DOI:
10.1039/D3RA02887A
(Paper)
RSC Adv., 2023,
13, 24333-24342
The development of ZnO nanoparticle-embedded graphitic-carbon nitride towards triple-negative breast cancer therapy†
Received
1st May 2023
, Accepted 28th July 2023
First published on 14th August 2023
Abstract
The present study deals with the effects of curcumin-loaded ZnO nanoparticles (NPs) embedded in graphitic-carbon nitride (g-C3N4) sheets for breast cancer cells. The synthesis of these sheets was carried out by a simple co-precipitation method. The physicochemical and thermal properties of the composite sheets were studied using various characterization techniques. The powder X-ray diffraction and high-resolution transmission electron microscopy analyses confirmed the hexagonal wurtzite phase of the ZnO nanoparticles, which were randomly distributed on the g-C3N4 nanosheets, generating a finely bonded interface between the two components. The X-ray photoelectron spectroscopy analysis confirmed the successful formation of the g-C3N4@ZnO composite, while the thermal studies revealed the thermal stability of the composite. In addition, the drug release and kinetics studies proved that the release of curcumin was more significant under acidic conditions (pH 5) compared with neutral pH (7.4). Further, the biological assays verified the antibacterial activity (against two different cultures of E. coli and S. aureus) and anticancer activity (against MDA-MB-231 cancer cells) of the g-C3N4@ZnO/C nanocomposite. Finally, the lactate dehydrogenase activity assay presented the cytotoxic assessment of the nanocomposite based on its cytoplasmic activity and the extent of enzymes released from the damaged cells.
1. Introduction
Recent developments in synthesis procedures and characterization techniques have unmasked the active role of many different nanomaterials over a range of biomedical applications, including drug delivery, bioimaging, sensor-based diagnosis, and externally controlled therapy.1–5 Among the nanomaterials currently applied in the biomedical sector, two-dimensional (2D) layered NPs have gained the most interest because of their unique physicochemical properties linked to their high and efficient surface area along with biocompatibility.6–8 Typical examples of this type of nanomaterial are graphene and its oxide derivative (graphene oxide; GO), which have already been explored in multiple biological applications, such as DNA detection,9,10 cell imaging,11 drug delivery,12 photodynamic13,14 and photothermal therapy.15 Considering such a strong background of graphene and its derivatives, researchers have developed graphitic carbon nitride (g-C3N4) with extraordinary features like semiconductivity, favourable electronic structure, chemical and thermal stability, visible light activity, and cost-effectiveness.16,17 g-C3N4 serves as an active photocatalyst on account of its ∼2.7 eV bandgap, enabling the effective excitation of electrons under visible-light irradiation.18 The quantum efficiency of g-C3N4 is similar to various other photocatalysts in the single-component category and can get degraded easily by the rapid recombination of photo-generated electrons and holes.19 This can result in the development of photochemically active and stable g-C3N4-mediated catalysts and hence deserves thorough investigation.
In order to enhance the efficiency of g-C3N4 and overcome its few limitations, researchers have developed heterogeneous composites by the coupling of NPs with g-C3N4. Nanoparticles are known to be effective in cancer diagnosis and treatment, as they have high surface-to-volume ratios, and the surface is enriched with sites of high coordination and unsaturation.20 It has been reported that certain metal oxide nanoparticles can destroy cancer cells selectively without harming normal cells.21 Specifically, ZnO nanoparticles are reported to have high potential in treating cancer due to their intrinsic hydrophilic property. Leelavathi et al. reported ZnO@g-C3N4 with antiproliferative activity against human cervical cancer.22 Curcumin has significant cancer-suppressive efficiency in vitro and in vivo against the progression of several cancers at all stages, including initiation, promotion, and propagation. Previous reports have demonstrated that curcumin-loaded ZnO nanoparticles show promising results against the rhabdomyosarcoma RD cell line.23 A recent report investigated the cytotoxicity of the ZnO–curcumin nanodispersion against HT-29 cancer cells.24 Yu Xia et al. reported the anticancer performance of curcumin-loaded-gelatin nanoparticles against MCF-7 cells.25
A heterogeneous composite of g-C3N4 and ZnO NPs was reported to demonstrate significantly improved photoabsorption and catalytic activity.26 Therefore, its synthesis has gained importance, and several methods of g-C3N4/ZnO composite synthesis have been introduced viz. two-step chemisorption method by Wang et al.,27 ball milling method by Liu et al.,28 calcination method by Zhu et al.,29 reflux method by Wang et al.,30 and reflux-vapour condensation method by Park et al.31 Among them, the reflux and reflux-vapor condensation methods resulted in composites with superior architectures, namely the core–shell type g-C3N4/ZnO nanocomposite (for improved photoelectrocatalytic performance) and 1D nanorods-coated g-C3N4 sheets at 0.12 mA cm−2 photocurrent density, respectively.32,33 It has been observed that the synthesis procedure plays a crucial role in the formation of well-established heterojunctions between the outer layers of ZnO NPs and the g-C3N4 sheets, which in most cases contribute to the superior photocatalytic property.31 Nevertheless, most of the synthesis procedures employed for the formation of g-C3N4/ZnO nanocomposites are not economically feasible, environment-friendly, and require multiple steps along with many by-products that are of no use. Considering all the issues associated with the formation of the g-C3N4/ZnO nanocomposite, we have attempted an in situ approach in this work toward the generation of ZnO NPs-embedded g-C3N4 (g-C3N4@ZnO). The formed composite was studied thoroughly for physicochemical and surface properties using different characterization techniques. Further, the drug release kinetic models of the curcumin-loaded g-C3N4@ZnO (termed as g-C3N4@ZnO/C) nanocomposite were obtained by studying the changes over time at two different pH conditions (5 and 7.4). Finally, the biological activity of the g-C3N4@ZnO composite was evaluated by the in vitro cancer cell viability and lactate dehydrogenase (LDH) assays, in addition to antibacterial activity tests against two different bacterial cultures.
