DOI:
10.1039/D3RA02193A
(Paper)
RSC Adv., 2023,
13, 19975-19980
Subtilisin integrated artificial plant cell walls as heterogeneous catalysts for asymmetric synthesis of (S)-amides†
Received
3rd April 2023
, Accepted 19th June 2023
First published on 3rd July 2023
Abstract
Subtilisin integrated artificial plant-cell walls (APCWs) were fabricated by self-assembly using cellulose or nanocellulose as the main component. The resulting APCW catalysts are excellent heterogeneous catalysts for the asymmetric synthesis of (S)-amides. This was demonstrated by the APCW-catalyzed kinetic resolution of several racemic primary amines to give the corresponding (S)-amides in high yields with excellent enantioselectivity. The APCW catalyst can be recycled for multiple reaction cycles without loss of enantioselectivity. The assembled APCW catalyst was also able to cooperate with a homogeneous organoruthenium complex, which allowed for the co-catalytic dynamic kinetic resolution (DKR) of a racemic primary amine to give the corresponding (S)-amide in high yield. The APCW/Ru co-catalysis constitutes the first examples of DKR of chiral primary amines when subtilisin is used as a co-catalyst.
1 Introduction
Chiral compounds have a key role in the chemical industry. Most of the active substances in drugs and natural products are optically active.1 Although they have the same chemical structure, enantiomers of chiral drugs can exhibit marked differences in biological activity. This is due to the fact that biomolecules (e.g. amino acids, sugars, nucleosides, nucleotides, etc.) of the biosynthetic apparatus, which produce our proteins, receptors and nucleic acids, are assembled as single enantiomers. During the past four decades many strategies were developed to direct the outcome of organic reactions toward enantiomerically pure products. Enzymes are well known to be powerful tools for the synthesis of chiral molecules with an extremely high degree of stereoselectivity.2 Amides are important functional groups in organic synthesis and they occur in around 35% of all drugs.3 An important way to get access to enantiopure amines and amides is the enzyme-mediated kinetic resolution (KR) of racemic primary amines. The stability, activity and selectivity of the enzyme Candida antarctica lipase B makes it one of the most common enzymes employed for this purpose in industry and academia. Lipases are usually R-selective, directing the stereoselectivity outcome of the amidation reactions only toward the R-enantiomer. Extensive studies were done by Bäckvall regarding the use of CALB in the synthesis of hybrid heterogeneous enzyme-metal catalysts for the dynamic kinetic resolution (DKR) of primary amines.4,5 Some significant examples are the cross-linked enzyme aggregate (CLEA), where CALB itself constitutes the heterogeneous support used as a matrix to incorporate palladium metal nanoparticles4b,c and the immobilization of CALB and palladium nanoparticles on the cavities of siliceous mesocellular foam.4a Subtilisin6 provides a complementary stereoselectivity to the lipase-catalyzed kinetic resolution since the stereospecificity of subtilisin is opposite to that of lipases.7 One of the drawbacks for the use of this enzyme was shown to be the decrease in activity and selectivity in organic solvents compared to the much more stable lipases. Due to this intrinsic structural problem, the use of subtilisin as catalyst was mainly limited to the kinetic resolution of secondary alcohols.7 More recently, alcohols were used as substrates for the subtilisin co-catalyzed DKR of alcohols.8 However, subtilisin has so far not been used for the DKR of primary amines. With respect to the kinetic resolution of chiral amines, only few papers were published until now. In 1989 Klibanov, in his seminal work, showed the possibility to use subtilisin for the stereoselective amidation of secondary amines.9 It was found that the enantioselectivity factor was strongly influenced by the nature of the reaction medium.9 Inspired by the Klibanov article, Gutman subsequently reported a continuous-flow bioreactor, where subtilisin was immobilized on glass beads, for the kinetic resolution of 1-(L-naphthy1)ethylamine.10 In 2009, a large screening on different classes of proteases, to determine the best acyl donor for the kinetic resolution of 3-amino-1-phenylbutane, was made.11 The results were then correlated to an automated docking determination of the binding affinity for subtilisin.11 In 2016, the construction of a continuous flow cascade reactor for the dynamic kinetic resolution of racemic N-Boc-phenylalanine ethyl thioester with benzylamine using subtilisin supported on macroporous silica gels was disclosed.12 To circumvent the decrease in reactivity of biomolecules in non-aqueous media a large variety of organic and inorganic materials were tested as heterogeneous solid supports.
