EE) was quite different from that of the other polar phases. The distribution of lipase was investigated in various TLPSs, as shown in Tables 1 and S3 in the ESI.† In most of the tested polymer/salt TLPSs, the majority of the lipases tended to be effectively enriched in the middle phase and had high recovery. In contrast, the distributions of enzyme in the IL/salt TLPSs were more complex; in some systems, the enzyme was enriched in the bottom phase (i.e., the [BMIM]BF4/Na2SO4 system). High recovery of the enzyme in the middle phase was conducive to the reuse of the enzyme because most of the products (CLA and ethanol) were separated from the middle phase through distribution to the other phases, which may protect the structure of the enzyme. Notably, in mostly low molecular weight PEG/salt TLPSs, lipase displayed excellent potential for use in the selective hydrolysis of CLA-EE because of its high catalytic efficiency and selectivity. For example, in the PEG 400/(NH4)2SO4 TLPS, high-purity c9,t11-CLA (91%) was obtained and the degree of hydrolysis was greatly increased by approximately 65% relative to that in the O/W system. However, the hydrolysis reaction did not occur in the DES/salt TLPSs. Previous studies have shown that some PFCs may alter the enzyme structure through extensive hydrogen bonding interactions, which may change the catalytic activity of the enzyme.31–33 In addition, it is noteworthy that acid salts severely limited the catalytic activity of the enzyme. For example, there was no hydrolysis reaction in the K2HPO4 system due to the alkaline environment. Furthermore, when the reaction was carried out in an O/W system, it was difficult to separate the oil phase from the water phase, and high-speed or high-temperature centrifugation was usually required.34 In the TLPS, this problem can be overcome because each phase can be easily separated by gravity or low-speed centrifugation, which also allows the enzyme to be reused. In summary, considering the high catalytic efficiency and selectivity of the enzyme, the PEG 400/(NH4)2SO4 TLPS was selected for further research.
Table 1 The effect of selective hydrolysis of CLA-EE isomers and the distribution behaviour of lipase AYS in different systemsa
|
PEG400a |
PEG400b |
PEG400c |
PEG600b |
PEG2000b |
BMIM[BF4]a |
BMIM[PF6]b |
CUc |
O/W |
Purity (%): the purity of c9,t11-CLA in FFA fractions after hydrolysis; hydrolysis (%): the hydrolysis degree of c9,t11 isomer; the composition of the salt in the TLPS: a Na2SO4 (15%, wt%), b (NH4)2SO4 (18%, wt%), c K2HPO4 (20%, wt%); and the composition of the PFC in the TLPS: 20% (wt%); CU: choline chloride : urea = 1 : 2, 35% CU, 25% K2HPO4. |
K |
24.2 ± 1.7 |
12.7 ± 1.3 |
7.6 ± 1.4 |
6.2 ± 0.3 |
1.2 ± 0.2 |
0.8 ± 0.0 |
96.6 ± 2.8 |
1.7 ± 0.3 |
— |
Y (%) |
95.8 ± 0.8 |
89.6 ± 2.3 |
85.3 ± 2.3 |
81.7 ± 0.6 |
45.1 ± 3.9 |
17.0 ± 0.7 |
99.7 ± 0.1 |
83.0 ± 2.5 |
— |
Purity (%) |
91.2 ± 0.3 |
91.1 ± 0.3 |
— |
91.2 ± 0.5 |
61.6 ± 1.1 |
76.5 ± 1.0 |
87.2 ± 0.2 |
— |
86.4 ± 0.6 |
Hydrolysis (%) |
34.5 ± 1.2 |
40.3 ± 1.3 |
— |
34.9 ± 1.8 |
24.6 ± 2.7 |
4.1 ± 1.5 |
31.6 ± 2.1 |
— |
24.1 ± 1.3 |
3.2 The effects of (NH4)2SO4 and PEG 400 concentration on the selective hydrolysis of CLA-EE in the TLPSs
To boost both the reaction efficiency and the ability to reuse the enzyme, the effects of the salt and PEG concentrations on the distribution of lipase in the PEG 400/(NH4)2SO4 TLPS were first studied (Fig. 3). As the concentrations of salt and PEG increased, the changes in the partition coefficient of lipase showed similar trends (first increasing and then decreasing). One possible reason is that the hydrophobic interaction between the phase and the lipase has an important influence on the partitioning behavior of the enzyme.35 However, the concentration of salt seemed to have a greater effect on the partition coefficient of lipase (from a maximum value of 17 to a minimum value of 2) than the concentration of PEG. In general, the change in lipase recovery was similar to that in the partition coefficient because the partition coefficient (K) had a large effect on lipase recovery (Y). However, with increasing PEG concentration, the change in lipase recovery seemed more complicated because the change in recovery was also affected by the phase volume ratio (middle/bottom, Rm/b). An increase in the PEG concentration led to a decrease in the partition coefficient and an increase in the phase volume ratio Rm/b.
