DOI:
10.1039/D3RA02471G
(Paper)
RSC Adv., 2023,
13, 21550-21557
Trace determination of disinfection by-products in drinking water by cyclic ion chromatography with large-volume direct injection
Received
13th April 2023
, Accepted 5th July 2023
First published on 18th July 2023
Abstract
A novel cyclic ion chromatography (IC) system was developed for the simultaneous determination of trace disinfection by-products (DBPs) in drinking water. Five DBPs (chlorite, bromate, chlorate, dichloroacetic acid, and trichloroacetic acid) were sensitively determined by large-volume direct injection, and the interferences of dominant inorganic anions present in water were eliminated online through the cyclic determination of the target analytes. Under optimized conditions, the obtained limits of detection (LODs) were in the range of 0.18–1.91 μg L−1 based on a signal-to-noise ratio (S/N) of 3 and an injection volume of 1.0 mL. The RSDs for peak area and retention time were in the range of 0.13–1.03% and 1.24–4.29%, respectively. Satisfactory recoveries between 92.3% and 106.4% were obtained by adding three concentration gradients of standards to the drinking water samples. The proposed method has advantages such as high sensitivity, facile automation, and no sample pretreatment, and might be a promising approach for routine analysis.
1 Introduction
Drinking water disinfection is an indispensable measure to guarantee public health, and can remove viruses, bacteria, and other micro-pollutants as well as provide purified water for human consumption.1–3 Disinfection by-products (DBPs) are a series of contaminants produced by the reaction of disinfectants (chlorine, chlorine dioxide, ozone, etc.) with natural compounds in water during the disinfection process.4 DBPs in drinking water can pose long-term health risks to humans, including potential carcinogenic, mutagenic, and reproductive toxicity, which has attracted considerable public attention.5 Chlorite, bromate, and chlorate are three typical hazardous inorganic oxyhalide DBPs, among which bromate has been identified as a potential carcinogen by the World Health Organization (WHO) and the United States Environmental Protection Agency USEPA.6,7 Haloacetic acids (HAAs) are also another group of DBPs that are detected in drinking water frequently,8 and dichloroacetic acid (DCAA) and trichloroacetic acid (TCAA) are two HAAs with the highest concentrations and carcinogenic risk in drinking water.9 Due to their widespread occurrence and potential health risks, some countries have established limit values for the content of oxyhalide DBPs and HAAs in drinking water. Therefore, it is necessary to conduct routine analysis of these DBPs in drinking water to ensure consumer health.
Multiple analytical methods have been developed for the determination of DBPs in drinking water. For example, the United States Environmental Protection Agency (USEPA) recommends using gas chromatography (GC) to detect HAAs.10 Before being injected into the GC system, the samples need to undergo pretreatment processes such as acidification, liquid–liquid extraction, and esterification derivatization, which are time-consuming and labor-intensive. Although liquid chromatography coupled with inductively coupled plasma mass spectrometry (ICP-MS) can detect bromate in water, it is limited to determining only one or two compounds, making the simultaneous determination of multiple substances challenging.11 Since chlorite, bromate, and chlorate in drinking water are present in an ionic form, and although DCAA and TCAA exist in a neutral form, their acid-dissociation coefficients (pKa) are low.12 Therefore, ion chromatography (IC) coupled with conductivity detection is a more suitable method for determining these substances.
To date, many reports have been published on the use of ion chromatography for determining oxyhalide DBPs and HAAs in drinking water.13,14 Compared with gas chromatography and ICP-MS, IC has obvious advantages such as simple sample treatment, rapid determination, and good reproducibility.15 However, due to the low concentrations of DBPs in water samples, IC with a large-volume injection method is usually adopted, which significantly improves the determination sensitivity of DBPs.16 However, this method also leads to the concentration of dominant inorganic anions (fluoride, chloride, nitrate, sulfate, etc.) present in water samples, causing interference with the DBPs determination.17 This interference is mainly eliminated by optimizing chromatographic conditions and offline pretreatment (e.g., on guard Ag pretreatment column to remove chloride ions and on guard Ba pretreatment column to remove sulfate ions) of water samples before IC injection.18 At present, there is still no universal ideal online IC technology to completely eliminate the influence of this disturbance on DBPs determination.
In this study, a novel cyclic ion chromatography system with a large-volume direct injection system was established. Automated and selective online elimination of inorganic anions was achieved by using valve-switching technology and cyclic measurement of the target DBPs. The positions of the enrichment column can be automatically changed by repeatedly switching the valve. After the first separation in the analytical column, the affected target DBPs were concentrated on the enrichment column, and a large amount of interfering components were directly discharged into the waste liquid. Then, DBPs which concentrated on the enrichment column were cut onto the analytical column again by column-switching for secondary separation. The elimination of coexisting interfering anions with high concentrations in the sample was realized by cyclic analysis of the DBPs. This method was successfully applied to the simultaneous determination of five DBPs (chlorite, bromate, chlorate, DCAA, and TCAA) in drinking water.