The greatest advantage of immobilization is the significant improvement of the stability of the enzyme by protecting it from undergoing denaturation at elevated temperatures and harsh reaction conditions. Another significant advantage is the ease of isolation and the reusability of the catalyst over successive catalytic cycles. Many different materials such as silica,13 inorganic oxides,14 resins,15 bio-16 and synthetic17 polymers were taken in consideration as scaffolds. Various immobilization techniques have been also developed, including adsorption, covalent binding, entrapment, encapsulation and cross-linking.18 The transition from petrochemical-based raw materials to biomass-based materials have attracted the interest of the scientific community during the last decades.19 Natural polymers received considerable attention because of their abundance, biocompatibility, bio-degradability and non-toxicity.20 Cellulose is the most abundant biopolymer in the world. Its use as a solid support, in the field of heterogeneous catalysis, offers great advantages such as great absorption capacity, good chemical stability, and insolubility in common solvents.21 We have been involved in the valorization of biomass, heterogeneous catalysis and on the construction of functional cellulose-based materials.22–24 Recently, we disclosed a concept for how to assemble artificial plant cell walls (APCW) containing lipase or lipase and Pd nanoparticles within a polysaccharide-based matrix (cellulose) with a multilayer architecture.24 Driven by the successful asymmetric synthesis of (R)-amides by this concept, we became intrigued if we could assemble APCW with activity for the asymmetric synthesis of chiral (S)-amides using subtilisin as enzyme and cellulose as the polysaccharide carrier component. Here we disclose the self-assembly of recyclable APCWs that operates as a highly enantioselective catalyst for the asymmetric synthesis of (S)-amides (Fig. 1). The APCW's co-catalysis with a Ru-complex allowed for the first examples of DKR of primary amines with a catalyst containing a protease.
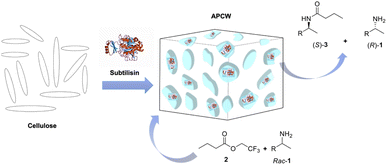 |
| Fig. 1 Simplified scheme of self-assembly of APCW and kinetic resolution of racemic amines 1. | |
2 Experimental
2.1 Self-assembling of APCW catalyst
APCW was self-assembled as shown in Fig. 2. To cellulose (60 mg), dispersed in sodium phosphate buffer (6 mL, 0.1 M, pH 7.2), Brij C10 (20 mg) was added. Brij was selected as the surfactant since it has a beneficial effect for enhancing subtilisin's and CALBs activity in kinetic resolutions of secondary alcohols and primary amines.8,24 The suspension was stirred with a spatula until completely solubilization of Brij. Next subtilisin (20 mg) was added, the mixture was stirred with a spatula until completely solubilization of the enzyme and rapidly frozen in liquid nitrogen. The catalyst was lyophilized for 70 hours to give APCW as a solid white foam.
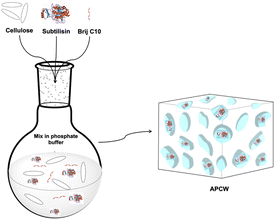 |
| Fig. 2 Self-assembly of APCW. | |
2.2 General procedure for the KR of rac-1
In a microwave vial, was added APCW, solvent (1 mL), amine rac-1 (0.25 mmol, 1 equiv.) and 2,2,2-trifluoroethyl butyrate 2 (85 mg, 0.5 mmol, 2 equiv.). The vial was sealed and flushed with nitrogen. The reaction was stirred at room temperature for the time reported in the table. The reaction mixture was directly purified by column chromatography (hexane
:
ethylacetate) to afford the desired product (S)-3 in the reported yield and ee.