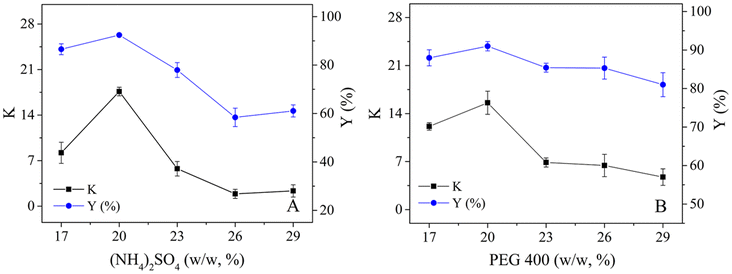 |
| Fig. 3 Effect of (NH4)2SO4 and PEG 400 concentration on the partition coefficient and recovery of lipase AYS in the TLPS; (A) concentration of PEG 400 (20%, wt%); (B) concentration of (NH4)2SO4 (20%, wt%). | |
The degree of CLA-EE isomer enzymatic hydrolysis and the purity of c9,t11-CLA in the product were studied (Fig. 4). In general, the purity of c9,t11-CLA was high level (approximately 90%) in most of the TLPSs, indicating that the selectivity of the enzyme was less affected by the changes in the salt and PEG concentrations. However, as the salt or PEG concentration increased, the hydrolysis degree of CLA-EE clearly changed and was similar to the change in the lipase partition coefficient. A possible reason for this result was that the enzyme is enriched in the middle phase of the TLPSs, which effectively improved the reaction efficiency because more enzyme was available to participate in the interfacial catalytic reaction between the middle phase and the top (substrate) phase.
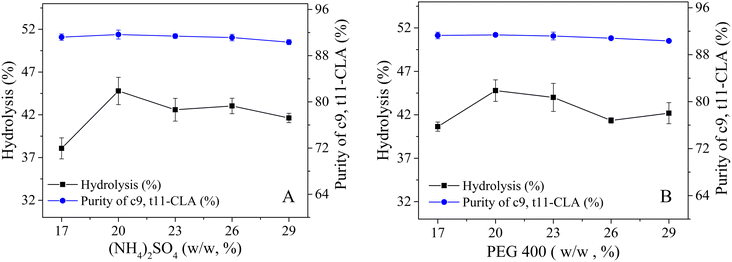 |
| Fig. 4 Effects of (NH4)2SO4 and PEG 400 concentration on selective hydrolysis of CLA-EE isomers in TLPS; (A) concentration of PEG 400 (20%, wt%); (B) concentration of (NH4)2SO4 (20%, wt%). | |
The catalytic efficiency of the enzyme is affected by pH, so the enzymatic hydrolysis of CLA-EE isomers was also studied at different pH values (Table S4 in the ESI†). In general, the degree of hydrolysis in alkaline environments was much lower than that in acidic environments. One possible reason for this result is that the structure of the enzyme was negatively affected by the alkaline environment. Furthermore, the product CLA can react with the acid salts to form fatty acid salts with surfactant properties, thus trapping the substrate at the interface and isolating it from the enzyme, resulting in a significant decline in the hydrolysis efficiency of CLA-EE. Considering that the inherent pH value of the (NH4)2SO4/PEG 400 system was within the optimal range, the pH value of the system did not need to be adjusted for the subsequent reaction. Considering the large partition coefficient of lipase and the high c9,t11-CLA enrichment efficiency after enzymatic hydrolysis, 20% (NH4)2SO4 and 20% PEG 400 was selected as the best system for further study.