2. Experimental details
2.1 Materials and methods
Zinc acetate dihydrate (Zn(OAc)2·2H2O), melamine, sodium hydroxide (NaOH), and curcumin of analytical reagent (AR) grade were purchased from Sigma-Aldrich (Mumbai, India). The breast cancer cell line, MDA-MD-231, of human origin was obtained from National Centre for Cell Science (NCCS), Pune, India. Dulbecco's Modified Eagle Medium (DMEM), fetal bovine serum (FBS), trypsin–EDTA, 3-(4,5-dimethylthiazol-2yl)-2,5-diphenyltetrazolium bromide (MTT), dimethyl sulphoxide (DMSO), sodium bicarbonate, sodium pyruvate, nicotinamide adenine dinucleotide (NADH), and the penicillin–streptomycin (Pen–Strep) antibiotic solution were purchased from Hi-Media Laboratories (Mumbai, India).
2.2 Fabrication of g-C3N4@ZnO nanocomposite
For the preparation of the g-C3N4@ZnO nanocomposite by an in situ route, 50 mL of a 1 M zinc acetate dihydrate solution was added to 1 g melamine first, followed by 50 mL of a 2 M NaOH solution in a dropwise manner. The solution mixture was stirred continuously until a high amount of white slurry was observed, indicating melamine–zinc hydroxide mixture formation. Stirring was continued for another 18 h, and then, the precipitate was filtered, washed several times with distilled water, and centrifuged to obtain the ZnO NPs as the product. The obtained ZnO NPs were dried in a hot air oven maintained at 100 °C in order to remove the moisture/water, ground to a fine powder, and finally sintered at 550 °C (at a 5 °C min−1 heating rate). The idea of increasing the sintering temperature to 550 °C is based on the fact that melamine undergoes a polycondensation reaction at this stage to form g-C3N4, and at the same time, the intermediate zinc hydroxide gets converted into ZnO NPs, further allowing the in situ generation of g-C3N4@ZnO.
2.3 Drug loading
For loading curcumin (drug) in g-C3N4@ZnO, we started with a formulation consisting of the carrier (g-C3N4@ZnO) and the drug at a weight ratio of 90
:
10; this sample was prepared by stirring the drug and carrier in the ethanol solvent for 1 h, and the solvent was removed by vacuum drying to obtain curcumin-loaded g-C3N4@ZnO (g-C3N4@ZnO/C) as the final product. To investigate the drug loading efficiency of g-C3N4@ZnO, 1 g of g-C3N4@ZnO/C in the dried form was dispersed in 200 mL of PBS (phosphate-buffered saline; pH 7.4), and absorbance was recorded at the absorption wavelength of 418 nm as a function of time using a UV-visible spectrophotometer.
2.4 Studies of curcumin drug release
The drug release kinetics was studied at two different physiological pH values 7.4 and 5 using the same UV-visible spectrophotometer set at an absorption wavelength of 418 nm. For the analysis, 1 g of g-C3N4@ZnO/C dispersed in 200 mL of PBS was placed on a shaking incubator at a temperature of 37 °C, and the shaking velocity was maintained at 100 rpm. At each specified time interval, about 1 mL of PBS solution was removed and replaced with fresh medium before the optical dispersion (OD) was recorded. The calibration plot was used to estimate the concentration of curcumin released into the buffer solution. The data shown indicate the mean ± SD (standard deviation) of three individual experiments.
2.5 Studies of antimicrobial activity
The antimicrobial activity assay for the g-C3N4@ZnO/C composite was performed against two different bacterial cultures, namely Gram-positive Staphylococcus aureus (S. aureus) and Gram-negative Escherichia coli (E. coli), using the agar diffusion method. For the test, the cultures were first grown overnight, and the inoculum sizes were adjusted to 0.5 using the McFarland standard. This inoculum was spread in all directions on the agar plate using a fresh-sterile swab and then subjected to room-temperature drying. The next step involved making some punctures in the agar gel using a sterile puncturing tool followed by the addition of discs containing the tested sample (g-C3N4@ZnO/C) at various concentrations on the agar medium with the help of sterile forceps. In this study, 30 mg mL−1 each of chloramphenicol and amoxycillin were selected as the positive controls for the microbial cultures of S. aureus and E. coli, respectively. All the cultures were incubated for a 24 h period at 37 °C, and at the incubation time, the diameter of the zone of inhibition (ZOI) and minimum inhibitory concentration (MIC) were recorded. The ZOI and MIC of g-C3N4@ZnO/C correspond to growth inhibition at a specified concentration and the lowest concentration that can inhibit the visible growth of the bacterial strain, respectively.
2.6 In vitro cancer cell viability assay
To investigate the anticancer activity of the g-C3N4@ZnO/C composite, in vitro cell viability studies were performed using the MTT assay using MDA-MB-231 human adenocarcinoma cells. Briefly, the cells were first seeded at a volume of 5 × 103 cells per mL in 96-well plates and were allowed to grow in a growth medium (DMEM supplemented with 10% FBS and 1% Pen–Strep solution) under 5% CO2 and 95% O2 at 37 °C. At the end of a 24 h incubation period, the cell medium was removed; the cells were washed with PBS two times, and fresh medium containing different concentrations (1, 5, 50, 100, 250 μg mL−1) of the g-C3N4@ZnO/C composite was added. Similarly, after incubation for 24 h, the sample-treated cells were subjected to medium removal two times PBS wash, and the addition of fresh medium containing 10 μL of the MTT reagent. After incubation for another 3 h, the supernatant was decanted from each well; 100 μL DMSO was added so as to dissolve the formazan crystals, if formed, by gently pipetting 2–3 times and finally, the absorbance at 570 nm was measured using a microplate reader. The following eqn (1) was used to calculate the growth inhibition rate of the g-C3N4@ZnO/C composite. |
 | (1) |
2.7 Lactate dehydrogenase (LDH) activity assay
The mechanistic action of the g-C3N4@ZnO/C composite in the MDA-MB-231 cancer cells was investigated by measuring the extent of LDH released from the cells following sample treatment. Similar to the cell viability assay, the MDA-MD-231 cells (1 × 105 cells per well) in a 96-well plate were treated with different concentrations of the g-C3N4@ZnO/C composite (1–250 g mL−1) for a 24 h period. At the end of incubation, the cell medium was collected, centrifuged for 10 min at 5000 rpm, and the pellet obtained was dissolved in a solution mixture consisting of 100 μL culture media, 100 μL sodium pyruvate (2.5 mg mL−1 of PBS), and 100 μL reduced NADH (2.5 mg mL−1 of PBS) and made up to a total volume of 3 mL using the 0.1 M potassium phosphate buffer (pH 7.4). Finally, the LDH content (IU L−1) in the culture medium was quantified by recording the speed of NADH oxidation at 340 nm for 3 min at 30 s intervals using a Shimadzu UV-vis spectrophotometer.