2.3 General procedure for the DKR of rac-1 catalyzed by APCW4 and SHVO catalyst
In a microwave vial was added the APCW4, 4-dimethyl-3-pentanol (1 mL), Shvo catalyst, amine rac-1 (0.25 mmol, 1 equiv.), 2,2,2-trifluoroethyl butyrate 2 (85 mg, 0.5 mmol, 2 equiv.) and Na2CO3. The vial was sealed and an argon balloon was connected. The reaction was stirred at 90 °C for the time reported in the table. The reaction mixture was directly purified by column chromatography (hexane/ethyl acetate 1
:
1) to afford the corresponding amide (S)-3 in the reported yield and ee.
3 Results and discussion
We began our research by screening the influence of the different components of APCW and tuning their ratio to find an optimal system for the kinetic resolution of amine 1a. As shown in the screening table, subtilisin itself is almost inactive in 3-methyl-3-pentanol (Table 1, entry 2) confirming what was reported in the literature and by the kinetic experiments (Fig. 3). Modifying subtilisin with a surfactant polyethylene glycol hexadecyl ether (Brij) led to an increased activity of the enzyme (Table 1, entry 3). The main drawback of this system is that it cannot be recycled and used for multiple reactions. The use of cellulose nanocrystals (CNC) as a support material also led to a similar increase of the activity (Table 1, entry 4). The results show that the nanocellulose alone can activate the subtilisin (entries 4 and 5). This is interesting since Park and Kim have demonstrated that dextrin does not improve the activity of subtilisin in kinetic resolutions of secondary alcohols.8d The assembly of APCW2 (CNC, subtilisin, Brij) was successful and turned out to be better than the previously tested systems. Different solvents were screened for the kinetic resolution of amine 1a (entries 6–11) and 3-methyl 3-pentanol appeared to be the best reaction medium (entry 7).
Table 1 Screening of the APCW-catalyzed kinetic resolutiona
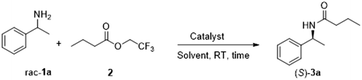
|
Entry |
APCW (assembled components) |
Solvent |
Time [h] |
Yield 3a b [%] |
ee 3a c [%] |
Reaction conditions: 1a (0.25 mmol, 1 equiv.), 2 (0.5 mmol, 2 equiv.), catalyst (9 mg), solvent (1 mL), room temperature. Isolated yield. Determined by chiral HPLC. Yield determined by 1H-NMR. Lyophilized subtilisin 2.2 mg used. Catalyst 18 mg used. Reaction performed at 4 °C. Na2CO3 was added (0.26 mmol). NaOAc was added (0.26 mmol). Ethyl butyrate used as acyl donor. ND stands for not determined. APCW = artificial cell wall, MCC = microcrystalline cellulose, CNC = cellulose nanocrystals, NFC = nanofibrilated cellulose, Brij = polyethylene glycol hexadecyl ether (Mn = 683). |
1d |
— |
3-Methyl 3-pentanol |
168 |
0 |
ND |
2d,e |
Subtilisin |
3-Methyl 3-pentanol |
168 |
8.5 |
ND |
3 |
Sub/Brij (1 : 1) |
3-Methyl 3-pentanol |
23 |
42 |
85 |
4 |
APCW1 CNC/Sub (1 : 1) |
3-Methyl 3-pentanol |
42 |
46 |
82 |
5 |
APCW1 |
THF |
46 |
37 |
45 |
6f |
APCW2 CNC/Sub/Brij (1 : 1:1) |
THF |
23 |
55 |
51 |
7 |
APCW2 |
3-Methyl 3-pentanol |
19 |
47 |
82 |
8 |
APCW2 |
Amyl alcohol |
42 |
40 |
75 |
9 |
APCW2 |
Toluene |