3.3 Microstructure of the TLPS and its effect on the catalytic efficiency of the enzyme
To explain the improvement in enzyme efficiency in the TLPS, CLA-EE was enzymatically hydrolyzed in an O/W system, an oil/middle phase (O/M: remove the bottom phase in TLPS) system and the TLPS (Fig. 5(A) and (B)). The results showed that at the same degree of hydrolysis, the selectivity of the enzyme remaining in the O/M system or the TLPS was much higher than that in the O/W system (Fig. 5(A)). A possible reason for this finding is that the solvent and salt improved the enzyme selectivity by increasing its conformational rigidity.36 In general, an increase in enzyme rigidity decreases the catalytic efficiency because maintaining optimal conformational flexibility is required for full activity.37,38 Surprisingly, the selectivity and catalytic efficiency of enzymes in TLPSs can be improved simultaneously. For example, the initial reaction rate (1 h) in the TLPS was increased by approximately 65% relative to that in the O/W system. The reason for this result is not completely clear, but notably, when the aqueous phase was replaced by the middle phase, the efficiency increased by approximately 18% (O/M system vs. O/W system) at 1 h. One possible explanation for this phenomenon is that the oil droplets in the middle phase were more dispersed than those in the water phase, resulting in a larger interfacial area between the oil droplets and the middle phase, which was more conducive to the catalytic efficiency of the enzyme.
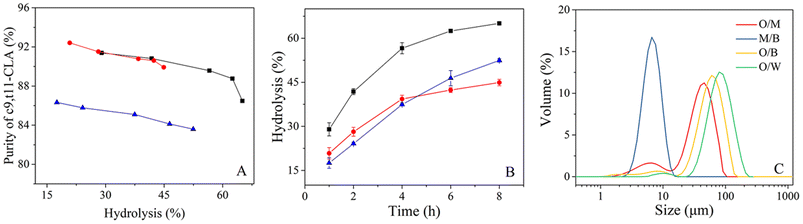 |
| Fig. 5 (A) The effects of selective hydrolysis of CLA-EE isomers and purity of c9,t11-CLA in products in the O/W system, O/M system and TLPS. (B) The degree of selective hydrolysis of CLA-EE isomers in the O/W system, O/M system and TLPS. Symbols: (●) O/M system; (■) TLPS; (▲) O/W system. (C) Size distribution of the oil droplets in water and the two different phases of the TLPS. O/M: oil phase in the middle phase; O/W: oil phase in water; M/B: middle phase in the bottom phase; O/B: oil phase in the bottom phase. | |
To test this assumption, the particle size distribution of the oil phase (CLA-EE) in different continuous phases was determined (Fig. 5(C) and Table S5 in the ESI†). When the continuous phase (aqueous phase) in the O/W system was replaced by the middle phase of the TLPS, the surface area of the CLA-EE droplets increased from 0.086 to 0.29 m2 g−1. In addition, when the bottom phase was added to the O/M system to form the TLPS, the hydrolysis degree of CLA-EE in the TLPS increased by an additional 40% relative to that in the O/M system (1 h). A possible reason for this result is that there was a very small amount of substrate dissolved in the middle phase. This part of CLA-EE reached the enzyme-enriched interface of the middle/bottom phase (M/B), where it was catalytically hydrolyzed by phase transfer catalysis. After measurement, we found that the interface area of this new catalytic interface (M/B) was nearly ten times the O/M interface area, which greatly improved the catalytic efficiency of the enzyme.
In an O/W two-phase system, there is only one interface, so the reactive interface area is clearly defined as the surface area of the oil droplet. However, the real area of the reaction interface is not easy to define in the TLPS because of the presence of multiple interfaces (i.e., the oil/middle phase interface, oil/bottom phase interface and bottom/middle phase interface). Therefore, to observe the true reaction interface during the high-speed stirring reaction, fluorescence images of the TLPS were obtained by confocal microscopy (Fig. 6). Fluorescein and Nile red were used to label the middle phase (green) and the oil phase (red), respectively. As shown in Fig. 6(A)–(C), dispersed droplets of the oil phase (CLA-EE) and a portion of the bottom phase droplets were surrounded by the middle phase, which was suspended in the continuous bottom phase, forming a new emulsion (W2 + O)/W1/W2 (W1 and W2 represent the middle and bottom phases, respectively). Compared with the conventional O/W system, the reaction that occurred at the interface of the oil phase and the middle phase could be effectively increased because the smaller size of the oil droplets (red) in the TLPS could be obtained (Fig. 6(C) vs. (D)). More importantly, the reaction could also occur at the interface of the small bottom phase wrapped in the middle phase, whose area was much larger than the O/M interface area in the TLPS. Additionally, the ethanol (byproduct) produced by the hydrolysis reaction can be extracted into the bottom phase from the middle phase (enzyme-enriched phase), reducing product inhibition and the toxicity to the enzyme. Furthermore, the improvement in the stereoselectivity of lipase in the TLPS played an important role in the purification of isomers during CLA-EE hydrolysis.