2 Experimental
2.1 Equipment
A Thermo Scientific Dionex ICS 2100 ion chromatograph (Sunnyvale, CA, USA) was employed in this research. It was composed of the following modules: an AS-DV auto-sampler, a dual-piston serial pump, an EG40 eluent generator, a DS6 conductivity detector, and two six-port valves. An ASRS 3000 suppressor (Thermo, Sunnyvale, CA, USA) was used for eluent suppression in the external-water mode. The analytical column was an IonPac AG19 (50 mm × 4 mm i.d., 5 μm) guard column and an IonPac AS19 (250 mm × 4 mm i.d., 5 μm) separation column. Another IonPac AG19 column (50 × 4 mm i.d., 5 μm) was used as the enrichment column. Polyether ether ketone (PEEK) tubes were used to connect all chromatographic modules, and the lengths of the connecting tubes were kept as short as possible to minimize system void volume. The cyclic IC system built with the above modules is shown in Fig. 1.
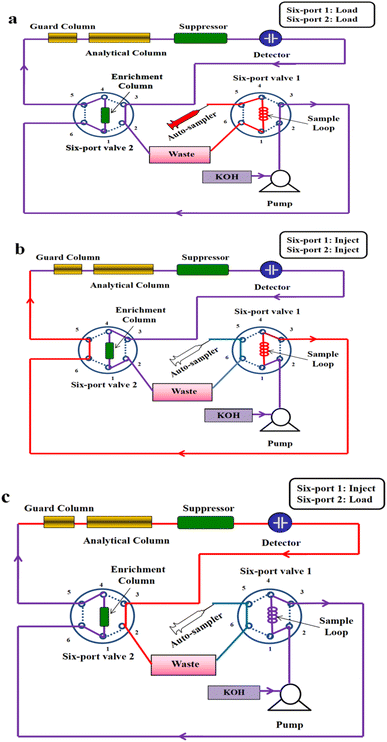 |
| Fig. 1 Chromatographic instrument configurations for the analysis of trace DBPs in drinking water. (a) System balancing and sample injecting; (b) online removal of interfering substances and collection of target components; (c) secondary analysis of target components. | |
2.2 Reagents and materials
Standard solutions, including chlorite, bromate, chlorate, DCAA, and TCAA, with a concentration of 1000 mg mL−1, were purchased from Anpu Experimental Technology Co., Ltd (Shanghai, China), respectively. Other anion standards were prepared from corresponding salts (Sinopharm Chemical Reagent Co. Ltd, China). Experimental water was obtained from a Milli-Q water purification system (Millipore, Bedford, MA, USA). Working standards were prepared by further diluting the above standards to the expected range. The solutions used in the experiment were stored in tightly sealed containers and refrigerated at 4 °C to prevent possible spoilage. Furthermore, stability tests showed that all solutions remained stable for at least three months under the storage conditions of this experiment. Water samples were filtered through a 0.45 μm membrane filter before being injected into the IC system.
2.3 Chromatographic conditions
The eluent generator was set to generate a concentration of 12 mmol per L KOH as an eluent to analyze the target compounds. The suppressor current was set at 115 mV. Data acquisition, instrument control, and the switching program of the two six-port valves were all controlled by Chromeleon 6.8 software (Dionex, USA). The positions of the enrichment column could be changed by switching the six-port valves. Correspondingly, the mobile phase of the enrichment column was also varied. In different states, the eluent flowing through the enrichment column was either KOH solution or water, which was converted from KOH liquid by the suppressor. The flow rate of the entire process was 1.0 mL min−1, and the sampling loop was set to a large-volume of 1.0 mL. The temperature of the detector cell and analytical column was 35 °C and 26 °C, respectively.
2.4 Experimental procedure
Four steps were involved in eliminating interferences of conventional anions and determining the concentrations of five DBPs: (i) loading the sample into the sample loop via an automatic sampling device, after balancing the chromatographic system; (ii) delivering the sample from the sample loop into the separation column and performing the first separation step; (iii) collecting the disturbed target components by the enrichment column and eliminating the matrix inorganic anions; and (iv) analyzing the disturbed target components for the second time by the analytical column. All these steps were achieved by controlling the cyclic IC system that we constructed in this study.