90 |
26 |
17 |
10 |
APCW2 |
MTBE |
46 |
35 |
35 |
11 |
APCW2 |
Dioxane |
17 |
42 |
64 |
12 |
APCW3 MCC/Sub/Brij (1 : 1:1) |
3-Methyl 3-pentanol |
22 |
39 |
87 |
13 |
APCW4 MCC/Sub/Brij (3 : 1:1) |
3-Methyl 3-pentanol |
23 |
44 |
86 |
14g |
APCW4 |
3-Methyl 3-pentanol |
70 |
35 |
90 |
15h |
APCW4 |
3-Methyl 3-pentanol |
45 |
43 |
86 |
16i |
APCW4 |
3-Methyl 3-pentanol |
45 |
37 |
88 |
17 |
APCW4 |
2,4-Dimethyl-3-pentanol |
24 |
48 |
90 |
18 |
APCW5 NFC/Sub/Brij (3 : 1:1) |
3-Methyl 3-pentanol |
27 |
44 |
85 |
19 |
APCW6 MCC/Sub/Brij (3 : 1:3) |
3-Methyl 3-pentanol |
21 |
46 |
84 |
20 |
APCW6 |
2-Ethylhexanol |
20 |
0 |
ND |
21j |
APCW6 |
3-Methyl 3-pentanol |
72 |
5 |
ND |
22 |
MCC/CNC or NFC |
3-Methyl 3-pentanol |
48 |
0 |
ND |
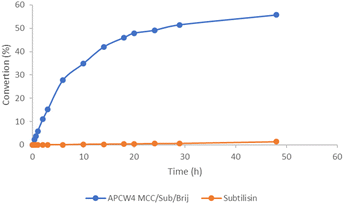 |
| Fig. 3 Monitoring the formation of amide (S)-3a as a function of time for the amidation of rac-1a using subtilisin and modified subtilisin with structural components in 2,4-dimethyl-3-pentanol. | |
Switching the cellulose source from CNC to MCC (microcrystalline cellulose) for the assembly of APCW3 gave a better result in terms of enantioselectivity. This could be due to the more robust structure of the microcrystalline cellulose that offers an extra degree of protection for the enzyme (entry 12). The tuning of the ratio between the components for the self-assembly of APCW4 led to an increase in the yield (entry 13). Lowering the temperature from 20 °C to 4 °C resulted in a slower reaction and required an increased reaction time (entry 14). Adding Na2CO3 or NaOAc as additives did not show any significant improvement (entries 15–16). Switching to 2,4-dimethyl-3-pentanol as solvent gave the best result in terms of yield and enantioselectivity (entry 17). NFC (nanofibilated cellulose) was also investigated as a polysaccharide component for the self-assembly of APCW5 (entry 18). The APCW 5-catalyzed KR of 1a gave results similar to when CNC and MCC were used as components (entries 7 and 13), which demonstrates the broad scope of the artificial plant cell wall concept.
Once the reaction condition had been optimized, the kinetic resolution of different racemic secondary amines was investigated (Scheme 1). Benzylic amines 1a–1e gave the corresponding S-amides 3a–3e in high yield and good ee. The absolute stereochemistry of the products was determined by comparison with the literature.9 Amines with an electron-withdrawing group (1c) or a methyl group (1d) in the p-position of the aromatic ring were well tolerated. Even rigid system such as 1e and aliphatic amine 1f gave the corresponding (S)-amide 3 with high ee. Bulky amine 1b bearing a naphthyl ring gave the best yield and ee. Since lifetime and recycling of heterogeneous catalysts have a key role for practical applications, we evaluated the efficiency of APCW conducting recycling experiments on the kinetic resolution of racemic 1-(1-naphthyl) ethylamine 1b. For example, APW6 was recycled 8 times without any significant loss in activity giving the corresponding (S)-3b in high yield and enantiomeric excess (Table 2).