3. Results and discussion
Fig. 1(a) shows the Fourier Transform-Infra Red (FT-IR) spectrum, which reveals the functional groups of the g-C3N4@ZnO composite. The characteristics peaks observed at 1637 and 1243 cm−1 can be linked to the C
N and C–N stretching vibrations originating from the g-C3N4 molecules,31 while the in-plane bending vibration was observed at 881 cm−1.29 Moreover, the additional peaks related to the individual components of ZnO and g-C3N4 are attributed to the successful formation of the g-C3N4/ZnO composite. Similarly, the powder X-ray diffraction (XRD) pattern of the g-C3N4@ZnO composite shown in Fig. 1(b) represents the distinct peaks of g-C3N4 at 2θ = 27.4° corresponding to the (100) diffraction plane, confirming the in-plane packing motif, while the other peak at 35° (002) confirms the stacking structure.34 The appearance of a single peak related to the g-C3N4 compound indicates that no impurities or by-products were formed during the synthesis process. In addition, the observation of distinct diffraction peaks for the ZnO compound is in good agreement with the JCPDS data 79-2205 and corresponds with the hexagonal structure, while the orderly arrangement of the peaks supports the high crystalline nature of ZnO. Hence, the X-ray crystalline diffraction data confirmed the formation of the g-C3N4@ZnO composite in a highly crystalline form with very high purity. The scanning electron microscopy (SEM) and high-resolution transmission electron microscopy (HR-TEM) analyses of the g-C3N4@ZnO nanocomposite depicted in Fig. 1(c) and (d), respectively, indicate that the morphology of the g-C3N4 and ZnO components appeared as sheets and rod-shaped, respectively. These structures also suggest that the ZnO nanorods were embedded within the g-C3N4 sheets, as visible in Fig. 1(c). Moreover, the HR-TEM image of ZnO provided in Fig. 1(d) represents the characteristic rod-shaped morphology corresponding to the hexagonal crystalline plane (001) (as indicated by the SAED pattern shown in the inset).34 Further, the HRTEM analysis of g-C3N4@ZnO confirmed the successful embedding of the ZnO nanorods inside the g-C3N4 sheets.
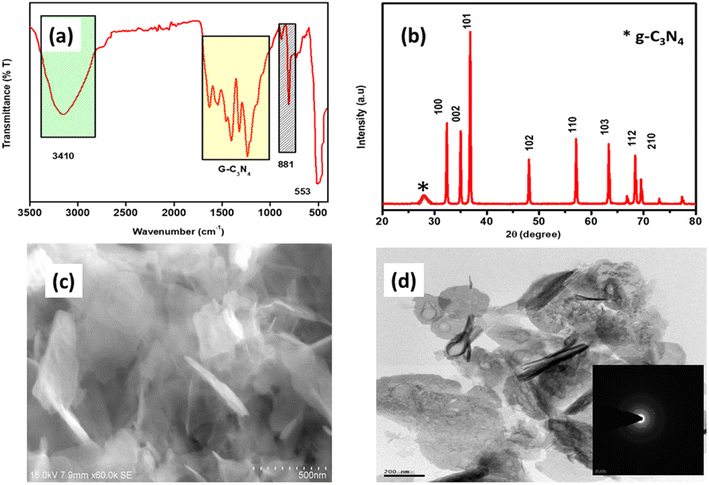 |
| Fig. 1 (a) The FTIR spectrum, (b) XRD pattern, (c) SEM, and (d) HRTEM analysis of the g-C3N4@ZnO nanocomposite. | |
Fig. 2(a) and (b) provide the particle size distribution and zeta potential studies of the g-C3N4@ZnO nanocomposite, respectively. The particle size distribution analysis indicates that g-C3N4@ZnO in the solution phase was within the nanometre size range i.e., the average particle size was around 122 nm (Fig. 2(a)). Further, the zeta potential measurements provided in Fig. 2(b) confirm the negative surface charges of the g-C3N4@ZnO composite with a value of −33.84 mV.
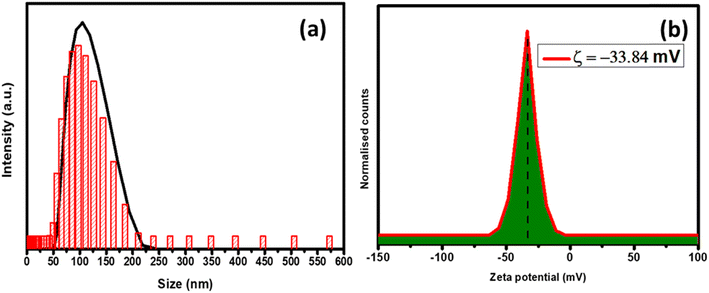 |
| Fig. 2 (a) The particle size analysis and (b) the zeta potential pattern of the g-C3N4@ZnO nanocomposite. | |
The X-ray photoelectron spectroscopy (XPS) analysis was performed to investigate the elemental composition of the g-C3N4/ZnO composite (a representative of heterojunctions), and the results are shown in Fig. 3(a) and (b). Fig. 3(a) represents the XPS analysis, wherein all the elements present in the composite, including Zn, O, C, and N, are seen as analysed by the CASA software. Moreover, the percentage elemental composition is shown in Fig. 3(b).