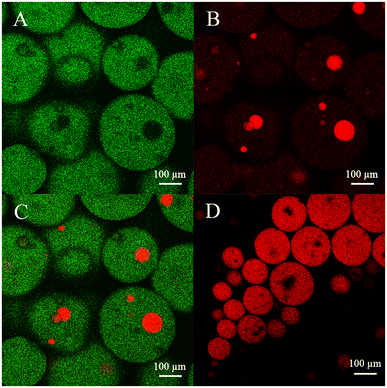 |
| Fig. 6 Confocal microscopy images. (A) TLPS microstructure obtained at λex 460 nm; green corresponds to the fluorescence of fluorescein enriched in the middle phase. (B) TLPS microstructure obtained at λex 553 nm; red corresponds to the fluorescence of Nile red enriched in the oil phase. (C) Microstructure image of the TLPS obtained by combining (A) and (B). (D) Microstructure of the O/W system. | |
3.4 Obtaining high-purity c9,t11-CLA by the combination of two-step selective enzymatic esterification-hydrolysis reactions
Although 90% pure c9,t11-CLA has been obtained in a TLPS by a one-step enzymatic hydrolysis reaction, it is still difficult to prepare a CLA isomer that can meet industrial requirements (over 95%) by this method. To solve this problem, a novel enzymatic esterification-hydrolysis method was developed to obtain high-purity isomers. First, the CLA mixture was selectively enzymatically esterified. After 10 h of esterification, the reaction mixture was washed with alkali solution and extracted with n-hexane, and a partially purified CLA-EE (purity of c9,t11 isomer, 85%) was obtained (Fig. 7(A)).
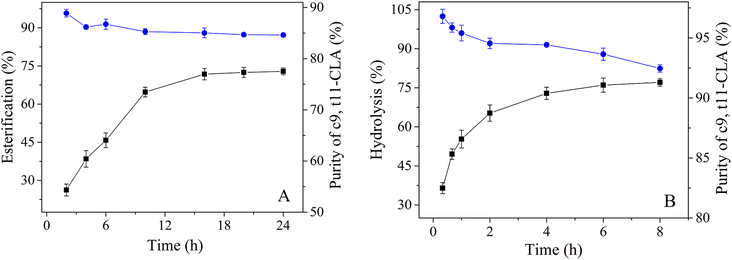 |
| Fig. 7 Purification of the c9,t11 isomer from the enzymatic esterification-hydrolysis two-step process. (A) Esterification process and (B) hydrolysis process. Symbols: (●) purity of c9,t11-CLA; (■) degree of esterification or hydrolysis. | |
Second, the CLA-EE from the first step was added to the (NH4)2SO4/PEG 400 system to form a TLPS, and enzyme solutions of different concentrations were studied (Fig. S1 in the ESI†). The reaction velocity depended on the amount of lipase, and it increased with the increase in the amount of lipase used. When the concentration of the enzyme solution was more than 5%, the degree of hydrolysis was less improved. Therefore, 5% of the enzyme solution was selected for further investigation. In this novel system, CLA-EE was further purified by selective enzymatic hydrolysis, and the c9,t11-CLA isomer was obtained with high purity (96%) after 1 h (Fig. 7(B)). Notably, in the TLPS, with the improvement in the hydrolysis degree of CLA-EE, the selectivity of the enzyme did not significantly change. Even after 6 h of hydrolysis, when the rate of hydrolysis of CLA-EE increased to 75%, the purity of c9,t11-CLA remained high (approx. 94%). This result indicates that approximately half of the c9,t11 isomers in the substrate as simply enriched with high purity through the enzymatic esterification-hydrolysis method, making this process suitable for industrial applications.
