DOI:
10.1039/D3RA03689H
(Paper)
RSC Adv., 2023,
13, 23708-23715
Spectral properties and self-reduction of Eu3+ to Eu2+ in aluminosilicate oxyfluoride glass
Received
2nd June 2023
, Accepted 31st July 2023
First published on 7th August 2023
Abstract
Eu-doped aluminosilicate oxyfluoride glass prepared via a melt-quenching method was investigated using X-ray diffraction, absorption spectroscopy, X-ray fluorescence spectrometry, photoluminescence spectroscopy and fluorescence decay curves. We found that the reduction of Eu3+ to Eu2+ ions occurred in the glass prepared in air. The emission spectra showed that the intensity of 4f65d → 4f7 transition of Eu2+ ions varied with increasing incident beam wavelength. Meanwhile, the fluorescence lifetimes of Eu3+: 5D0 → 7F2 monitored at 617 nm in the glass change with the variation of excitation wavelength. The energy transfer between Eu2+ and Eu3+ and the emission mechanisms of Eu2+ ions in the glass were also discussed.
1 Introduction
The photoluminescence (PL) of Eu-doped materials has been of extensive interest not only because of the excellent red emission of Eu3+ (ascribed to 4f–4f electronic forbidden transitions and the exhibiting of a series of highly bright and sharply narrow emissions),1–3 but because of the characteristic broadband emission of Eu2+ (attributed to inter-configurational 4f65d → 4f7(8S7/2) transitions and tuned from near-ultraviolet (UV) to red in different hosts).4–7 Impressively, due to stronger coupling to lattice vibrations (i.e., the strong interaction of 5d electrons with the local crystal field in vicinity of Eu2+), the unique electronic configuration of Eu2+ (4f7-4f65d) makes its luminescent properties sensitive to the coordination environment.8,9 This is why the emission of Eu2+ could present colorful emissions, which has recently made it a perfect candidate for color-tunable phosphors, such as the blue phosphor Mg2Al4Si5O18:Eu2+,10 the green phosphor Rb3M(Li3SiO4)4:Eu2+ (M = Rb or Na),11 the green-to-yellowish-orange phosphor Cs2MP2O7:Eu2+ (M = Ba, Sr, and Ca),12 the red phosphor CaS: Eu,13 and so on. Thus, Eu2+-doped phosphors are of great technological interest for applications in white LED, optical thermometers, backlight displays, and X-ray storage, etc.10,11,14
Aluminosilicate oxyfluoride glasses and glass-ceramics containing two kinds of anions (O2− and F−) with different valence electrons and different degrees of polarization is another kind of promising host for RE ions because they combine the advantages of the high mechanical strength of oxide glass and the low phonon energy of fluoride glass.14–16 Still, instead of the high-performance narrow-band emissions in Eu2+-activated phosphors for some special applications (e.g., fluorescent illumination and X-ray intensifying panels),17,18 divalent europium ions generally emit only a broad band blue emission in vitreous hosts.19,20 In other words, the amorphous structure of glass, which resulting in a higher phonon energy, enhances the overlap of electronic orbit among energy levels in 4f65d configuration, i.e., the broad blue emission originates in the minor splitting energy of Eu 5d-band. However, the broad band emission of Eu2+ ions precisely makes it possible to generate tunable smooth spectra, especially warm white light-emitting, in single rare earth doped glass materials by regulating the emission intensity ratio between Eu3+ and Eu2+.21,22 In addition, differing from the reduction process in phosphors synthesis (i.e., in a strongly reducing atmosphere, e.g., H2/N2, etc.), Eu3+ → Eu2+ conversion in glass melting is fulfilled in air by adding a reducing agent into the glass composition (e.g., Al) or adjusting the optical basicity (Λth) of the glass matrix (i.e., to make the Λth value of base glass less than 0.585 by optimizing glass composition.).3,12,23–25 Obviously, the reduction routes implemented in Eu-doped glasses are relatively convenient. All these mean that Eu-doped oxyfluoride glass materials have promising prospects in the quality lighting field.
In the present study, we developed an Eu-doped glass composition within the system SiO2–Al2O3–BaF2–Na2O containing both Eu3+ and Eu2+ ions, which was synthesized by partially reducing Eu3+ ions without using a reducing atmosphere and/or any reductant during the melting process. We performed multispectral excitation measurements (at the incident wavelength (λex) ranging from 320 nm to 360 nm with an interval of 5 nm) and structure analysis to study the emission properties of Eu2+ ions in the glass sample. The energy transfer from Eu2+ to Eu3+ and the emission mechanisms of Eu2+ ions in the glass sample were discussed.
2 Experimental
Aluminosilicate oxyfluoride glass with the nominal molar composition of 40SiO2–25Al2O3–20BaF2–15Na2O:1Eu was synthesized by a melt-quenching method, using SiO2 (99.99%, Aladdin), Al2O3 (99.99%, Aladdin), BaF2 (99.99%, Aladdin), Na2CO3 (99.99%, Aladdin), and Eu2O3 (99.99%, Aladdin) as raw materials. All the chemicals were used as received without further purification. Accurately weighed 10 g per batch of raw materials were fully mixed and melted in a covered alumina crucible at 1723 K for 1 h. The melt was cast quickly into a metal mold with cover on copper-alloy plate of 573 K and finally cooled down to room temperature in air to obtain glass sample. It should be noted that the melting crucible for melting the glass batch was covered by another bigger alumina crucible to protect local atmosphere, i.e., to reduce the volatilization loss of fluoride.26,27 The obtained glass sample was further polished to 1.5 mm in thickness for further optical measurements after annealed 10 h at 520 °C.