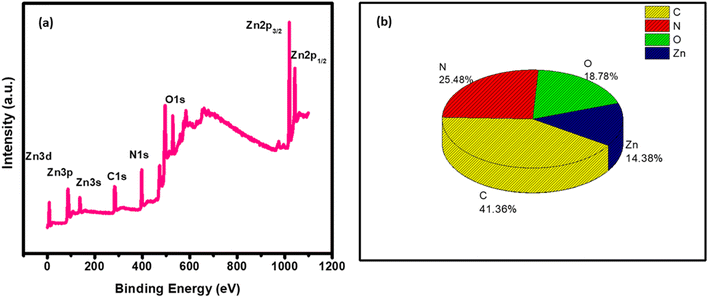 |
| Fig. 3 (a) The XPS survey spectrum and (b) percentage elemental composition of g-C3N4@ZnO. | |
Similarly, in the XPS analysis of the surface species of the g-C3N4@ZnO sample, as shown in Fig. 4(a–d), the C 1s spectrum presented two different peaks at 284.9 and 288.2 eV corresponding to the surface adventitious C and sp2-bonded C in the N
C–N coordination bond, respectively (Fig. 4(a)).35 Further, in the O 1s spectrum (Fig. 4(b)), the peaks observed at 531.2 and 529.3 eV can be attributed to chemisorbed (OC) oxygen, oxygen from the deficient regions (OV), and the O22− species in the lattice (OL).33 Based on the observation of the O peak at 529.3 eV related to the chemisorbed oxygen species, it can be inferred that this element is associated with the high-level surface defects in the g-C3N4/ZnO nanocomposite, while the other peak at 531.2 eV is from the surface hydroxyl functional groups.34 Similarly, the N and Zn spectra shown in Fig. 4(c) and (d) confirm the presence of the respective elements in the composite; the characteristic peaks of Zn appearing at 1020.0 and 1042.0 eV can be linked to Zn 2p3/2 and 2p1/2, respectively. Further, the observation of a positive shift for the O 1s binding energy position and the negative shift of the Zn 2p peaks suggest the possible chemical bond formation between the ZnO and g-C3N4 components in the composite.
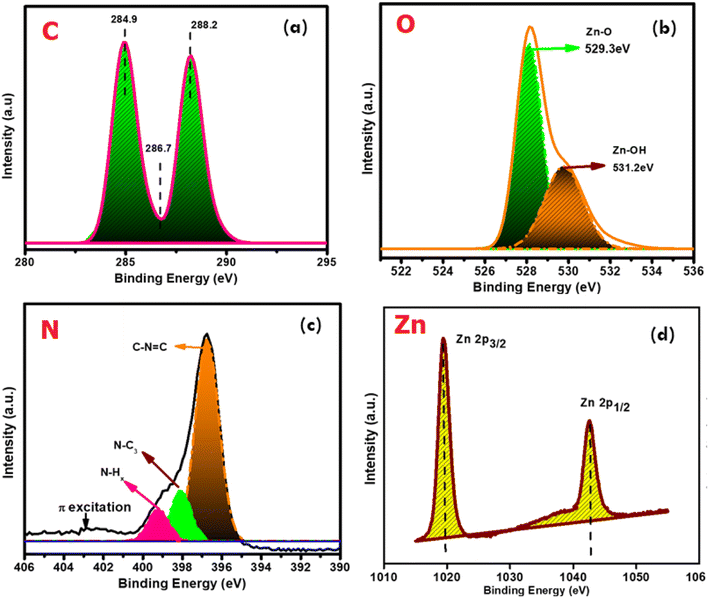 |
| Fig. 4 The deconvoluted spectra of the elements (a) C, (b) O, (c) N, and (d) Zn present in the g-C3N4@ZnO sample. | |
The comparison of the thermogravimetric analysis (TGA) and differential thermal analysis (DTA) studies of the g-C3N4/ZnO composite is provided in Fig. 5. Weight loss in the spectrum is categorized into three different regions comprising minor weight loss, significant weight loss, and the residual mass. In the graph, a minor weight loss is observed at around 180 °C, which is linked to the loss of adsorbed water molecules, moisture, or hydroxyl groups. The significant weight loss of the composite at around 450 °C can be linked to the phase change of melamine into g-C3N4. In the same way, the significant weight loss around 550 °C may be due to the degradation of the g-C3N4 layer in the g-C3N4@ZnO composite.36
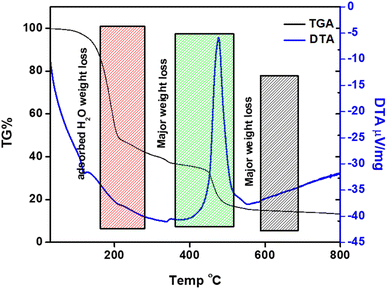 |
| Fig. 5 Comparison of the TGA and DTA profiles of the g-C3N4@ZnO composite. | |
The atomic force microscopic (AFM) studies provided the topological analysis of the g-C3N4@ZnO composite. In Fig. 6(a) and (b), the top view of the ZnO@g-C3N4 sample shows a well-organized homogenous pattern. In addition, the analysis indicated that the nanorods of ZnO were immobilized inside the layers of g-C3N4, and thus, the results are in good agreement with the HRTEM analysis (Fig. 1(d)).