Although several multistep enzymatic methods to purify CLA isomers have been reported10,15,20 (Fig. 8(A)), these processes require not only multistep reactions (two-step esterification (a) and (c) and two-step chemical hydrolysis (b) and (d)) but also a series of complex separation steps (i.e., multistage molecular distillation and/or urea adduct formation). As a result, the whole reaction process is extremely complex and can even involve more than 10 steps to obtain high-purity c9,t11-CLA. When this novel enzymatic esterification-hydrolysis reaction based on the TLPS was used to produce CLA isomers with high purity, the complex steps of rehydrolysis and reesterification and some separation procedures with high costs and potential health hazards were reduced or avoided (Fig. 8(B)). As a result, the whole reaction process was greatly simplified to less than 4 steps. Considering that this novel process could also greatly improve reaction efficiency and product purity, we believe that it has better industrial application prospects than the multistep enzymatic esterification process.
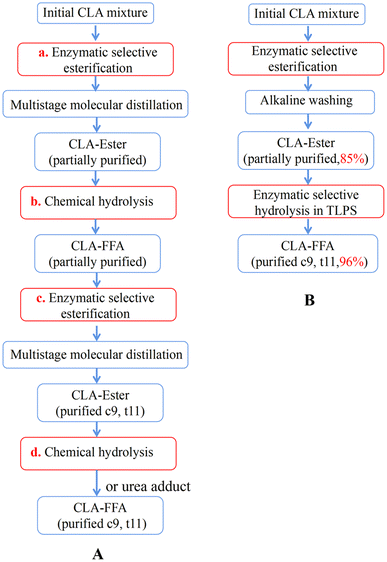 |
| Fig. 8 Process of enzymatic purification of c9,t11-CLA; (A) traditional multistep enzymatic methods; (B) two-step enzymatic esterification-hydrolysis method. | |
3.5 Reuse of the PEG-enriched phase containing lipase in TLPS
Although selective enzymatic reactions can effectively produce high-purity products containing the c9,t11 isomer, the operational stability and reuse of the enzyme limit the industrial-scale applications of this process. In TLPSs, the PEG-enriched phase (middle phase) can be reused as a liquid immobilization support since most of the enzyme is concentrated in this phase.
To evaluate the reusability of the enzyme in the TLPS, CLA-EE was repeatedly hydrolyzed by the PEG-enriched phase, and the results are shown in Fig. 9. Generally, after 8 batches, the selectivity of the enzyme was not significantly changed, and the purity of c9,t11-CLA was maintained between 93-96%. After 8 repeated reactions, the enzyme activity remained at more than 90% of its initial activity, and the hydrolysis efficiency was 70% that of the initial hydrolysis efficiency. As mentioned above, PEG helps to protect lipase from harmful substances during the selective hydrolysis of CLA-EE, which may be part of the reason for the good reusability.39
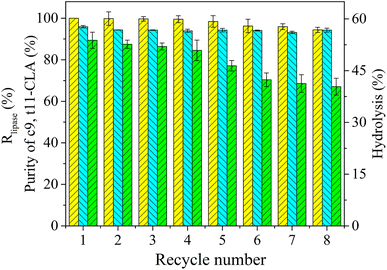 |
| Fig. 9 Reusability of the PEG-enriched phase (middle phase) of the TLPS for the hydrolysis of CLA-EE. (Yellow) Rlipase: relative enzyme activity; (cyan) purity of c9,t11-CLA; (green) the degree of hydrolysis. | |
In previous reports, immobilized enzymes were used for selective esterification to purify CLA isomers.19 After 6 batches, the rate of esterification rate was only 20–60% that of the original value. Furthermore, recovery of the immobilized enzymes required washing with phosphate buffer solution and drying, making their recovery less convenient in industrial applications. In addition, as the solid carrier is decomposed by the high-speed rotating impeller, the immobilized enzyme may be irreversibly lost, making recovery and recycling of the lost enzyme difficult. TLPSs can overcome the above problems because the droplets dispersed with vigorous stirring will automatically reassemble to form an enzyme-rich phase after stirring is stopped. In addition, most of the available solid immobilization carriers may have potential health hazards, and PEG may be safer and more stable because of its nontoxicity and low vapor pressure.40
4. Conclusions
In summary, an enzymatic esterification-hydrolysis method based on a TLPS was developed and used to produce the c9,t11-CLA isomer in high purity. Compared with the conventional two-step enzymatic esterification method, this novel method not only produced highly pure c9,t11-CLA (96%) with high conversion (approx. 36%) but also greatly simplified the reaction and separation processes. The high hydrolysis efficiency and selectivity of the enzyme for CLA esters in the TLPS were the major reasons why this novel method could be successfully developed. When the TLPS was stirred at high speed, a novel (W2 + O)/W1/W2 multiple emulsion was formed, which was the main factor responsible for improving the hydrolysis efficiency of the enzyme because it not only relieved product inhibition but also provided a larger catalytic interface. Furthermore, the enzymes used in this system were recycled, and the products could be conveniently isolated. This novel system also has several other advantages suitable for industrialization, such as easy recovery of the product, low toxicity and high enzyme reusability.