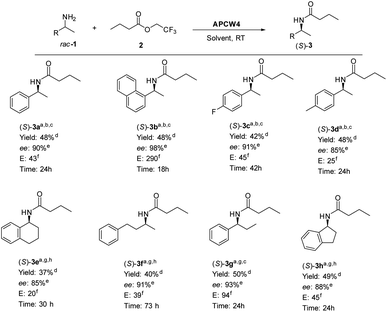 |
| Scheme 1 APCW4-catalyzed deracemization of chiral aminesa. aReaction conditions: rac-1 (0.25 mmol, 1 equiv.), 2 (0.5 mmol, 2 equiv.), solvent (1 mL), APCW4, room temperature. bAPCW4 (9 mg, 11 wt% (1 mg) subtilisin), used. c2,4-Dimethyl-3-pentanol used as solvent. dIsolated yield. eDetermined by chiral HPLC. fE = enantiomeric ratio (selectivity factor as determined by Chen et al.25). gAPCW4 (18 mg, 11 wt% (2 mg) subtilisin) used. h3-Methyl 3-pentanol used as solvent. | |
Table 2 Recycling of APCW6 for the catalytic KR of racemic 1ba
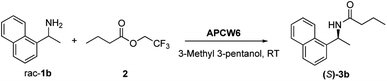
|
Cycle |
Time [h] |
Yield 3b b [%] |
ee 3b c [%] |
Ed |
Reaction conditions: 1b (0.5 mmol, 1 equiv.), 2 (1.0 mmol, 2 equiv.), 3-methyl 3-pentanol (2 mL), APCW6 (54 mg, 9 wt% (4.9 mg) subtilisin), room temperature. Isolated yield. Determined by chiral HPLC. E = enantiomeric ratio, selectivity factor as determined by Chen et al. |
1 |
22 |
39 |
96 |
259 |
2 |
22 |
41 |
95 |
206 |
3 |
22 |
51 |
94 |
170 |
4 |
22 |
42 |
97 |
235 |
5 |
22 |
46 |
96 |
194 |
6 |
23 |
45 |
96 |
175 |
7 |
22 |
42 |
96 |
133 |
8 |
22 |
38 |
98 |
208 |
9 |
27 |
38 |
98 |
203 |
10 |
43 |
40 |
97 |
151 |
Dynamic kinetic resolution is an elegant way to overcome the yield limit of 50% imposed by the kinetic resolution. For this to be achieved with great success, selective racemization of the primary amine substrate has to occur during the reaction. However, palladium-catalyzed racemization of benzylic primary amines, in the presence of hydrogen under harsh conditions, can lead to the destructive formation of side products.26 In 2005, Bäckvall disclosed that the ruthenium Shvo catalyst is able to promote in situ racemization of various types of amines under inert atmosphere.27 Bearing this in mind, we tested the racemization of enantiopure (S)-amines 1 in an unusual solvent for this transformation such as 2,4-dimethyl-3-pentanol. We found that the Shvo catalyst slowly promoted the racemization of (S)-1-(1-naphthyl) ethylamine (1b) and (S)-1-phenylethan-1-amine (1a), respectively (Table 3, entries 2–3). However, a high reaction temperature not optimal for subtilisin was required. Despite this inconvenience we decided to investigate the DKR using the Shvo catalyst from a conceptual standpoint. The APCW4/Shvo co-catalyzed system was able to catalyze a DKR in a synergistic fashion (Table 4). For example, (S)-amide 3b was synthesized in 75% yield and 76% ee using combined APCW4/Shvo co-catalysis at 90 °C (Table 4, entry 6). Thus, a DKR can be achieved but needs further development. In this context, a suitable racemization catalyst operating at lower temperatures in solvents such as 2,4-dimethyl-3-pentanol is desirable. Another option would be to improve the subtilisin catalysts enantioselectivity and thermal stability in a solvent optimal for the Shvo co-catalyst by directed evolution or protein engineering.28
Table 3 Screening for the racemization catalyzed by Shvo catalysta
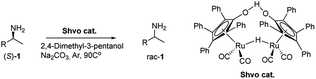
|
Entry |
R |
SHVO cat. [mol%] |
Time [h] |
eeb [%] |
Reaction conditions: 1 (0.25 mmol, 1 equiv.), 2,4-dimethyl-3-pentanol (1 mL), Shvo catalyst, Na2CO3 (0.275 mmol, 1.1 equiv.), 90 °C, Ar. Determined by chiral HPLC. |
1 |
1-Naft |
4.4 |
22 |
94 |
2 |
1-Naft |
18.4 |
23 |
74 |
3 |
Ph |
4.4 |
24 |
67 |