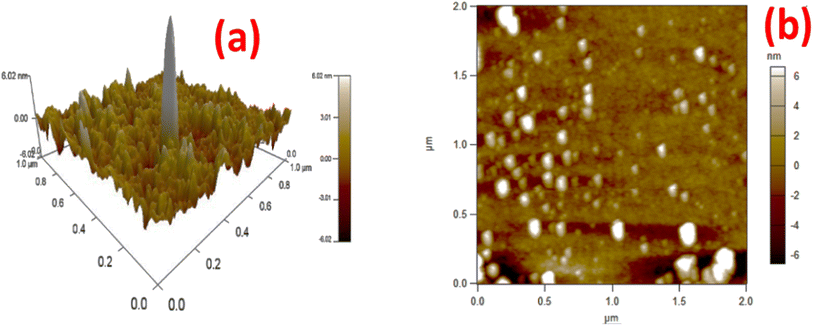 |
| Fig. 6 The AFM topography of the g-C3N4@ZnO composite (a) 3D and (b) 2D images. | |
The release of curcumin from the composite of g-C3N4@ZnO at pH 7.4 (neutral) and 5 (slightly acidic) are compared in Fig. 7(a) and (b), while the release kinetics are presented in Fig. 7(c). From the analysis, it could be observed that the composite released 90% of the loaded drug at the acidic pH of 5 in the first 60 min; however, at the neutral pH of 7.4, the drug release from the composite was around 66%. This difference in release pattern is due to the enhanced interaction of protons in the acidic medium, which allows the g-C3N4 groups to open up the cavities/pores easily to mediate the release of curcumin.
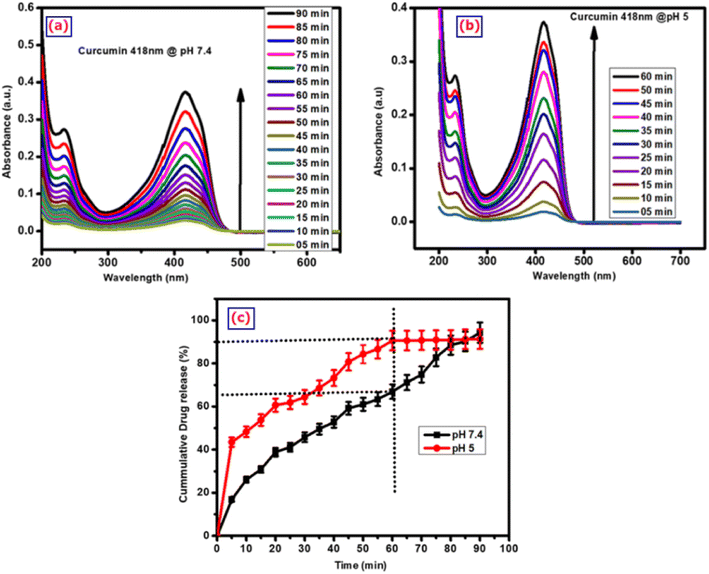 |
| Fig. 7 The UV-vis spectroscopic analysis of curcumin drug released from the g-C3N4@ZnO/C composite at pH (a) pH 7.4 and (b) 5; (c) the corresponding curcumin drug release kinetics from the composite at pH 7.4 and 5. | |
The antibacterial activity of the g-C3N4@ZnO/C composite against the bacterial cultures of E. coli and S. aureus at different drug concentrations between 25 and 100 mg mL−1 is shown in Fig. 8(i) and (ii). It can be observed from the figure that the composite exhibited significant levels of antibacterial activity against both cultures, and this activity increased with an increase in concentration. Such activity of the composite is due to the presence of Zn2+ and curcumin in the composite, i.e., the interaction of Zn2+ with the cell wall results in the depletion of the cell membrane, causing the release of the intracellular fluids, which is associated with loss of viability. Additionally, the curcumin drug also interacts with the physiological pathways of the cells, disrupts the normal mechanisms and eventually leads to bacterial cell death (Table 1).
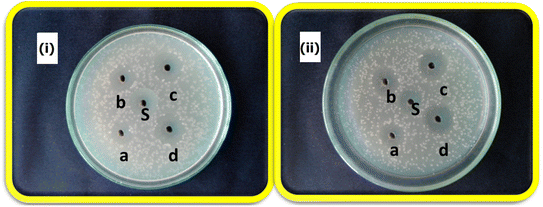 |
| Fig. 8 The antibacterial activity of g-C3N4@ZnO/C against (i) E. coli and (ii) S. aureus. a, b, c, d, and S correspond to the testing sample concentrations 25, 50, 75, and 100 mg mL−1 and control, respectively. | |
Table 1 The ZOI of g-C3N4@ZnO/C against (i) E. coli and (ii) S. aureus. a, b, c, d, and S correspond to the testing sample concentrations 25, 50, 75, and 100 mg mL−1 and control, respectively
Microorganisms |
Zone of inhibition (mm) |
a |
b |
c |
d |
S |
Staphylococcus aureus |
8 |
9 |
11 |
13 |
13 |
Escherichia coli |
8 |
10 |
12 |
14 |
12 |
The cell viability and proliferation studies of the g-C3N4@ZnO/C composite were carried out by the MTT assay, i.e. the cells were treated with different concentrations of the drug (1 to 250 μg mL−1) and for 7 consecutive days (day 1–7), and the results are shown in Fig. 9(i) and (ii), respectively. In the concentration- and time-dependent cell viability studies, the reduction in cell viability was significant at 250 μg mL−1 (day 1). As the concentration increased, the reduction in viable cells was also enhanced. Further, the microscopic images of the treated cells shown in Fig. 9(iii) indicate decreased cell viability levels over different periods and concentrations. The mechanism of action of the drug from the nanocomposite is given in the ESI section (Fig. S1)† along with the confocal images of (a) control, (b) g-C3N4@ZnO, (C) curcumin, and (d) g-C3N4@ZnO/curcumin, as well as (d) cell viability at 24 h (Fig. S2†). In the confocal microscopy images, we could see curcumin inside the MDA-MD-231 cells. The cell viability results showed that the minimum cell viability (%) was observed in g-C3N4@ZnO/curcumin nanocomposite, indicating its effective performance against MDA-MD-231 cells.