3 Results and discussion
3.1 Operation procedure of the cyclic IC system
Fig. 1 illustrates the configuration diagram of our cyclic IC system. By switching six-port valve 1 and six-port valve 2, the connection patterns of modules in the IC system can be modified to achieve different objectives. Sample injection was accomplished by switching valve 1 (Fig. 1a and b). The collection and secondary analysis of the disturbed components were mainly achieved by switching valve 2. It was worth noting the suppressor in the IC system can convert the KOH eluent to water. When valve 2 was in the “injection” position (Fig. 1b), the enrichment column was connected behind the detector cell. In this condition, water or waste liquid containing sample ions was the mobile phase which flowing through the enrichment column. As the above liquids had no elution capability, target compounds could be concentrated in the enrichment column. Due to the first separation of the analytical column, the target compounds and the interfering matrix have been separated preliminary. By switching valve 2, the disturbed target compounds were concentrated on the enrichment column, and the interfering components were discharged into the waste directly. Then, with valve 2 in the “load” position, the enrichment column was placed at the front of the guard column (Fig. 1c), and the KOH eluent was the mobile phase which flowing through the enrichment column. The components concentrated on the enrichment column can be eluted onto the analytical column under the action of KOH eluent for the secondary separation. Owing to most of the matrix have been discharged into the waste, their effects could be eliminated in the process of secondary analysis.
3.2 Selection of chromatographic parameters
Traditional IC methods for the simultaneous determination of oxyhalide DBPs and HAAs have strict requirements on parameters such as the concentration and gradient of KOH eluent, flow rate, and column temperature.19 In contrast, the chromatographic parameters of the cyclic IC in this study were relatively flexible. However, to expand the cyclic IC system applications, we optimized the IC parameters in terms of improving separation, shortening analysis time, and increasing detection sensitivity. We used a large sample loop (1.0 mL) to improve sensitivity. Meanwhile, an isocratic analysis with 12 mmol per L KOH eluent was selected, taking into account both separation efficiency and analysis time. In addition, the columns of the IC system were kept in a constant temperature environment of 26 °C to maintain the stability of the entire process. Based on the above chromatographic parameters, we also focused on optimizing the switching opportunities of the two switching valves (valve 1 and valve 2).
3.3 Interference of the matrix concentration
In this study, the influence of inorganic anion matrices on the determination of DBPs were investigated. Drinking water quality standards in China, the EU, and the US EPA all explicitly stipulate the maximum allowable concentrations of common inorganic anions in drinking water.20 The traditional IC method was used to investigate the effect of the concentration of inorganic anion matrix in water samples on the determination of target DBPs. We found that when the concentrations of fluoride and sulfate are at their maximum allowable levels (fluoride: 1.5 mg L−1, sulfate: 250 mg L−1), neither substance interferes with the determination of the five DBPs. Similarly, trace amounts of nitrite and bromide ions in drinking water do not interfere with the measurement of the target DBPs. Nevertheless, due to the lower resolution between the chromatographic peaks of chloride and DCAA, as well as nitrate and TCAA, when the concentrations of chloride and nitrate increase, they may interfere with the determination of DCAA and TCAA, respectively. As shown in Fig. 2a. When the chloride concentration exceeds 1.5 mg L−1 in the determination of drinking water by the traditional IC method, the peak area of DCAA (100 μg L−1) decreases significantly. Likewise, the peak area of TCAA (100 μg L−1) also experiences a significant decrease when the nitrate concentration exceeds 2.0 mg L−1 (Fig. 2b). Therefore, it is necessary to employ the cyclic IC system to eliminate chloride and nitrate interference online.
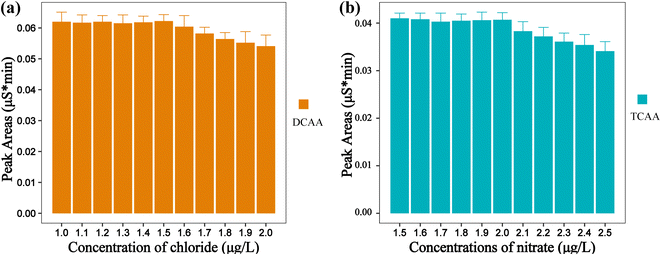 |
| Fig. 2 Effect of inorganic anion matrix content in standard solutions on the peak areas of target sustances using the traditional IC method (n = 6). (a) DCAA, 100 μg L−1; (b) TCAA, 100 μg L−1. Conditions: eluent, 12 mmol per L KOH; flow rate, 1.0 mL min−1; suppressor current, 115 mV; analytical column, IonPac AG19 + AS19; temperature of the detector cell, 35 °C; temperature of the analytical column, 26 °C; sampling volume, 1.0 mL. | |
3.4 Optimization of switching time
Table 1 illustrates the cut windows of the valves and how the system performs clearly. In this study, the cut windows of valve 2 were essential important for the entire experiment, and were strongly associated with the efficiency of matrix elimination and the accurate determination of analytes. Three cut windows of valve 2 were optimized in our study by determining actual tap water samples spiked with five DBPs standards. The first opportunity was from 0.0 min to the time (0.5 min) when the sample was completely washed onto the guard column with KOH eluent, ensuring that the sample was entirely eluted onto the analytical column for the first separation after injecting, and not concentrated on the enrichment column. At this point, under the action of the KOH eluent, fluoride, chlorite, and bromate were completely separated in the analytical column, and sequentially eluted out of the chromatographic column and then into the waste after being detected by the detector. Subsequently, the sequentially eluted species were chloride and DCAA, which could not be baseline separated from each other. Therefore, the second cut window was aimed at eliminating the interference of chloride on DCAA. When DCAA eluted from the chromatographic column (9.7 min), the valve 2 was switched, and the enrichment column was placed behind the detector cell to concentrate DCAA and some chloride ions. We found that if the enrichment column was immediately switched back to the front of the guard column after complete enrichment of DCAA, nitrate ions would interfere with the second analysis of DCAA. To avoid this situation, we set the time of enrichment column cutting back to the front of the guard column at 12.5 min. Nitrite ions were also concentrated on the enrichment column under the conditions of our optimized cut window (9.7–12.5 min) along with all DCAA and a small amount of chloride.