The amorphous characteristic of sample was determined with an X-ray diffraction (XRD, Shimadzu LabX XRD-6100) with Cu/Kα (λ = 1.540598 Å) by short scanning in the 2θ range of 10–70° (2θ) at a scan rate of 6 ° min−1. The UV/VIS/NIR absorption spectrum was recorded in the wavelength range from 200 to 450 nm using a Model U-4100 Spectrophotometer. The final product composition was measured using the X-ray Fluorescence Spectrometer (ZSX Primus II, Rigaku, JPN). The photoluminescence and decay curves were performed in FLS980 fluorescence spectrometer (FLS980, Edinburgh Instruments, UK) equipped with a Xe-lamp and μs flash lamp as excitation sources. All spectroscopic measurements were performed at room temperature.
3 Results and discussion
The X-ray diffraction pattern of the Eu-doped aluminosilicate oxyfluoride glass is shown in Fig. 1A. The characteristic amorphous halo (i.e., a broad hump located at 27° (2θ)) and no diffraction peaks (i.e., no crystalline phases precipitated from the sample) are observed in the spectrum. Fig. 1B shows the absorption spectrum of the Eu-doped as-prepared glass. It can be seen from this figure, a broad absorption band peaking at 275 nm and two absorption shoulders on the low-energy side, decomposed into three fitting bands using Gaussian function, are observed. The most remarkable band, peaking at 275 nm, is assigned to aluminosilicate oxyfluoride glass host absorption. The other two weaker absorption peaks could be attributed to the electronic transition of 4f7 → 4f65d, i.e., the 5d orbital is split into two components t2g (318 nm) and eg (389 nm) by the crystal-field strength of the coordinates in the vicinity of Eu2+ ions.28 In addition, the Eu3+ also shows an absorption peak in the range 388–396 nm, whereas the absorption due to the Eu3+: f–f transitions in aluminosilicate glass is obscure owing to their small absorption coefficients.28 It is noteworthy that, differing from the absorption spectra in Eu-doped phosphors (i.e., where the absorption band of host and those of Eu2+ ions were obviously separated),12,29 the absorption bands of host and Eu2+ ions are closely overlapping in the aluminosilicate oxyfluoride glass. In addition, this absorption band is not in agreement with Eu2+ absorption spectra in oxide aluminosilicate glasses, where a broad absorption band considered to contain only the eg and t2g splitting components of 5d orbitals of Eu2+ ions were observed, and believed that the sites of cubic symmetry with 8- and 12-fold coordination resulted in a splitting to eg component at lower energy and t2g component at higher energy.28,30 This result indicates that the phonon energy caused by lattice vibration of the aluminosilicate oxyfluoride host glass has extended into the bandgap of Eu2+ ions,31,32 which means the 5d energy level of the doped Eu2+ ions should be widen. Furthermore, IR spectra also manifests the multiple broad-band absorption peaks (Fig. 1C. 950 cm−1: Si–O–Si, 690 cm−1: Al–O, and 440 cm−1: Si–O–Si and O–Si–O, etc.), meaning various energies (often referred to collectively as ‘phonon energy’) contained in glass. The broad bands will benefit the overlap of electronic orbit among energy levels in 4f65d configuration of Eu2+ ions. This predicts that, compared to the emission spectra of Eu2+ in above-mentioned hosts, especially the phosphor hosts, a broader emission band of Eu2+ will be observed in the studied host glass, i.e., the 40SiO2–25Al2O3–20BaF2–15Na2O glass.
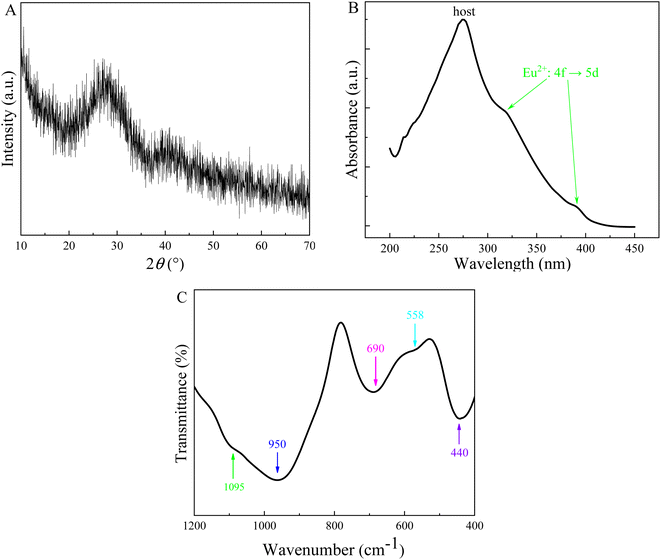 |
| Fig. 1 XRD patterns spectrum (A), optical absorption spectrum (B) and IR spectra (C) for the Eu-doped 40SiO2–25Al2O3–20BaF2–15Na2O glass. The broad absorption band in (B) contains two shoulders, indicating it could be deconvoluted into three Gaussian functions. IR spectra in (C) manifests multiple broad-band absorption peaks. | |
Fig. 2 exhibits the excitation spectra of Eu2+ and Eu3+ ions for Eu-doped aluminosilicate oxyfluoride glass. We can see from this figure that the 5d band of Eu2+ is observed in the excitation spectrum monitored at 471 nm. Furthermore, the broad excitation band (i.e., the energy transfer Eu2+: 8S7/2 → 5d) can be fitted into two bands by Gaussian function, indicating that the 5d orbital of Eu2+ ions in the studied glass system is split to two components t2g and eg by its surrounding crystal field. At the same time, one can observe in this figure, two weaker excitation bands around 320 nm (just showing half peak because of a severe disturbance by the frequency-doubled excitation peak of FLS1000 fluorescence spectrometer used in this study) and 370 nm can also be distinguished in the excitation spectrum of Eu3+ ions. This indicates the possibility that energy transfer (ET) from Eu2+ ions 5d level to the Eu3+ ions 4f levels. Indeed, as Malchukova proved,20 the overlap between the broad emission of Eu2+ ions and the various excitation bands of Eu3+ ions is observed in Fig. 3.