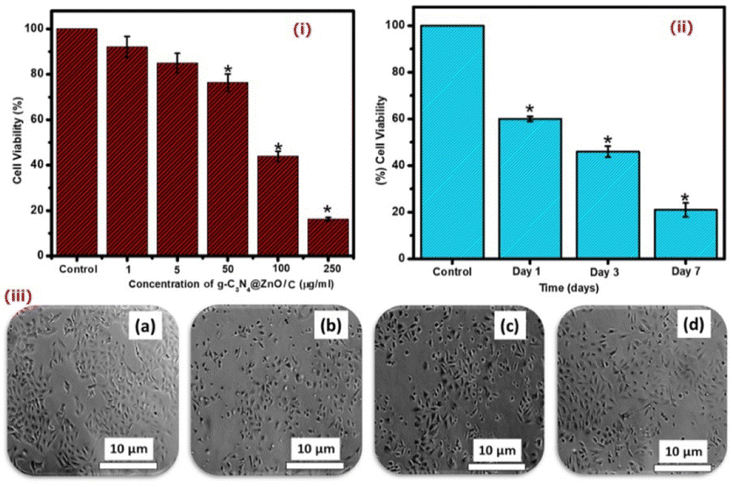 |
| Fig. 9 The in vitro cell viability and proliferation studies of MDA-MD-231 cells treated with the g-C3N4@ZnO/C sample at different (i) concentrations and (ii) treatment periods, as evaluated by the MTT assay. (iii) The cell morphology of MDA-MD-231 cells treated with g-C3N4@ZnO/C at the IC50 of 94 μg mL−1, as photographed using a simple microscope: (a) control, (b) day 1, (c) day 3, and (d) day 7. | |
The reduction in cell viability followed by treatment with the C3N4@ZnO/C sample presents preliminary evidence for the anticancer activity of the formulation and can be attributed to the presence of Zn metal and the medicinal properties of curcumin.
The cytotoxic mechanism of the g-C3N4@ZnO/C composite was determined using the LDH-colorimetric assay, as shown in Fig. 10. The assay is dependent on the cytoplasmic LDH enzyme-catalyzed oxidation of lactate to pyruvate and vice versa as LDH is a known marker for understanding membrane integrity and also a regulator of vital biochemical reactions.37,38 From the graph, it is clear that the g-C3N4@ZnO/C-treated cells released LDH, which increased with drug concentration. LDH released from the plasma damages the cell membrane, causes shrinkage of the cytoplasm, and cleaves the DNA into smaller fragments.39,40 A significant increase in the release of LDH from the cells was observed at concentrations above 50 μg mL−1, and at the highest concentration 250 μg mL−1, LDH release was around 78% compared with the control sample. Therefore, g-C3N4@ZnO/C shows significant levels of toxicity to the cancer cells, and thus, the composite can be a potential platform for the treatment of tumour cells.
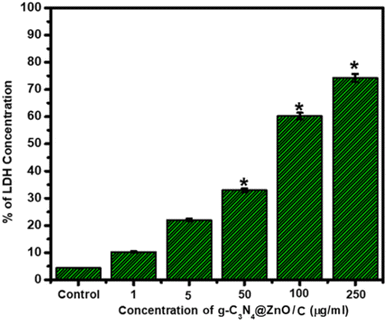 |
| Fig. 10 Variations in LDH (%) release from MDA-MD-231 cells treated with the g-C3N4@ZnO/C sample at different concentrations up to 250 μg mL−1. | |
4. Conclusion
In summary, we indicate the successful fabrication of the g-C3N4@ZnO composite using an in situ fabrication process followed by the efficient loading of curcumin drug into the composite. On testing the composite, the FT-IR analysis showed the conjugation of the g-C3N4 groups with ZnO, while the crystallinity of the composite was determined by XRD. The HRTEM analysis confirmed the rod-shaped morphology of the particles, which were partially wrapped in the sheets of g-C3N4. Moreover, the elements present in the composite were analyzed using XPS, which confirmed the presence of C, N, Zn, and O and thereby verified the successful formation of the g-C3N4/ZnO composite. The drug release profiles of curcumin at two different pHs indicated that the acidic pH 5 is more suitable for sustainable drug release. The biological evaluation of the g-C3N4@ZnO/C sample supported its significant antibacterial activity against E. coli and S. aureus in addition to the anticancer action against the MDA-MB-231 cancer cells, demonstrating a very low IC50 value of 94 μg mL−1 at 24 h. This composite is the first to be prepared by an in situ method and used for treatment against MDA-MB-231 cells. From the SEM, TEM and AFM analyses, we confirmed that the ZnO nanoparticles were embedded in the 2D stacked layers of g-C3N4. Usually, a two-step process is employed for preparing this composite. We have adopted a single-step process, and ZnO growth on the layers of g-C3N4 is clearly visible from the morphological analysis. In this work, we have achieved the embedding of nanoparticles with a rod-like morphology on a layered structure. The rod-like morphology serves as a good tool for sustained drug delivery. Further observation of LDH activity indicated that the mechanistic action of g-C3N4@ZnO/C is mediated by the presence of Zn and curcumin drug, thereby providing preliminary evidence that this drug-loaded nanocomposite can be an ideal candidate for treating breast cancer cells.
Conflicts of interest
There is no conflict of interest with this work.
Acknowledgements
One of the authors Varun Prasath Padmanabhan would like to acknowledge the financial support from Council of Scientific and Industrial Research (CSIR), Government of India, for the Research Associate Fellowships (09/115(0793)/2020 EMR-I).