Table 1 System operation procedure
Time (min) |
Valve 1 |
Valve 2 |
Position of enrichment column |
Events |
−0.5–0.0 |
Load |
Load |
Before the guard column |
System balancing; sample injecting |
0.0–0.5 |
Inject |
Inject |
After the detector cell |
Washing sample onto the analytical column with KOH eluent |
0.5–9.7 |
Inject |
Load |
Before the guard column |
Separating and analyzing of components with retention weaker than DCAA |
9.7–12.5 |
Inject |
Inject |
After the detector cell |
Collecting of DCAA and nitrite online, eliminating the interfering chloride ions |
12.5–18.5 |
Inject |
Load |
Before the guard column |
Separating and analyzing of components with retention weaker than TCAA and stronger than DCAA, analyzing of DCAA by analytical column secondary |
18.5–20.1 |
Inject |
Inject |
After the detector cell |
Collecting of TCAA online, eliminating the interfering nitrate ions |
20.1–50 |
Inject |
Load |
Before the guard column |
Analyzing of TCAA by analytical column secondary, separating and analyzing of components with retention stronger than TCAA |
50–60 |
Load |
Load |
Before the guard column |
Purification chromatographic system; equilibrium chromatographic system |
To optimize the second cut window of valve 2 switching, the end time of the switching cut window was set at 12.5 min, while the start time was varied within the range of 9.3 min to 9.9 min. To ensure maximum removal of chloride, it was recommended that the start time of the cut window of valve 2 be delayed as much as possible since the time of the chloride peak is earlier than that of the DCAA. However, after 9.7 min, some of the DCAA could not be entirely collected, resulting in a sharp decrease in peak area (Fig. 3a). As a result, the optimum switching cut window of valve 2 was established at 9.7–12.5 min. Additionally, 18.5–20.1 min was selected as the third cut windows of valve 2. At this point, the DCAA, a small amount of chloride, and nitrite ions concentrated on the enrichment column in the previous stage have been completely eluted out and analyzed for the second time by the analytical column before being discharged into the waste. As shown in Fig. 3b, 18.5 min was the optimal start time for the third cut window. Under the optimized cut window of 18.5–20.1 min, the TCAA was completely concentrated on the enrichment column while minimizing the presence of nitrate matrix as much as possible.
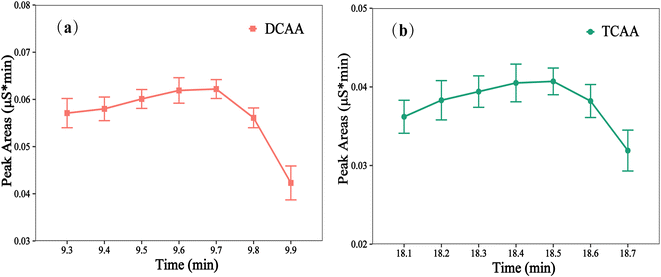 |
| Fig. 3 Effect of the switching time of valve 2 on the peak areas of target substances using the cyclic IC method (n = 6). (a) DCAA, 100 μg L−1; (b) TCAA, 100 μg L−1. Conditions: eluent, 12 mmol per L KOH; flow rate, 1.0 mL min−1; suppressor current, 115 mV; analytical column, IonPac AG19 + AS19; enrichment column, IonPac AG19; temperature of the detector cell, 35 °C; temperature of the analytical column, 26 °C; sampling volume, 1.0 mL. | |
Fig. 4 displays the representative chromatogram of actual tap water samples spiked with 5 DBPs standards. As shown in Fig. 4, Chlorite (peak a), bromate (peak b), and chlorate (peak d) were not affected by the coexisting inorganic anions, and they could be directly quantified by the first separation of analytical column. Nevertheless, DCAA (peak c) and TCAA (peak e) were obviously interfered by the tail peaks of chloride and nitrate, respectively. Under the optimized switching windows, the above interferences were eliminated, and baseline separation was achieved (peak c′ and e′) by cycle analysis.