Table 4 Screening of APCW4/Shvo co-catalyzed dynamic kinetic resolutiona

|
Entry |
R |
SHVO catalyst [mol%] |
APCW4 [mg] |
Time [h] |
Yield 3 b [%] |
ee 3 c [%] |
Reaction conditions: 1 (0.25 mmol, 1 equiv.), 2 (0.5 mmol, 2 equiv.) 2,4-dimethyl-3-pentanol (1 mL), Shvo catalyst, APCW4, Na2CO3 (0.275 mmol, 1.1 equiv.), 90 °C, Ar. Isolated yield. Determined by chiral HPLC. Na2CO3 (0.75 mmol, 3 equiv.). |
1 |
Ph |
4.4 |
9 |
120 |
52 |
43 |
2 |
Ph |
8.8 |
9 |
92 |
44 |
44 |
3 |
Ph |
16.6 |
18 |
48 |
61 |
57 |
4 |
Ph |
— |
9 |
29 |
44 |
40 |
5 |
1-Nph |
18.4 |
18 |
71 |
69 |
70 |
6d |
1-Nph |
24 |
18 |
71 |
75 |
76 |
7d |
1-Nph |
— |
18 |
28 |
40 |
62 |
4 Conclusions
We have disclosed that the concept of artificial plant-cell wall (APCW) assembly can dramatically improve the catalytic activity of proteases for organic reactions in non-aqueous media. This was demonstrated by the kinetic resolution of several racemic primary amines to give the corresponding (S)-amides in high yields and ees. The heterogeneous APCW catalyst showed a remarkable recyclability and was used in 10 cycles without decreasing the high ee of the synthesized product amide. The combination of the APCW catalyst with a ruthenium metal catalyst allowed for tandem catalysis and for the first example of an (S)-selective dynamic kinetic resolution of a racemic primary amine with a heterogeneous hybrid enzyme/metal catalyst. Further investigation of APCW and other self-assembled heterogeneous catalyst and multi-catalyst systems based on materials from renewable resources for organic transformations and enzyme catalysis are ongoing in our laboratories.
Conflicts of interest
There are no conflicts of interest.
Acknowledgements
The authors thank the European Union, the Swedish Research Council (VR), and the Knut & Alice Wallenberg Foundation (KAW) for financial support.
Notes and references
- L. A. Nguyen, H. He and C. Pham-Huy, Int. J. Biomed. Sci., 2006, 2, 85–100 CAS.
- M. T. Reetz, J. Org. Chem., 2009, 74, 5767–5778 CrossRef CAS PubMed.
-
(a) T. Henkel, R. M. Brunne, H. Mîller and F. Reichel, Angew. Chem., Int. Ed., 1999, 38, 643–647 CrossRef CAS;
(b) A. K. Ghose, V. N. Viswanadhan and J. J. Wendoloski, J. Comb. Chem., 1999, 1, 55–68 CrossRef CAS PubMed.
-
(a) K. Engström, E. V. Johnston, O. Verho, K. Gustafson, M. Shakeri, C.-W. Tai and J.-E. Bäckvall, Angew. Chem., Int. Ed., 2013, 52, 14006–14010 CrossRef PubMed;
(b) T. Görbe, K. P. Gustafson, O. Verho, G. Kervefors, H. Zheng, X. Zou, E. V. Johnston and J.-E. Bäckvall, ACS Catal., 2017, 7, 1601–1605 CrossRef;
(c) K. P. Gustafson, T. Görbe, G. de Gonza-lo-Calvo, N. Yuan, C. L. Schreiber, A. Shchukarev, C.-W. Tai, I. Persson, X. Zou and J.-E. Bäckvall, Chem.–Eur. J., 2019, 25, 9174–9179 CrossRef CAS PubMed.
- For contributions by others to hybrid metal/enzyme catalysts for DKR of amines see:
(a) X. Li, Y. Cao, Y. Sun, J. Xiong, L. Wang, Z. Liu, J. Li, J. Ma, J. Ge, H. Xiao and R. N. Zare, Nat. Catal., 2019, 2, 718–725 CrossRef CAS;
(b) M. Filice, M. Marciello, M. P. Morales and J. M. Palomo, Chem. Commun., 2013, 49, 6876–6878 RSC;
(c) S. Dutta, N. Kumar, S. Dubbu, S. W. Jang, A. Kumar, H. Ohtsu, J. Kim, S. H. Cho and I. S. Lee, Angew. Chem., Int. Ed., 2020, 132, 3444–3450 CrossRef;
(d) S. Gao, Z. Wang, L. Ma, Y. Liu, J. Gao and Y. Jiang, ACS Catal., 2020, 10, 1375–1380 CrossRef CAS.