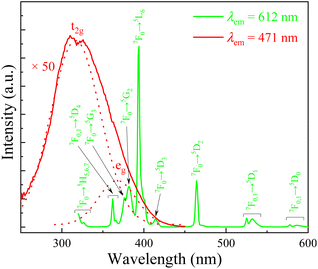 |
| Fig. 2 Excitation spectra for Eu2+ ions (red line) and Eu3+ ions (green line) monitored at 471 and 612 nm, respectively, measured for the Eu-doped 40SiO2–25Al2O3–20BaF2–15Na2O glass. The broad excitation band was deconvoluted to two Gaussian functions (dotted red lines). The excitation spectrum and its two Gaussian fitting bands were magnified 50 times in intensity (for interpretation of the references to color in this figure legend, the reader referred to the web version of this article). | |
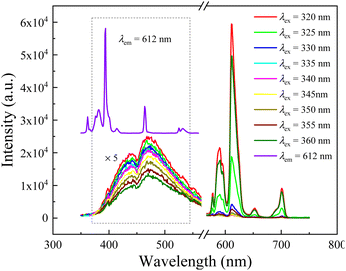 |
| Fig. 3 Emission spectra for Eu2+ ions in dependence on excitation wavelength from 320 nm to 360 nm with an interval of 5 nm and excitation spectrum for Eu3+ ions (purple line) monitored at 612 nm, respectively, measured for Eu-doped 40SiO2–25Al2O3–20BaF2–15Na2O as-prepared glass. The emission spectra bands at the wavelength range from 350 to 560 nm are magnified 5 times in intensity and show “holes” corresponding to Eu3+ ions absorption. Excitation spectrum of Eu3+ ions is vertically shifted to make comparison (for interpretation of the references to color in this figure legend, the reader referred to the web version of this article). | |
The down conversion luminescence observed in the Eu-doped 40SiO2–25Al2O3–20BaF2–15Na2O as-prepared glass can be attributed to both Eu3+ and Eu2+ ions, as shown in Fig. 3. This result confirms therefore that trivalent europium ions have been partially reduced to divalent europium in this studied aluminosilicate oxyfluoride glass composition synthesized in air at high temperature. In other words, the broad blue-green emission with maximum at 470 nm undoubtedly belongs to the transition 4f65d → 4f7 of Eu2+ ions. However, in this work, no reducing atmosphere or agent is employed to promote the reduction of Eu3+ ions (Eu2O3 as the precursor material). Thus, the existence of Eu2+ ions in the present aluminosilicate oxyfluoride glass system obtained in normal atmospheric condition can be explained adopting optical basicity model proposed by Duffy and Ingram characterizing the glasses based on acid–base property.33 Unlike the classical principle to elucidate the synthesis mechanism for europium monoxide (EuO) via calculating various thermodynamics parameters (e.g., Gibbs free energy, configurational entropy, etc.) and analyzing the effect of high temperature on the reduction of Eu3+ → Eu2+,34,35 the optical basicity theory is fundamentally correlated to the chemical bonding in a solid and to the optical properties of a material through the polarizability of electron clouds around atoms (ions) by electromagnetic waves. Optical basicity of a glass is assumed to represent the average oxygen environment throughout the material. The optical property of glasses, e.g., the reduction–oxidation of cations, can be used to predict through optical basicity concepts.36,37
From the optical basicity concept, as the optical basicity of glass matrix is below a certain critical value (i.e., critical value of 0.585), it favors the transformation of higher oxidation state to lower oxidation state for multivalent cation europium.25 Considering equivalent mole fractions of constituent oxide or fluoride that contributes to the overall glass stoichiometry and their individual reported values of basicity along with number of oxygen or fluorine atoms, the optical basicity (Λth) of the glass matrix is calculated using Duffy's empirical formula:
|
 | (1) |
where,
qi is the number of oxygen or fluorine atoms in the
ith component oxide or fluoride,
xi is the
ith component oxide or fluoride mole fraction, and
Λi is the optical basicity of
ith oxide or fluorides. Herein, fluorine in the glass composition can be volatile during the preparation of glass at high temperature, leading the final product composition being different from the nominal one as designed.