References
- E. C. Dreaden, A. M. Alkilany, X. Huang, C. J. Murphy and M. A. El-Sayed, The golden age: gold nanoparticles for biomedicine, Chem. Soc. Rev., 2012, 41(7), 2740–2779 RSC.
- C. M. Cobley, J. Chen, E. C. Cho, L. V. Wang and Y. Xia., Gold nanostructures: a class of multifunctional materials for biomedical applications, Chem. Soc. Rev., 2011, 40(1), 44–56 RSC.
- X.-d. Wang, T.-y. Zhou, X.-h. Song, Y. Jiang, C. J. Yang and X. Chen, Chameleon clothes for quantitative oxygen imaging, J. Mater. Chem., 2011, 21(44), 17651–17653 RSC.
- K. Yang, L. Feng, X. Shi and Z. Liu, Nano-graphene in biomedicine: theranostic applications, Chem. Soc. Rev., 2013, 42(2), 530–547 RSC.
- X. Cai, H. Dong, J. Ma, H. Zhu, W. Wu, M. Chu, Y. Li and D. Shi, Effects of spatial distribution of the nuclear localization sequence on gene transfection in catiomer–gene polyplexes, J. Mater. Chem. B, 2013, 1(12), 1712–1721 RSC.
- K. P. Loh, Q. Bao, G. Eda and M. Chhowalla, Graphene oxide as a chemically tunable platform for optical applications, Nat. Chem., 2010, 2(12), 1015 CrossRef CAS PubMed.
- X. Chen, B. Su, G. Wu, C. J. Yang, Z. Zhuang, X. Wang and X. Chen, Platinum nanoflowers supported on graphene oxide nanosheets: their green synthesis, growth mechanism, and advanced electrocatalytic properties for methanol oxidation, J. Mater. Chem., 2012, 22(22), 11284–11289 RSC.
- L. Feng, L. Wu and X. Qu, New horizons for diagnostics and therapeutic applications of graphene and graphene oxide, Adv. Mater., 2013, 25(2), 168–186 CrossRef CAS PubMed.
- C.-H. Lu, H. -H. Yang, C. -L. Zhu, X. Chen and G. -N. Chen, A graphene platform for sensing biomolecules, Angew. Chem., 2009, 121(26), 4879–4881 CrossRef.
- S. He, B. Song, D. Li, C. Zhu, W. Qi, Y. Wen, L. Wang, S. Song, H. Fang and C. Fan, A graphene nanoprobe for rapid, sensitive, and multicolor fluorescent DNA analysis, Adv. Funct. Mater., 2010, 20(3), 453–459 CrossRef CAS.
- Q. Liu, B. Guo, Z. Rao, B. Zhang and J. R. Gong, Strong two-photon-induced fluorescence from photostable, biocompatible nitrogen-doped graphene quantum dots for cellular and deep-tissue imaging, Nano Lett., 2013, 13(6), 2436–2441 CrossRef CAS PubMed.
- Z. Liu, J. T. Robinson, X. Sun and H. Dai, PEGylated nanographene oxide for delivery of water-insoluble cancer drugs, J. Am. Chem. Soc., 2008, 130(33), 10876–10877 CrossRef CAS PubMed.
- B. Tian, C. Wang, S. Zhang, L. Feng and Z. Liu, Photothermally enhanced photodynamic therapy delivered by nano-graphene oxide, ACS Nano, 2011, 5(9), 7000–7009 CrossRef CAS PubMed.
- P. Huang, J. Lin, X. Wang, Z. Wang, C. Zhang, M. He and K. Wang, et al., Light-triggered theranostics based on photosensitizer-conjugated carbon dots for simultaneous enhanced-fluorescence imaging and photodynamic therapy, Adv. Mater., 2012, 24(37), 5104–5110 CrossRef CAS PubMed.
- J. T. Robinson, S. M. Tabakman, Y. Liang, H. Wang, H. S. Casalongue, D. Vinh and H. Dai, Ultrasmall reduced graphene oxide with high near-infrared absorbance for photothermal therapy, J. Am. Chem. Soc., 2011, 133(17), 6825–6831 CrossRef CAS PubMed.
- M. Li, L. Zhang, M. Wu, Y. Du, X. Fan, M. Wang, L. Zhang, Q. Kong and J. Shi, Mesostructured CeO2/g-C3N4 nanocomposites: remarkably enhanced photocatalytic activity for CO2 reduction by mutual component activations, Nano Energy, 2016, 19, 145–155 CrossRef CAS.
- J. Tian, L. Zhang, M. Wang, X. Jin, Y. Zhou, J. Liu and J. Shi, Remarkably enhanced H2 evolution activity of oxidized graphitic carbon nitride by an extremely facile K2CO3-activation approach, Appl. Catal., B, 2018, 232, 322–329 CrossRef CAS.
- S. Cao, J. Low, J. Yu and M. Jaroniec, Polymeric photocatalysts based on graphitic carbon nitride, Adv. Mater., 2015, 27(13), 2150–2176 CrossRef CAS PubMed.
- F. Su, S. C. Mathew, G. Lipner, X. Fu, M. Antonietti, S. Blechert and X. Wang, mpg-C3N4-catalyzed selective oxidation of alcohols using O2 and visible light, J. Am. Chem. Soc., 2010, 132(46), 16299–16301 CrossRef CAS PubMed.
- Y. Zhang, M. Li, X. Gao, Y. Chen and T. Liu, Nanotechnology in cancer diagnosis: progress, challenges and opportunities, J. Hematol. Oncol., 2019, 12, 1–13 CrossRef PubMed.
- C. Maksoudian, N. Saffarzadeh, E. Hesemans, N. Dekoning, K. Buttiens and S. J. Soenen, Role of inorganic nanoparticle degradation in cancer therapy, Nanoscale Adv., 2020, 2, 3734–3763 RSC.