Conflicts of interest
There are no conflicts to declare.
Acknowledgements
This work was supported by National Key Research and Development Program of China (2019YFD1002405), National Natural Science Foundation of China (22178132, 21776103), National Science Fund for Distinguished Young Scholars of China (31725022), Natural Science Foundation of Guangdong Province (2020A1515011006).
Notes and references
- H. S. Moon, H. G. Lee, C. S. Chung, Y. J. Choi and C. S. Cho, Nutr. Metab., 2008, 5, 16 CrossRef PubMed.
- L. J. den Hartigh, Nutrients, 2019, 11, 370 CrossRef CAS PubMed.
- M. Dachev, J. Bryndova, M. Jakubek, Z. Moucka and M. Urban, Processes, 2021, 9, 454 CrossRef CAS.
- S. H. Li, L. W. Xu, J. J. Qing, X. D. Wu, H. X. Li, H. B. Chen and X. H. Liu, Food Chem., 2023, 409, 135257 CrossRef CAS PubMed.
- M. Viladomiu, R. Hontecillas and J. Bassaganya-Riera, Eur. J. Pharmacol., 2016, 785, 87–95 CrossRef CAS PubMed.
- B. Yang, H. Q. Chen, C. Stanton, R. P. Ross, H. Zhang, Y. Q. Chen and W. Chen, J. Funct. Foods, 2015, 15, 314–325 CrossRef CAS.
- G. Fuke and J. L. Nornberg, Crit. Rev. Food Sci. Nutr., 2017, 57, 1–7 CrossRef CAS PubMed.
- J. Wang, X. Liu, X. Zhang, J. Liu, S. Ye, S. Xiao, H. Chen and H. Wang, Chem. Phys. Lipids, 2013, 175, 27–32 CrossRef PubMed.
- O. Berdeaux, W. W. Christie, F. D. Gunstone and J. L. Sebedio, J. Am. Oil Chem. Soc., 1997, 74, 1011–1015 CrossRef CAS.
- T. Kobayashi, T. Nagao, Y. Watanabe, Y. Yamauchi-Sato, S. Negishi and Y. Shimada, J. Am. Oil Chem. Soc., 2006, 83, 93–99 CrossRef CAS.
- N. Niezgoda, P. Mitula and C. Wawrzenczyk, Przem. Chem., 2011, 90, 949–957 CAS.
- A. A. Khaskheli, F. N. Talpur, A. C. Aydin, S. Jawaid, M. A. Surhio and H. I. Afridi, Biosci., Biotechnol., Biochem., 2017, 81, 2002–2008 CrossRef CAS PubMed.
- N. Niezgoda and C. Wawrzenczyk, J. Mol. Catal. B: Enzym., 2014, 100, 40–48 CrossRef CAS.
- H. Uehara, T. Suganuma, S. Negishi, S. Ueno and K. Sato, J. Am. Oil Chem. Soc., 2006, 73, 261–267 CrossRef.
- T. Nagao, Y. Yamauchi-Sato, A. Sugihara, T. Iwata, K. Nagao, T. Yanagita, S. Adachi and Y. Shimada, Biosci., Biotechnol., Biochem., 2003, 67, 1429–1433 CrossRef CAS PubMed.
- Y. C. Mei, H. Q. Chen, B. Yang, J. X. Zhao, H. Zhang and W. Chen, Int. J. Food Microbiol., 2022, 369, 109593 CrossRef CAS PubMed.
- J. Kim, M.-Y. Chung, H.-D. Choi, I.-W. Choi, I.-H. Kim, H. S. Chun and B. H. Kim, J. Am. Oil Chem. Soc., 2017, 94, 577–585 CrossRef CAS.
- Y. Yamauchi-Sato, T. Nagao, T. Yamamoto, T. Terai, A. Sugihara and Y. Shimada, J. Oleo Sci., 2003, 52, 367–374 CrossRef CAS.