38 Thus, we used the X-ray fluorescence spectrometer to measure the glass matrix composition of the obtained sample. The obtained base glass composition is 37.66SiO
2–27.44Al
2O
3–17.34BaF
2–3.86BaO–13.70Na
2O (in mol%).
Based on the previous reports,39,40 the optical basicity values of oxide or fluoride are listed as the following: Λ(SiO2) = 0.48, Λ(Al2O3) = 0.6, Λ(BaF2) = 0.50, Λ(BaO) = 1.15, Λ(Na2O) = 1.15. Using the Duffy's empirical formula, the optical basicity of the base glass is calculated to be 0.5864, which is very close to the critical optical basicity (Λth = 0.585). According to the theory proposed by Duffy, a higher optical basicity favors a higher valence state of the multivalence metal ions.41 This implies that it is more propitious to generation a lower valence ion, i.e., Eu2+, at lower optical basicity in this work. In addition, Z. Y. Lin et al. reported the self-reduction of Eu3+ to Eu2+ in a kind of aluminosilicate glass with the optical basicity of 0.59.14 They proposed that the structures of aluminosilicate glass play an important role in the reduction of Eu3+ ions, which changes the critical optical basicity value under the special chemical environment. In the present study, the base glass composition also contains SiO2 and Al2O3. Thus, the formation of Eu2+ is realized in the present glass.
Seen from Fig. 3, the emission intensity of Eu2+ decreases slightly in turn with the increasing excitation wavelength from 320 to 360 nm (i.e., the wavelength range between the fitting peaks of t2g and eg for the 5d energy level of Eu2+ ions). It can be attributed to the reduced energy transfer capacity of 8S7/2 level, directly leading to a reducing luminescence efficiency, as the incident light deviates from the peak of excitation band for Eu2+ ions monitored at 471 nm. However, the emission intensity of Eu3+ ions in the red wavelength range shows a variation trend, i.e., it decreases before increases with the increasing incident light wavelength in the studied excitation wavelength range (320–360 nm). Despite the possibility of energy transfer from Eu2+ to Eu3+ exists, as mentioned above, the emission of Eu2+ ions is so weak that the transferred energy is faint. Thus, the intenser luminescence of Eu3+ excited under 320, 325 and 360 nm, respectively, can be ascribe to the notable overlap between incident to the excitation transition of Eu3+: 7F0 → 5H5,6,7 and 7F0,1 → 5D4. Whereas, the rest weaker luminescence of Eu3+ may be mainly related to the ET from Eu2+ to Eu3+ ions, especially the emission spectra under 335–355 nm excitation, because that no excitation band is shown in this wavelength range for Eu3+ ions shown as in Fig. 2. Moreover, this result falls in line with the energy transfer hypothesis in the section of excitation analysis, i.e., the ET from Eu2+ ions 5d level to the Eu3+ ions 4f levels.
Fluorescence decay analysis is very useful for understanding the mechanism of rare earth luminescence, e.g., energy transfer and quenching behavior etc. Fig. 4 shows the decay curves of Eu3+ (monitored at λem = 617 nm) in the aluminosilicate oxyfluoride as-prepared glass under multispectral excitation at 320, 325, 330, 335, 340, 345, 350, 355, 360 nm, respectively. All the decay curves are fitted by a double exponential decay function with time constant parameters (ExpDec2) in Origin software using the below given expression:42
|
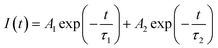 | (2) |
where
I(
t) is the emission intensity at time
t.
A1 and
A2 are the decay constants.
τ1 and
τ2 are the lifetimes of the two channels contributing to the decay processes. The average fluorescence lifetime (
τ) is estimated as follows:
|
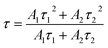 | (3) |
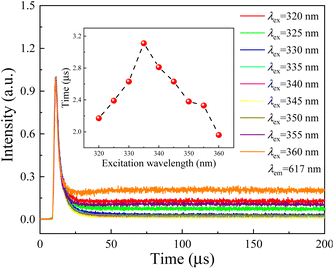 |
| Fig. 4 Room-temperature luminescence decay curves of the emission Eu3+: 5D0 → 7F2 at 617 nm excited by multispectral from 320 to 360 nm with an interval of 5 nm. All curves are normalized to make comparison. Inset: the dependence of decay lifetimes (τ) on excitation wavelength (λex). The red spheres in the inset refer to the fluorescence lifetimes of Eu3+: 5D0 in the glass sample. The dashed curves are guide for eyes to show the nonmonotonic change of τ with increasing λex. | |
Interestingly, τ evolves non-monotonically with λex as seen in the inset of Fig. 4. With increasing the excitation light wavelength, the fluorescence time first increases from 2.2 to 3.1 μs, and then decreases to 2.0 μs. This result confirms therefore that the emission mechanism of Eu3+ ions in the studied aluminosilicate oxyfluoride as-prepared glass changes with incident beam, i.e., the faster decay (e.g., excited at 320 and 325 nm) may relate to excited state absorption, and the slower decay (e.g., excited at 355 and 360 nm) can be put down to energy transfer. This implies that the excitation process is faster than the energy transfer for Eu3+ ions in the studied glass system. The mechanism of energy transfer from Eu2+: 5d to the Eu3+: 4f is similar to that described in20,28, i.e., the excited in 4f65d (eg) state of Eu2+ ions transfers the energy by means of non-radiative transition 4f65d (eg) → 8S7/2 to the ground state resulting in the excitation of 5D0 level of Eu3+ ions. We also observed another evidence for the energy transfer from Eu2+ to Eu3+ ions by analyzing the excitation spectrum (Fig. 2) and emission spectra (Fig. 3) of Eu2+, i.e., the discontinuous peaks, especially at 320 nm in the excitation band monitored at 471 nm. This type of effect where band emission is suppressed only at the position of RE absorption lines is known to be characteristic of resonant radiative energy transfer, i.e., the reabsorption mechanism.43,44 Significantly, being inconsistent with the results of Jiménez et al.,44 the “holes” on the emission bands of Eu2+ don't directly correspond the Eu3+ ions absorption peaks on its excitation band, as shown in Fig. 3. The possible reason may be the strong phonon energy of the aluminosilicate oxyfluoride host glass as observed in the above-mentioned absorption and IR analyses, i.e., implying the occurrence of multi-phonon relaxation.