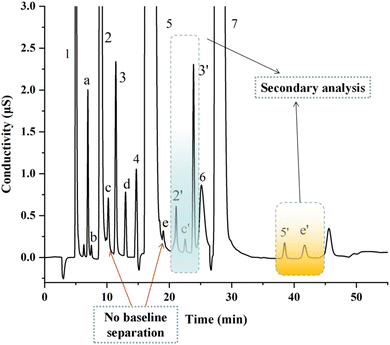 |
| Fig. 4 Chromatogram of actual tap water samples spiked with 5 DBPs standards. Peaks for first analysis (add levels): 1 = fluoride; 2 = chloride; 3 = nitrite; 4 = bromide; 5 = nitrate; 6 = carbonate; 7 = sulfate; a = chlorite (0.1 mg L−1); b = bromate (0.02 mg L−1); c = DCAA (0.1 mg L−1); d = chlorate (0.1 mg L−1); e = TCAA (0.1 mg L−1); peaks for secondary analysis: 2′ = chloride; c′ = DCAA (0.1 mg L−1); 3′ = nitrite; 5′ = nitrate; e′ = TCAA (0.1 mg L−1). | |
3.5 Analytical performances
Under the above optimized conditions, five standard solutions containing DBPs at various concentrations ranging from 5.00–100 μg L−1 (1.0–20 for bromate) were analyzed. Each target DBPs exhibited satisfactory linearity within the studied range, with all determination coefficients R ≥ 0.9991. The limits of detection (LODs) and limits of quantification (LOQs), calculated by injecting a 1.0 mL volume of a standard solution with a concentration of 5.0 μg L−1 (bromate: 1.0 μg L−1) and based on signal-to-noise ratios (S/N) of 3 and 10, were calculated to be in the range of 0.18–1.91 μg L−1 and 0.60–6.37 μg L−1, respectively. The precision results of the cyclic IC method were obtained by calculating the relative standard deviation (RSD) values for 6 repetitive injections of 5.0 μg L−1 (1.0 μg L−1 for bromate) standard solutions. The RSD for peak area and retention time ranged from 0.13–1.03% and 1.24–4.29%, respectively. All analytical performances of the proposed method are listed in Table 2.
Table 2 Calibration parameters (five points) for the DBPs in standard solutions (n = 6)
Analytes |
Linear range (μg L−1) |
Determination coefficient (R) |
LODsa (μg L−1) |
LOQsb (μg L−1) |
RSD (%) |
Retention time |
Peak area |
LODs: limits of detection. LOQs: limit of quantification. |
Chlorite |
5.00–100 |
0.9992 |
0.18 |
0.60 |
0.13 |
1.24 |
Bromate |
1.00–20.0 |
0.9991 |
0.38 |
1.27 |
0.25 |
2.76 |
DCAA |
5.00–100 |
0.9991 |
0.59 |
1.97 |
0.54 |
3.05 |
Chlorate |
5.00–100 |
0.9995 |
0.43 |
1.43 |
0.22 |
2.17 |
TCAA |
5.00–100 |
0.9990 |
1.91 |
6.37 |
1.03 |
4.29 |
This method was applied for the simultaneous determination of five DBPs in actual drinking water. The anion matrix in the samples has no interference with the determination of target analytes by using the cyclic IC system. Spiked-recovery experiments with three concentration levels were also performed using three typical samples (two tap water and one mineral water) to determine the accuracy of the method. As shown in Table 3, the method had spiking recovery rates of 92.3–105.3% with an RSD of 1.80–3.92% at the low concentration level, 94.8–106.4% with an RSD of 0.95–3.54% at the medium concentration level, and 95.3–102.7% with an RSD of 0.61–3.77% at the high concentration level. These results were satisfactory for trace analysis. Therefore, the complete resolution of chromatographic peaks and accurate quantification of five DBPs with high concentration of anion matrix in drinking water were achieved by the cyclic IC method.