- H. Leelavathi, R. Arulmozhi, R. Muralidharan, N. Abirami, S. Tamizharasan and A. Kumarasamy, Exploration of ZnO decorated g-C3N4 amphiphilic anticancer drugs for antiproliferative activity against human cervical cancer, J. Drug Deliv. Sci. Technol., 2022, 22, 103126 CrossRef.
- W. P. T. D. Perera, R. K. Dissanayake, U. I. Ranatunga, N. M. Hettiarachchi, K. D. C. Perera, J. M. Unagolla, R. T. De Silva and L. R. Pahalagedar, Curcumin loaded zinc oxide nanoparticles for activity-enhanced antibacterial and anticancer applications, RSC Adv., 2020, 10, 30785–30795 RSC.
- Z. Sayyar, H. Jafarizadeh-Malmiri and N. Beheshtizadeh, A study on the anticancer and antimicrobial activity of curcumin nanodispersion and synthesized ZnO nanoparticles, Process Biochem., 2022, 121, 18–25 CrossRef CAS.
- Y. Xia, R. Xu, S. Ye, J. Yan, P. Kumar, P. Zhang and X. Zhao, Microfluidic Formulation of Curcumin-Loaded Multiresponsive Gelatin Nanoparticles for Anticancer Therapy, ACS Biomater. Sci. Eng., 2023, 9, 3402–3413 CrossRef CAS PubMed.
- X. Yuan, S. Duan, G. Wu, L. Sun, G. Cao, D. Li, H. Xu, Q. Li and D. Xia, Enhanced catalytic ozonation performance of highly stabilized mesoporous ZnO doped g-C3N4 composite for efficient water decontamination, Appl. Catal., A, 2018, 551, 129–138 CrossRef CAS.
- Y. Wang, R. Shi, J. Lin and Y. Zhu, Enhancement of photocurrent and photocatalytic activity of ZnO hybridized with graphite-like C3N4, Energy Environ. Sci., 2011, 4(8), 2922–2929 RSC.
- W. Liu, M. Wang, C. Xu, S. Chen and X. Fu, Significantly enhanced visible-light photocatalytic activity of g-C3N4 via ZnO modification and the mechanism study, J. Mol. Catal. A: Chem., 2013, 368, 9–15 CrossRef.
- Y.-P. Zhu, M. Li, Y.-L. Liu, T.-Z. Ren and Z.-Y. Yuan, Carbon-doped ZnO hybridized homogeneously with graphitic carbon nitride nanocomposites for photocatalysis, J. Phys. Chem. C, 2014, 118(20), 10963–10971 CrossRef CAS.
- J. Wang, Z. Yang, X. Gao, W. Yao, W. Wei, X. Chen, R. Zong and Y. Zhu, Core-shell g-C3N4@ ZnO composites as photoanodes with double synergistic effects for enhanced visible-light photoelectrocatalytic activities, Appl. Catal., B, 2017, 217, 169–180 CrossRef CAS.
- T. J. Park, R. C. Pawar, S. Kang and C. S. Lee, Ultra-thin coating of gC 3 N 4 on an aligned ZnO nanorod film for rapid charge separation and improved photodegradation performance, RSC Adv., 2016, 6(92), 89944–89952 RSC.
- Y. Wang, X. Liu, J. Liu, B. Han, X. Hu, F. Yang and Z. Xu, et al., Carbon quantum dot implanted graphite carbon nitride nanotubes: excellent charge separation and enhanced photocatalytic hydrogen evolution, Angew. Chem., 2018, 130(20), 5867–5873 CrossRef.
- W.-K. Jo and N. C. S. Selvam, Enhanced visible light-driven photocatalytic performance of ZnO–g-C3N4 coupled with graphene oxide as a novel ternary nanocomposite, J. Hazard. Mater., 2015, 299, 462–470 CrossRef CAS PubMed.
- X. Wang, K. Maeda, A. Thomas, K. Takanabe, G. Xin, J. M. Carlsson, K. Domen and M. Antonietti, A metal-free polymeric photocatalyst for hydrogen production from water under visible light, Nat. Mater., 2009, 8(1), 76–80 CrossRef CAS PubMed.
- X. Zhang, J. Qin, Y. Xue, P. Yu, B. Zhang, L. Wang and R. Liu, Effect of aspect ratio and surface defects on the photocatalytic activity of ZnO nanorods, Sci. Rep., 2014, 4, 4596 CrossRef PubMed.
- C. Chang, Y. Fu, M. Hu, C. Wang, G. Shan and L. Zhu, Photodegradation of bisphenol A by highly stable palladium-doped mesoporous graphite carbon nitride (Pd/mpg-C3N4) under simulated solar light irradiation, Appl. Catal., B, 2013, 142, 553–560 CrossRef.
- J. H. Zheng, Q. Jiang and J. S. Lian, Synthesis and optical properties of flower-like ZnO nanorods by thermal evaporation method, Appl. Surf. Sci., 2011, 257(11), 5083–5087 CrossRef CAS.
- A. Mathialagan, M. Manavalan, K. Venkatachalam, F. Mohammad, W. C. Oh and S. Sagadevan, Fabrication and physicochemical characterization of g-C3N4/ZnO composite with enhanced photocatalytic activity under visible light, Opt. Mater., 2020, 100, 109643 CrossRef CAS.
- R. K. Murry, P. K. Gerner, P. A. Mays and V. W. Rodwell, Harper's Textbook of Biochemistry, Appleton & Lange, Stanford, 2000 Search PubMed.
- A. L. Holder, R. Goth-Goldstein, D. Lucas and C. P. Koshland, Particle-induced artifacts in the MTT and LDH viability assays, Chem. Res. Toxicol., 2012, 25(9), 1885–1892 Search PubMed.
|
This journal is © The Royal Society of Chemistry 2023 |
Click here to see how this site uses Cookies. View our privacy policy here.