The effect of varying incident beam wavelength (λex from 320 to 360 nm) on the luminescence color in Eu-doped aluminosilicate oxyfluoride as-prepared glass is displayed in Fig. 5. The luminescence color can be finely tuned by changing the excitation wavelength, corresponding CIE coordinates vary from yellowish pink (0.5422, 0.3369), warm white light (0.3845, 0.2895), to greenish blue (0.2016, 0.247).
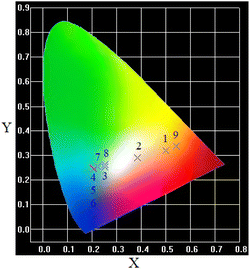 |
| Fig. 5 Dependence of CIE chromaticity diagram on excitation wavelength for the Eu-doped 40SiO2–25Al2O3–20BaF2–15Na2O glass. The numbers 1 to 9 represent the chromaticity coordinates under 320 to 360 nm excitation (for interpretation of the references to color in this figure legend, the reader referred to the web version of this article). | |
Surprisingly, we observed the heterogeneous distribution of Eu2+ ions in the studied Eu-doped glass system by analyzing the emission spectra of the other side of sample shown as in Fig. 6, i.e., a photoluminescence increases before decreases with the increasing incident light wavelength from 330–360 nm which is contrary to that shown in Fig. 3. The phenomenon may be related to the variation of the local environment of Eu2+ ions. In other words, the results indicate that the phase separation has occurred in the studied glass. That is that the rich fluorine phase with a lower optical basicity has emerged from the interpenetrating oxide phase. As mentioned above, the low optical basicity promoted the reduction from Eu3+ to Eu2+, i.e., the concentration of Eu2+ in rich fluoride is higher than that in dominant oxide phase. This is why the intensity of Eu2+ in Fig. 6 is obviously stronger than those in Fig. 3. The high symmetry properties of fluoride increase the splitting degree. Thus, the luminescence mechanism of Eu2+ ions under 330–360 nm excitation also changes along with it.
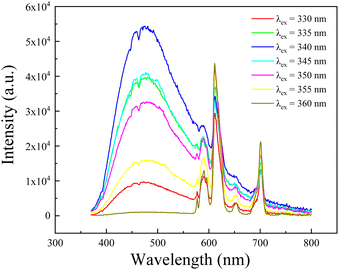 |
| Fig. 6 Emission spectra for Eu2+ ions in dependence on excitation wavelength from 330 nm to 360 nm with an interval of 5 nm, respectively, measured for the Eu-doped 40SiO2–25Al2O3–20BaF2–15Na2O glass (for interpretation of the references to color in this figure legend, the reader referred to the web version of this article). | |
As using 330, 335, and 340 nm to excite the sample, the component of 5d level captured transition electron is eg state, and the larger the wavelength of incident light, the closer the incident excitation energy is to the peak of eg state. Thus, the emission intensity of Eu2+ ions increases with the enhancing excitation wavelength. But, as the incident light wavelength increases further, excitation electrons jump into t2g state. Then, as mentioned above, the incident light from 340 to 360 nm deviates from the peak of excitation band. So, the emission intensity of Eu2+ ions decreases with the enhancing excitation wavelength. The increase in relative concentration of Eu2+ ions leads to richer white light emission shown as in Fig. 7. These results indicate that, just by Eu-individually-doped aluminosilicate oxyfluoride glass, various color emissions can be achieved, and the sample has extensive application prospect in solid-state light devices.
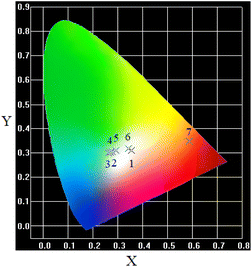 |
| Fig. 7 Dependence of CIE chromaticity diagram on excitation wavelength for the Eu-doped 40SiO2–25Al2O3–20BaF2–15Na2O glass. The numbers 1 to 7 represent the chromaticity coordinates under 330 to 360 nm excitation (for interpretation of the references to color in this figure legend, the reader referred to the web version of this article). | |
4 Conclusions
Eu-doped aluminosilicate oxyfluoride glasses have been prepared by the melt-quenching method. In the glass prepared in the air condition, the reduction of Eu3+ to Eu2+ ions occurred in the air condition. It is confirmed that the presence of significant amount fluoride in the glass composition is critical to promote this reduction process due to it causes the decrease of optical basicity for the studied glass system. With the increasing incident light wavelength (in the studied excitation wavelength range 320–360 nm), the emission intensity of Eu3+ ions decreases before increases in the red wavelength range. At the same time, it was noticed that the fluorescence time first increases before decreases. In addition, the heterogeneous distribution of Eu2+ ions due to phase separation is observed in the studied luminescence glass system. The results indicate that the spectral properties depend on the excitation mode. The colorful emission of Eu-individual-doped aluminosilicate oxyfluoride glasses may bring potential applications in light-emitting diode systems, three-dimensional displays, etc.