- N. A. M. Azrin, M. S. M. Ali, R. Rahman, S. N. Oslan and N. Noor, Biotechnol. Appl. Biochem., 2022, 1–18 Search PubMed.
- R. J. Kazlauskas and A. N. E. Weissfloch, J. Mol. Catal. B: Enzym., 1997, 3, 65–72 CrossRef CAS.
-
(a) M.-J. Kim, Y. Chung, Y. K. Choi, H. K. Lee, D. Kim and J. Park, J. Am. Chem. Soc., 2003, 125, 11494–11495 CrossRef CAS PubMed;
(b) L. Borén, B. Martín Matute, Y. Xu, A. Córdova and J.-E. Bäckvall, Chem.–Eur. J., 2006, 12, 225 CrossRef PubMed;
(c) M. J. Kim, Y. I. Chung, Y. K. Choi, H. K. Li, D. Kim and J. Park, J. Am. Chem. Soc., 2003, 125, 11494 CrossRef CAS PubMed;
(d) S. G. Martinez, E. Alvira, L. Vergara Cordero, A. Ferrer, I. Montanés-Clemente and G. Barletta, Biotechnol. Prog., 2002, 18, 1462 CrossRef PubMed;
(e) K. Kim, E. Lee, C. Kim, J. Park and M.-J. Kim, Org. Biomol. Chem., 2017, 15, 8836 RSC.
- H. Kitaguchi, P. A. Fitzpatrick, J. E. Huber and A. M. Klibanov, J. Am. Chem. Soc., 1989, 111, 3095–3096 CrossRef.
- A. L. Gutman, E. Meyer, E. Kalerin, F. Polyak and J. Sterling, Biotechnol. Bioeng., 1992, 40, 760–767 CrossRef CAS PubMed.
- A.-L. Bottalla, S. Queyroy, N. Azzi-Schue, N. Vanthuyne, S. Gastaldi, M. P. Bertrand and G. Gil, Tetrahedron: Asymmetry, 2009, 20, 2823–2834 CrossRef CAS.
- P. Falus, L. Cerioli, G. Bajnýczi, Z. Boros, D. Weiser, J. Nagy, D. Tessaro, S. Servi and L. Poppea, Adv. Synth. Catal., 2016, 358, 1608–1617 CrossRef CAS.
-
(a) T. Jesionowski, Colloids Surf., A, 2001, 190, 153–165 CrossRef CAS;
(b) T. Jesionowski and A. Krysztafkiewicz, Colloids Surf., A, 2002, 207, 49–58 CrossRef CAS.
-
(a) M. L. Foresti, G. Valle, R. Bonetto, M. L. Ferreira and L. E. Briand, Appl. Surf. Sci., 2010, 256, 1624–1635 CrossRef CAS;
(b) D. Vallés, S. Furtado, C. Villadóniga and A. M. B. Cantera, Process Biochem., 2011, 46, 592–598 CrossRef;
(c) R. Reshmi, G. Sanjaya and S. Sugunan, Catal. Commun., 2007, 8, 393–399 CrossRef CAS;
(d) Z. Yang, S. Si and C. Zhang, Microporous Mesoporous Mater., 2008, 111, 359–366 CrossRef CAS.
- J. Wang, J. Zhu and S. Wu, Appl. Microbiol. Biotechnol., 2015, 99, 4691–4700 CrossRef CAS PubMed.
-
(a) H. Horchani, I. Aissa, S. Ouertani, Z. Zarai, Y. Gargouri and A. Sayari, J. Mol. Catal. B: Enzym., 2012, 76, 125–132 CrossRef CAS;
(b) K. Vijayaraghavan, D. Yamini, V. Ambika and N. Sravya Sowdamini, Crit. Rev. Biotechnol., 2009, 29, 67–77 CrossRef CAS PubMed;
(c) A. A. Homaei, R. Sariri, F. Vianello and R. Stevanato, J. Chem. Biol., 2013, 6, 185–205 CrossRef PubMed;
(d) W. Tischer and F. Wedekind, Top. Curr. Chem., 1999, 200, 95–126 CrossRef CAS.