Table 3 Data on analysis of real samples and spiked recoveries of five DBPs
Analytes |
Original (μg L−1) |
Low level |
Medium level |
High level |
Add level (μg L−1) |
Recovery (%) |
RSD (%) |
Add level (μg L−1) |
Recovery (%) |
RSD (%) |
Add level (μg L−1) |
Recovery (%) |
RSD (%) |
ND: not detected (lower than the limit of detection). |
Sample 1 (tap water) |
Chlorite |
NDa |
5.00 |
97.4 |
2.13 |
20.0 |
98.3 |
1.22 |
50.0 |
100.3 |
2.42 |
Bromate |
ND |
1.00 |
98.3 |
3.04 |
4.00 |
97.6 |
1.74 |
10.0 |
98.5 |
1.83 |
DCAA |
10.1 |
5.00 |
96.6 |
3.47 |
20.0 |
100.2 |
0.96 |
50.0 |
99.7 |
1.65 |
Chlorate |
ND |
5.00 |
105.3 |
1.91 |
20.0 |
99.4 |
2.41 |
50.0 |
101.4 |
0.61 |
TCAA |
15.2 |
5.00 |
94.7 |
3.29 |
20.0 |
95.2 |
3.54 |
50.0 |
96.2 |
2.76 |
![[thin space (1/6-em)]](https://www.rsc.org/images/entities/char_2009.gif) |
Sample 2 (tap water) |
Chlorite |
12.6 |
5.00 |
97.8 |
1.80 |
20.0 |
99.4 |
1.44 |
50.0 |
102.7 |
0.80 |
Bromate |
ND |
1.00 |
97.6 |
2.43 |
4.00 |
100.6 |
1.26 |
10.0 |
99.3 |
2.62 |
DCAA |
ND |
5.00 |
102.1 |
2.46 |
20.0 |
98.3 |
1.78 |
50.0 |
98.1 |
1.14 |
Chlorate |
9.26 |
5.00 |
99.4 |
2.29 |
20.0 |
106.4 |
2.61 |
50.0 |
97.4 |
2.35 |
TCAA |
ND |
5.00 |
94.7 |
2.72 |
20.0 |
96.1 |
3.10 |
50.0 |
95.9 |
3.77 |
![[thin space (1/6-em)]](https://www.rsc.org/images/entities/char_2009.gif) |
Sample 3 (mineral water) |
Chlorite |
ND |
5.00 |
96.4 |
2.41 |
20.0 |
99.4 |
2.33 |
50.0 |
100.4 |
1.46 |
Bromate |
2.41 |
1.00 |
97.0 |
1.93 |
4.00 |
98.9 |
2.12 |
10.0 |
98.6 |
0.82 |
DCAA |
ND |
5.00 |
93.6 |
2.75 |
20.0 |
95.6 |
0.95 |
50.0 |
97.9 |
1.84 |
Chlorate |
ND |
5.00 |
96.1 |
3.17 |
20.0 |
99.4 |
1.53 |
50.0 |
101.6 |
2.64 |
TCAA |
ND |
5.00 |
92.3 |
3.92 |
20.0 |
97.5 |
2.39 |
50.0 |
95.3 |
3.69 |
3.6 Methods comparison
To evaluate the applicability of the cyclic IC method for the determination of trace DBPs, we compared the method used in this study with IC methods reported in the literature in terms of method performance and the greenness level. As shown in Table 4, the LODs of the cyclic IC and the literature methods were both in the μg L−1 level. The mobile phase used in the cyclic IC was a KOH solution, which resulted in a lower background conductivity value of the chromatogram baseline than an IC system using Na2CO3 solution as the eluent. Consequently, the LODs of the cyclic IC system were relatively lower. Additionally, the cyclic IC method demonstrated better quantification precision than the literature methods. This is due to the elimination of matrix ions through cyclic analysis, enabling accurate quantification of the target analytes. It should be noted that the retention time precisions of DCAA and TCAA were slightly reduced in the cyclic IC system. In terms of analysis time, the cyclic IC method required a longer duration than the literature methods. Thus, by using two assessment tools (analytical eco-scale and GAPI) to evaluate the level of greenness of the developed method27,28 (Table 5), the cyclic IC system consumed more solvents, reagents and energy than the conventional methods. However, due to the use of a low concentration of KOH solution as the mobile phase instead of organic solvents, the waste generated by the cyclic IC system was relatively less hazardous to the health of the operator and the environment. As a result, the eco-scale assessment shows that both cyclic ion chromatography and conventional IC methods are environmentally friendly. The main advantage of the cyclic IC method, as assessed by GAPI, is that the samples do not require complex pretreatment and can be injected directly into the system for automated analysis. This reduces the time and cost associated with sample pretreatment and manual operation. Overall, the cyclic IC method outperforms traditional IC methods in terms of performance indicators and level of automation. Therefore, this method can be utilized as a green routine approach for the daily detection of water samples.