Author contributions
Lei Liu: investigation, writing – original draft. Xianying Shao: investigation, writing – original draft. Zhenyuan Zhang: investigation, original draft. Jiayu Liu: investigation, original draft. Yuebo Hu: supervision, data curation, methodology, investigation, writing – original draft – review & editing, validation. Chaofeng Zhu: supervision, data curation, methodology, writing – review & editing, validation.
Conflicts of interest
The authors declare that they have no known competing financial interests or personal relationships that could have appeared to influence the work reported in this paper.
Acknowledgements
This work is supported by the National Natural Science Foundation of China (No. 61368007), the Natural Science Foundation of Shandong Province (Grant No. ZR2019MEM053).
References
- R. Klement, K. Drdlíková, M. Kachlík, D. Drdlík, D. Galusek and K. Maca, Photoluminescence and optical properties of Eu3+/Eu2+-doped transparent Al2O3 ceramics, J. Eur. Ceram. Soc., 2021, 41(9), 4896–4906, DOI:10.1016/j.jeurceramsoc.2021.03.029.
- G. Blasse and B. C. Grabmaier, Luminescence Materials, Springer, Berlin, 1994 Search PubMed.
- Y. D. Ma, X. S. Peng, M. Z. Fei, W. N. Zhang, L. M. Teng, F. F. Hu, R. F. Wei and H. Guo, Adjustable white luminescence and high thermal stability in Eu2+/Eu3+/Tb3+/Al co-doped aluminosilicate oxyfluoride glass, J. Alloys Compd., 2020, 846, 156435, DOI:10.1016/j.jallcom.2020.156435.
- F. Liu, J. D. Budai, X. F. Li, J. Z. Tischler, J. Y. Howe, C. J. Sun, R. S. Meltzer and Z. W. Pan, New Ternary Europium Aluminate Luminescent Nanoribbons for Advanced Photonics, Adv. Funct. Mater., 2013, 23(16), 1998–2006, DOI:10.1002/adfm.201202539.
- T. Hu, Y. Gao, M. S. Molokeev, Z. G. Xia and Q. Y. Zhang, Eu2+ Stabilized at Octahedrally Coordinated Ln3+ Site Enabling Red Emission in Sr3LnAl2O7.5 (Ln = Y or Lu) Phosphors, Adv. Opt. Mater., 2021, 9(9), 2100077, DOI:10.1002/adom.202100077.
- G. J. Gao, S. Krolikowski, M. Y. Peng and L. Wondraczek, Tailoring super-broad photoluminescence from Eu2+ and dual-mode Eu2+/Eu3+-doped alkaline earth aluminoborate glasses through site-similarity and ligand acidity, J. Lumin., 2016, 180, 234–240, DOI:10.1016/j.jlumin.2016.08.025.
- P. Dorenbos, Energy of the first 4f7 → 4f65d transition of Eu2+ in inorganic compounds, J. Lumin., 2003, 104(4), 239–260, DOI:10.1016/S0022-2313(03)00078-4.
- S. S. Liang, P. P. Dang, G. G. Li, Y. Wei, Y. Wei, H. Z. Lian and J. Lin, New Insight for Luminescence Tuning Based on Interstitial Sites Occupation of Eu2+ in Sr3Al2-xSixO5-xNxCl2 (x = 0-0.4), Adv. Opt. Mater., 2018, 6(22), 1800940, DOI:10.1002/adom.201800940.
- Y. Pan, X. Xie, Q. Huang, C. Gao, Y. Wang, L. Wang, B. Yang, H. Su, L. Huang and W. Huang, Inherently Eu2+/Eu3+ Codoped Sc2O3 Nanoparticles as High-Performance Nanothermometers, Adv. Mater., 2018, 30(14), 1705256, DOI:10.1002/adma.201705256.
- D. Stefańska and P. J. Dereń, High Efficiency Emission of Eu2+ Located in Channel and Mg-Site of Mg2Al4Si5O18 Cordierite and Its Potential as a Bi-Functional Phosphor toward Optical Thermometer and White LED Application, Adv. Opt. Mater., 2020, 8(22), 2001143, DOI:10.1002/adom.202001143.
- M. Liao, Q. Wang, Q. M. Lin, M. X. Xiong, X. Zhang, H. F. Dong, Z. P. Lin, M. R. Wen, D. Y. Zhu, Z. F. Mu and F. G. Wu, Na Replaces Rb towards High-Performance Narrow-Band Green Phosphors for Backlight Display Applications, Adv. Opt. Mater., 2021, 9(17), 2100465, DOI:10.1002/adom.202100465.