- P. Maksym, M. Tarnacka, A. Dzienia, K. Matuszek, A. Chrobok, K. Kaminski and M. Paluch, Macromolecules, 2017, 50, 3262–3272 CrossRef CAS.
- R. A. Sheldon, Adv. Synth. Catal., 2007, 49, 1289–1307 CrossRef.
- W. Keim, Pet. Chem., 2010, 50, 298–304 CrossRef.
- S. Rajeswari, T. Prasanthi, N. Sudha, R. P. Swain, S. Panda and S. Goka, World J. Pharm. Pharm. Sci., 2017, 6, 472–494 CAS.
- S. Tongye and S. Gnanakaran, Biophys. J., 2009, 96, 3032–3040 CrossRef PubMed.
-
(a) L. Deiana, Y. Jiang, C. Palo-Nieto, S. Afewerki, C. A. Incerti-Pradillos, O. Verho, C.-W. Tai, E. V. Johnston and A. Córdova, Angew. Chem., Int. Ed., 2014, 53, 3447–3451 CrossRef CAS PubMed;
(b) C. Palo-Nieto, S. Afewerki, M. Andersson, C.-W. Tai, P. Berglund and A. Córdova, ACS Catal., 2016, 6, 3932–3940 CrossRef CAS;
(c) L. Deiana, S. Afewerki, C. Palo-Nieto, O. Verho, E. V. Johnston and A. Córdova, Sci. Rep., 2012, 2(851), 1–7 Search PubMed.
-
(a) M.-B. Li, Y. Yang, A. A. Rafi, M. Oschmann, E. S. Grape, A. K. Inge, A. Córdova and J.-E. Bäckvall, Angew. Chem., Int. Ed., 2020, 59, 10391–10395 CrossRef CAS PubMed;
(b) A. Córdova, S. Afewerki, R. Alimo-hammadzadeh, I. Sanhueza, C.-W. Tai, S. H. Osong, P. Engstrand and I. Ibrahem, Pure Appl. Chem., 2019, 91, 865–874 CrossRef;
(c) M.-B. Li, J. Yang, Y. Yang, G.-Y. Xu, G. Luo, J. Yang and J.-E. Bäckvall, Angew. Chem., Int. Ed., 2021, 60, 670–674 CrossRef CAS PubMed.
-
(a) L. Deiana, A. A. Rafi, V. R. Naidu, C.-W. Tai, J.-E. Bäckvall and A. Córdova, Chem. Commun., 2021, 57, 8814–8817 RSC;
(b) For other bioinspired artificial heterogeneous multi-catalyst systems see: L. Deiana, A. A. Rafi, C.-W. Tai, J.-E. Bäckvall and A. Córdova, ChemCatChem, 2023, e202300250 CrossRef.
- E-values were calculated according to: C.-S. Chen, Y. Fujimoto, G. Girdaukas and C. Sih, J. Am. Chem. Soc., 1982, 104, 7294 CrossRef CAS.
- N. Parvulescu, P. A. Jacobs and D. E. De Vos, Appl. Catal., A, 2009, 368, 9–1610 CrossRef.
- J. Paetzold and J.-E. Bäckvall, J. Am. Chem. Soc., 2005, 127, 17620–17621 CrossRef CAS PubMed.
-
(a) H. Zhao and F. H. Arnold, Protein Eng., 1999, 12, 47–53 CrossRef CAS PubMed;
(b) R. Dorau, T. Görbe and M. Svedendahl Humble, ChemBioChem, 2018, 19, 338–346 CrossRef CAS PubMed;
(c) M. Dickman, R. C. Lloyd and J. B. Jones, Tetrahedron: Asymmetry, 1998, 9, 4099–4102 CrossRef CAS;
(d) For example, we investigated the use of subtilisin Pd-nanoparticles as the racemization catalyst in toluene. The Pd-nanoparticle-catalyzed racemization is fast while the subtilisin operates poorly in this solvent, which is contrary to CALB..
|
This journal is © The Royal Society of Chemistry 2023 |
Click here to see how this site uses Cookies. View our privacy policy here.