Table 4 Comparative data for the determination of DBPs by ion chromatography methods
Analytes |
Column(s) |
Eluent |
Flow rate (mL min−1) |
Analysis time (min) |
Detector |
Quantification precision (RSD, %) |
LODs (μg L−1) |
Ref. |
BrO3−, ClO3− |
Metosep A Dual 1 |
1 mmol per L ortho-phthalic acid, 2% MeCN |
1.0 |
15 |
UV/Vis |
1.31–2.06 |
5.2–10 |
21 |
BrO3−, ClO2−, ClO3−, Br− |
IonPac AS 19-HC + AG 19-HC |
9 mmol per L Na2CO3 |
1.3 |
25 |
Conductivity |
0.54–8.81 |
μg L−1 levels |
22 |
UV/Vis |
ClO2−, BrO3−, ClO3−, ClO4− |
IonPac AS 20 + AG 20 |
5–100 mmol per L NaOH |
0.375 |
18 |
Conductivity |
3.49–6.78 |
2–27 |
23 |
ClO3−, NO2− |
IonPac AS 19 + AG 19 |
KOH gradient |
1 |
>30 |
Conductivity |
<2 |
2.2 |
24 |
BrO3−, ClO2−, ClO3−, Br− |
IonPac AS 19-HC + AG 19-HC |
9 mmol per L Na2CO3 |
0.4 |
25 |
Conductivity |
0.10–3.66 |
1.32–2.55 |
25 |
BrO3−, ClO2−, I− |
IonPac AS 19-HC |
9 mmol per L Na2CO3 |
1.1 |
6.5 |
UV/Vis |
0.66–4.60 |
μg L−1 levels |
26 |
ClO2−, BrO3−, DCAA, ClO3−, TCAA |
IonPac AS 19 + AG 19 |
12 mmol per L KOH |
1.0 |
60 |
Conductivity |
0.13–1.03 |
0.18–1.91 |
This study |
Table 5 Greenness assessment of the proposed method and traditional method according to analytical eco-scale and GAPI
Eco-scale assessment |
GAPI assessment |
Category |
Description (per sample) |
Category |
Description (per sample) |
Cyclic IC system |
Traditional IC method21–26 |
Cyclic IC system |
Traditional IC method21–26 |
Reagents |
Sample preparation |
Reagents (g) |
28.8 mg NaOH |
6.20–23.9 mg Na2CO3/3.6–72 mg NaOH |
(1) Collection; (2) preservation; (3) transport; (4) storage |
(1) Off line; (2)—; (3)—; (4) normal conditions |
(1) Off line; (2)—; (3)—; (4) normal conditions |
Water (mL) |
60 |
15–30 |
(5) Type of method: direct or indirect; (6) scale of extraction; (7) solvents/reagents used; (8) additional treatments |
(5) Direct; (6) no; (7) no; (8) no |
(5) Indirect; (6) no; (7) no; (8) pretreatment |
Instrumentation |
Reagents and solvents |
Energy (W h) |
150 |
37.5–75 |
(9) Amount; (10) health hazard; (11) safety hazard |
(9) 28.8 mg NaOH + 60 mL water; (10) low; (11) safe |
(9) 6.20–23.9 mg Na2CO3/3.6–72 mg NaOH + 15–30 mL water; (10) low; (11) safe |
Occupational safety |
Safe |
Safe |
Instrumentation |
Hazard |
Low |
Low |
(12) Energy (W h) |
(12) 150 |
(12) 37.5–75 |
Waste (mL) |
60 |
15–30 |
(13) Occupational hazard |
(13) Safe |
(13) Safe |
Total comment |
Green method |
Green method |
(14) Waste; (15) waste treatments |
(14) 60 mL; (15) no treatment |
(14) 15–30 mL; (15) no treatment |
4 Conclusions
In this study, a novel cyclic IC method has been proposed based on valve switching technology. This method achieved the simultaneous determination of five trace DBPs (chlorite, bromate, DCAA, chlorate, and TCAA) in drinking water through large-volume injection. Meanwhile, interferences from chloride and nitrate in the drinking water samples were eliminated online by the cyclic determination of DCAA and TCAA, respectively. Under optimal conditions, the proposed method showed good accuracy, precision, and linearity over a wide range of concentrations. Compared to traditional IC methods, drinking water samples can be injected directly into the cyclic IC system for analysis without pretreatment. Therefore, the cyclic IC method can be a promising alternative for the determination of trace DBPs in drinking water. Additionally, the method can be applied as an online matrix elimination technique to determine trace substances in various samples containing high concentrations of salt matrices.
Author contributions
Haibao Zhu: writing – original draft, methodology, formal analysis. Zheng Ruan: data curation, formal analysis, investigation. Han Wang: methodology, project administration, writing – review and editing. Danhua Liu: supervision, writing – review and editing. Hongfang Tang: writing – review and editing. Jiahong Wang: methodology, writing – review and editing, supervision.
Conflicts of interest
The authors declare that they have no conflicts of interest.
Acknowledgements
This work was supported by the Basic Scientific Research Project of Hangzhou Medical College (YS2021006), Medical and Health Projects of Zhejiang Province, China (2022PY049), and Basic Public Welfare Research Project of Zhejiang Province, China (LGC20B050001).