- Y. Wei, Z. Y. Gao, S. W. Liu, S. T. Chen, G. C. Xing, W. Wang, P. P. Dang, A. A. A. Kheraif, G. G. Li and J. Lin, Highly Efficient Green-to-Yellowish-Orange Emitting Eu2+-Doped Pyrophosphate Phosphors with Superior Thermal Quenching Resistance for w-LEDs, Adv. Opt. Mater., 2020, 8(6), 1901859, DOI:10.1002/adom.201901859.
- J. N. Spencer, D. Merrikin, S. J. A. Pope, D. J. Morgan, N. Carthey and D. M. Murphy, CW EPR Investigation of Red-Emitting CaS:Eu Phosphors: Rationalization of Local Electronic Structure, Adv. Opt. Mater., 2020, 8(22), 2001241, DOI:10.1002/adom.202001241.
- Z. Y. Lin, H. D. Zeng, Y. F. Yang, X. L. Liang, G. R. Chen and L. Y. Sun, The Effect of Fluorine Anions on the Luminescent Properties of Eu-Doped Oxyfluoride Aluminosilicate Glasses, J. Am. Ceram. Soc., 2010, 93(10), 3095–3098, DOI:10.1111/j.1551-2916.2010.04067.x.
- Y. B. Hu, R. J. Dou and J. B. Qiu, Spectroscopic properties and energy transfers in Tb3+/Ho3+/Yb3+ tri-doped oxyfluoride silicate glasses, J. Non-Cryst. Solids, 2015, 420, 12–16, DOI:10.1016/j.jnoncrysol.2015.04.007.
- Y. B. Hu, J. B. Qiu, Z. G. Song, Z. W. Yang, Y. Yang, D. C. Zhou, Q. Jiao and C. S. Ma, Spectroscopic properties of Tm3+/Er3+/Yb3+ co-doped oxyfluorogermanate glasses containing silver nanoparticles, J. Lumin., 2014, 145, 512–517, DOI:10.1016/j.jlumin.2013.08.022.
- K. Takahashi, Progress in Science and Technology on Photostimulable BaFX:Eu2+ (X = Cl, Br, I) and Imaging Plates, J. Lumin., 2002, 100(1–4), 307–315, DOI:10.1016/s0022-2313(02)00447-7.
- D. Dutzler, M. Seibald, D. Baumann and H. Huppertz, Alkali Lithosilicates: Renaissance of a Reputable Substance Class with Surprising Luminescence Properties, Angew. Chem., Int. Ed., 2018, 57(41), 13676–13680, DOI:10.1002/anie.201808332.
- X. Y. Sun, X. C. Le, Z. H. Xiao, X. H. Shi, W. F. Wang, Z. F. Hu, Q. M. Yang, R. F. Wei and H. Guo, Self-reduction of Eu3+ to Eu2+ in europium-doped Li2B4O7 glass prepared in air, J. Am. Ceram. Soc., 2020, 103(5), 3119–3125, DOI:10.1111/jace.17012.
- E. Malchukova and B. Boizot, Reduction of Eu3+ to Eu2+ in aluminoborosilicate glasses under ionizing radiation, Mater. Res. Bull., 2010, 45(9), 1299–1303, DOI:10.1016/j.materresbull.2010.04.027.
- T. K. Pietrzak, A. Gołębiewska, J. Płachta, M. Jarczewski, J. Ryl, M. Wasiucionek and J. E. Garbarczyk, Photoluminescence of partially reduced Eu2+/Eu3+ active centers in a NaF–Al2O3–P2O5 glassy matrix with tunable smooth spectra, J. Lumin., 2019, 208, 322–326, DOI:10.1016/j.jlumin.2018.12.060.
- Y. Gao, S. Murai, K. Shinozaki, J. B. Qiu and K. Tanaka, Phase-Selective Distribution of Eu2+ and Eu3+ in Oxide and Fluoride Crystals in Glass-Ceramics for Warm White-Light-Emitting Diodes, ACS Appl. Electron. Mater., 2019, 1(6), 961–971, DOI:10.1021/acsaelm.9b00129.
- J. Yan, L. X. Ning, Y. C. Huang, C. M. Liu, D. J. Hou, B. B. Zhang, Y. Huang, Y. Tao and H. B. Liang, Luminescence and electronic properties of Ba2MgSi2O7:Eu2+: a combined experimental and hybrid density functional theory study, J. Mater. Chem. C, 2014, 2(39), 8328–8332, 10.1039/c4tc01332h.
- Y. Gao, J. B. Qiu and D. C. Zhou, Investigation of optical properties: Eu with Al codoping in aluminum silicate glasses and glass-ceramics, J. Am. Ceram. Soc., 2017, 100(7), 2901–2913, DOI:10.1111/jace.14807.
- K. Biswas, S. Balaji, D. Ghosh, A. D. Sontakke and K. Annapurna, Near-infrared frequency down-conversion and cross-relaxation in Eu2+/Eu3+–Yb3+ doped transparent oxyfluoride glass and glass-ceramics, J. Alloys Compd., 2014, 608, 266–271, DOI:10.1016/j.jallcom.2014.04.126.
- Y. B. Hu, S. W. Qiu, Y. Gao and J. B. Qiu, Crystallization and spectroscopic properties in Er3+ doped oxyfluorogermanate glass ceramics containing Na, Opt. Mater., 2015, 45, 82–86, DOI:10.1016/j.optmat.2015.03.014.