- W. H. Yu, D. S. Tong, M. Fang, P. Shao and C. H. Zhou, J. Mol. Catal. B: Enzym., 2015, 111, 43–50 CrossRef CAS.
- T. Nagao, Y. Shimada, Y. Yamauchi-Sato, T. Yamamoto, M. Kasai, K. Tsutsumi, A. Sugihara and Y. Tominaga, J. Am. Oil Chem. Soc., 2002, 79, 303–308 CrossRef CAS.
- B. Braibant, D. Bourgeois and D. Meyer, Sep. Purif. Technol., 2018, 195, 367–376 CrossRef CAS.
- C. E. D. Padilha, C. D. Nogueira, H. N. Almeida, W. de Medeiros, M. A. Oliveira, J. S. de Araujo and E. S. dos Santos, Food Bioprod. Process., 2020, 120, 151–157 CrossRef CAS.
- Z. Li, H. Chen, J. Su, W. Wang, H. Chen, B. Yang and Y. Wang, J. Agric. Food Chem., 2019, 67, 2570–2580 CrossRef CAS PubMed.
- Z. Li, Z. Yang, H. Chen, H. Chen, B. Yang and Y. Wang, Sep. Purif. Technol., 2021, 264, 118404 CrossRef CAS.
- G. Rabbani, E. Ahmad, N. Zaidi, S. Fatima and R. H. Khan, Cell Biochem. Biophys., 2012, 62, 487–499 CrossRef CAS PubMed.
- B. C. Lopez, L. E. Cerdan, A. R. Medina, E. N. Lopez, L. M. Valverde, E. H. Pena, P. A. G. Moreno and E. M. Grima, J. Biosci. Bioeng., 2015, 119, 706–711 CrossRef PubMed.
- Y. H. Wang, X. F. Li, Y. X. Liang, B. Yang and S. H. Zhang, J. Mol. Catal. B: Enzym., 2007, 46, 20–25 CrossRef CAS.
- G. P. McNeill, C. Rawlins and A. C. Peilow, J. Am. Oil Chem. Soc., 1999, 76, 1265–1268 CrossRef CAS.
- Z. Li, H. Chen, W. Wang, M. Qu, Q. Tang, B. Yang and Y. Wang, Chem. Commun., 2015, 51, 12943–12946 RSC.
- Z. Li, B. Jiang, D. Zhang and Z. Xiu, Sep. Purif. Technol., 2009, 66, 472–478 CrossRef CAS.
- M. M. Zaman, Y. Hayashi, M. M. R. Talukder and T. Kawanishi, Biochem. Eng. J., 2006, 29, 46–54 CrossRef CAS.
- M. M. R. Talukder, T. Takeyama, Y. Hayashi, J. C. Wu, T. Kawanishi, N. Shimizu and C. Ogino, Appl. Biochem. Biotechnol., 2003, 110, 101–112 CrossRef CAS PubMed.
- H. S. Kim, S. H. Ha, L. Sethaphong, Y.-M. Koo and Y. G. Yingling, Phys. Chem. Chem. Phys., 2014, 16, 2944–2953 RSC.
- G. Hu, J. Li and G. Zeng, J. Hazard. Mater., 2013, 261, 470–490 CrossRef CAS PubMed.
- S. S. Nadar, R. G. Pawar and V. K. Rathod, Int. J. Biol. Macromol., 2017, 101, 931–957 CrossRef CAS PubMed.
- A. M. Klibanov, Nature, 2001, 409, 241–246 CrossRef CAS PubMed.
- A. Karshikoff, L. Nilsson and R. Ladenstein, FEBS J., 2015, 282, 3899–3917 CrossRef CAS PubMed.
- S. H. Wang, X. H. Meng, H. Zhou, Y. Liu, F. Secundo and Y. Liu, Catalysts, 2016, 6, 32 CrossRef.
- T. Keeling-Tucker, M. Rakic, C. Spong and J. D. Brennan, Chem. Mater., 2000, 12, 3695–3704 CrossRef CAS.
- J. S. P. Kumar, V. K. Garlapati, A. Dash, P. Scholz and R. Banerjee, Algal Res., 2017, 21, 138–147 CrossRef.
|
This journal is © The Royal Society of Chemistry 2023 |
Click here to see how this site uses Cookies. View our privacy policy here.