References
- J. Wei, B. Ye, W. Wang, L. Yang, J. Tao and Z. Hang, Sci. Total Environ., 2010, 408, 4600–4606 CrossRef CAS PubMed
. - F. Dong, Z. Pang, J. Yu, J. Deng, X. Li, X. Ma, A. M. Dietrich and Y. Deng, J. Hazard. Mater., 2022, 423, 127113 CrossRef CAS PubMed
. - M. R. Khan, M. S. Samdani, M. Azam and M. Ouladsmane, J. King Saud Univ., Sci., 2021, 33, 101408 CrossRef
. - Z. Li, G. Song, Y. Bi, W. Gao, A. He, Y. Lu, Y. Wang and G. Jiang, Environ. Sci. Technol., 2021, 55, 4103–4114 CrossRef CAS PubMed
. - U. von Gunten, Water Res., 2003, 37, 1469–1487 CrossRef CAS PubMed
. - D. Tao, R. Wang, S. Shi, L. Yun, R. Tong, Y. e. Peng, W. Guo, Y. Liu and S. Hu, Sci. Total Environ., 2020, 740, 139888 CrossRef CAS PubMed
. - M. Ye, P. N. Nesterenko, Y. Lu, X. Huang, X. Jin and M. Chen, J. Chromatogr. Sci., 2021, 59, 217–222 CAS
. - E. E. Chang, Y. P. Lin and P. C. Chiang, Chemosphere, 2001, 43, 1029–1034 CrossRef CAS PubMed
. - C. Y. Yang, Y. P. Hang and X. L. Zhong, Chin. J. Anal. Chem., 2007, 35, 1647–1650 Search PubMed
. - USEPA, Method 552, Environmental Monitoring and System Laboratory, Cincinnati, OH, 1990 Search PubMed
. - E. S. Gilchrist, D. A. Healy, V. N. Morris and J. D. Glennon, Anal. Chim. Acta, 2016, 942, 12–22 CrossRef CAS PubMed
. - D. Martínez, J. Farré, F. Borrull, M. Calull, J. Ruana and A. Colom, J. Chromatogr., 1998, 808, 229–236 CrossRef
. - S. Cheng, Y.-P. Wu, T. R. Young, M. C. Dodd, J. Wu, H. Zhang, Z.-L. Huo, Y.-T. Qian, Y. Li, W.-T. Li and A.-M. Li, Sci. Total Environ., 2021, 754, 142297 CrossRef CAS PubMed
. - H. B. Teh and S. F. Y. Li, J. Chromatogr., 2015, 1383, 112–120 CrossRef CAS PubMed
. - Y. Liu and S. Mou, Microchem. J., 2003, 75, 79–86 CrossRef CAS
. - R. Michalski, LC-GC Eur., 2011, 24, 244–250 CAS
. - R. Michalski, Pol. J. Environ. Stud., 2005, 14, 257–268 CAS
. - H. Dong, K. Xiao, Y. Xian, Y. Wu and L. Zhu, Food Chem., 2019, 270, 196–203 CrossRef CAS PubMed
. - S. A. Snyder, B. J. Vanderford and D. J. Rexing, Environ. Sci. Technol., 2005, 39, 4586–4593 CrossRef CAS PubMed
. - J. Fu, C.-H. Huang, C. Dang and Q. Wang, Chin. Chem. Lett., 2022, 33, 4495–4504 CrossRef CAS
. - M. El Haddad, R. Mamouni, M. Ridaoui and S. Lazar, J. Saudi Chem. Soc., 2015, 19, 108–111 CrossRef
. - H. P. Wagner, B. V. Pepich, D. P. Hautman and D. J. Munch, US EPA Method 317.0, 2001 Search PubMed
. - J. P. Hutchinson, C. Johns, M. C. Breadmore, E. F. Hilder, R. M. Guijt, C. Lennard, G. Dicinoski and P. R. Haddad, Electrophoresis, 2008, 29, 4593–4602 CrossRef CAS PubMed
. - N. Wang, R. Q. Wang and Y. Zhu, J. Hazard. Mater., 2012, 235–236, 123–127 CrossRef CAS PubMed
. - J. D. Pfaff, D. P. Hautman and D. J. Munch, US EPA Method 300.1, 1997 Search PubMed.
- V. M. Matsis and E. C. Nikolaou, US EPA Method 326.0, Desalination, 2008, 224, 231e239 CrossRef
. - D. Moema, T. A. Makwakwa, B. E. Gebreyohannes, S. Dube and M. M. Nindi, J. Food Compos. Anal., 2023, 117, 105131 CrossRef CAS
. - H. M. Hafez, S. El Deeb, M. Mahmoud Swaif, R. Ismail Ibrahim, R. Ali Kamil, A. Salman Abdelwahed and A. Ehab Ibrahim, Microchem. J., 2023, 185, 108262 CrossRef CAS
.
|
This journal is © The Royal Society of Chemistry 2023 |
Click here to see how this site uses Cookies. View our privacy policy here.