- Y. B. Hu, X. Y. Shao, Z. Y. Wang, X. L. Xu, X. J. Han, H. Z. Tao and Y. Z. Yue, BaAl2Si2O8 polymorphs and a novel reversible transition of BaAlF5 in supercooled oxyfluoride aluminosilicate liquids, J. Eur. Ceram. Soc., 2021, 41(14), 7282–7287, DOI:10.1016/j.jeurceramsoc.2021.07.021.
- M. Nogami, T. Yamazaki and Y. Abe, Fluorescence properties of Eu3+ and Eu2+ in Al2O3–SiO2 glass, J. Lumin., 1998, 78(1), 63–68, DOI:10.1016/s0022-2313(97)98281-8.
- X. T. Li, P. L. Li, C. J. Liu, L. Zhang, D. J. Dai, Z. H. Xing, Z. P. Yang and Z. J. Wang, Tuning the luminescence of Ca9La(PO4)7: Eu2+ via artificially inducing potential luminescence centers, J. Mater. Chem. C, 2019, 7(46), 14601–14611, 10.1039/c9tc04828f.
- M. Nogami and Y. Abe, Enhanced emission from Eu2+ ions in sol-gel derived Al2O3–SiO2 glasses, Appl. Phys. Lett., 1996, 69(25), 3776–3778, DOI:10.1063/1.116995.
- Y. B. Hu, Y. Shen, C. F. Zhu, S. J. Liu, H. Liu, Y. F. Zhang and Y. Z. Yue, Optical bandgap and luminescence in Er3+ doped oxyfluoro-germanate glass-ceramics, J. Non-Cryst. Solids, 2021, 555, 120533, DOI:10.1016/j.jnoncrysol.2020.120533.
- G. V. Prakash, Absorption spectral studies of rare earth ions (Pr3+, Nd3+, Sm3+, Dy3+, Ho3+ and Er3+) doped in NASICON type phosphate glass, Na4AlZnP3O12, Mater. Lett., 2000, 46(1), 15–20, DOI:10.1016/S0167-577X(00)00135-X.
- J. A. Duffy and M. D. Ingram, An interpretation of glass chemistry in terms of the optical basicity concept, J. Non-Cryst. Solids, 1976, 21(3), 373–410, DOI:10.1016/0022-3093(76)90027-2.
- A. S. Borukhovich and A. V. Troshin, Methods of Synthesis of Europium Monoxide, in Europium Monoxide, Springer Series in Materials Science, 2018, 265, pp. 37–67, DOI:10.1007/978-3-319-76741-3_2.
- G. J. McCarthy and W. B. White, On the stabilities of the lower oxides of the rare earths, J. less-common met., 1970, 22(4), 409–417, DOI:10.1016/0022-5088(70)90128-1.
- Z. G. Song, D. C. Zhou, Z. W. Yang, Z. Y. Yin, R. F. Wang, J. H. Shang, K. Lou, Y. Y. Xu, Y. B. Hu and J. B. Qiu, Correlation between optical basicity and properties of broadband infrared fluorescence of bismuth-doped germanate glasses, J. Chin. Ceram. Soc., 2010, 38(11), 2133–2137 CAS.
- K. G. F. Baucke and A. J. Duffy, The effect of basicity on redox equilibria in molten glasses, Phys. Chem. Glasses, 1991, 32, 211–218 Search PubMed.
- C. Bocker, S. Bhattacharyya, T. Höche and C. Rüssel, Size distribution of BaF2 nanocrystallites in transparent glass ceramics, Acta Mater., 2009, 57(20), 5956–5963, DOI:10.1016/j.actamat.2009.08.021.
- J. A. Duffy, A common optical basicity scale for oxide and fluoride glasses, J. Non-Cryst. Solids, 1989, 109(1), 35–39, DOI:10.1016/0022-3093(89)90438-9.
- P. P. Pawar, S. R. Munishwar and R. S. Gedam, Eu2O3 doped bright orange-red luminescent lithium alumino-borate glasses for solid state lighting, J. Lumin., 2018, 200, 216–224, DOI:10.1016/j.jlumin.2018.04.026.
- M. Fockele, J. F. Ahlers, F. Lohse, J.-M. Spaeth and R. H. Bartram, Optical properties of atomic thallium centers in alkali halides, J. Phys. C: Solid State Phys., 1985, 18(9), 1963–1974, DOI:10.1088/0022-3719/18/9/029.
- N. Víjaya, K. Upendra Kumar and C. K. Jayasankar, Dy3+-doped zinc fluorophosphate glasses for white luminescence applications, Spectrochim. Acta, Part A, 2013, 113, 145–153, DOI:10.1016/j.saa.2013.04.036.
- B. Di Bartolo, Optical Interactions in Solids, Wiley, New York, 1968, Ch. 18 Search PubMed.
- J. A. Jiménez, S. Lysenko, H. Liu, E. Fachini and C. R. Cabrera, Investigation of the influence of silver and tin on the luminescence of trivalent europium ions in glass, J. Lumin., 2010, 130(1), 163–167, DOI:10.1016/j.jlumin.2009.08.007.
|
This journal is © The Royal Society of Chemistry 2023 |
Click here to see how this site uses Cookies. View our privacy policy here.