DOI:
10.1039/D3RA05664C
(Paper)
RSC Adv., 2023,
13, 28686-28702
Facile synthesis of a new covalent organic nanosheet (CON-KEY1) based on polyamide links as an effective heterogeneous catalyst in C–C cross coupling reactions
Received
19th August 2023
, Accepted 23rd September 2023
First published on 2nd October 2023
Abstract
C–C coupling reactions represent a fundamental synthetic methodology widely employed in academic and industrial settings. Herein, we present a report on developing and synthesizing a heterogeneous catalyst that is environmentally compatible and has recycling capabilities. Furthermore, the utilization of this catalyst for C–C coupling reactions was explored. A novel amide-based CON was prepared via the reaction of a novel [2,2′-bipyridine]-5,5′-diamine (BDA) and 1,3,5-tris(4-carboxyphenyl) (TCB). TCB was activated with carbonyl diimidazole (CDI) and then reacted with BDA to synthesize favorable CON (i.e., CON-KEY1). Finally, the CON synthesized was reacted with palladium chloride ions, and the palladium-containing organocatalytic complex was decorated with the abbreviated Pd/CON-KEY1. This new heterogeneous complex was fully characterized through the required techniques, including FT-IR, EDX, XRD, TEM, SEM, ICP, TGA-DTA, N2 isotherms, and elemental mapping analysis. Computer simulation results include a multi-sheet 2D framework proposed by CON-KEY1. As a result, palladium ions were found to be arranged between the layers and on the CON surface. This heterogeneous complex functioned as a catalyst precursor in both the Suzuki–Miyaura coupling reaction of aryl boronic acids with aryl halides and the Heck reaction of aryl halides with acrylate derivatives or styrene. The desired coupling products with various functional groups were successfully attained with excellent yields of up to 98%. Simple set-up, improved yields, short reaction times, non-toxic solvents, catalyst durability, and high turnover frequency are among the distinct advantages of this synthetic method. Some other outstanding features of this catalytic system include convenient separation of catalysts and products, high yields, almost complete conversion, high selectivity, and good turnover frequency (TOF). The results show that the highest product efficiency in the reaction was achieved in the shortest possible time using Pd/CON-KEY1. Theoretical studies demonstrated the precedence of the palladium complexation with nitrogen atoms of CON-KEY1 rather than oxygen ones. Natural Bond Orbital (NBO) analysis affirmed that the system with Pd–N bonds (Eg = 0.089 eV) is more reactive with high electron conductivity compared to the Pd–O system (Eg = 0.120 eV).
1. Introduction
Covalent organic frameworks (COFs) and Covalent Organic Nanosheets (CONs) are emerging types of organic polymers, with permanent porosity and highly ordered structures, which are two- or three-dimensional (2D or 3D) porous crystalline structures prepared through reticular chemistry. In contrast to other polymers, a key feature of COFs and CONs is that they are structurally predesignable, synthetically controllable, and functionally manipulable. Indeed, topological design diagrams provide a geometric guide for structural tiling of extended porous polygons, and polycondensation reactions guide synthetic routes for building predesigned primary and higher-order structures.1–8
Due to their extraordinary properties in structural engineering and ordered structures, CONs are good candidates for acting as ligands, forming metal complexes, and depositing metal nanoparticles on substrates.9 In fact, due to nitrogen groups in the bonds between structures, CONs may form complexes that can be used as excellent supports in catalysis-related reactions.2,10–13 Moreover, a critical point in using covalent organic frameworks as supports for heterogeneous catalytic systems is their high specific surface area and facile separation from the reaction mixture.12,14
Our aim for using CONs as catalyst supports is that it provides a unique basis for using these mesoporous polymers in catalysis. A cross-coupling reaction is a transformation in which two fragments are joined together with the help of a metal catalyst. One of the most important reasons for using palladium-containing catalysts is their ability to perform carbon–carbon and carbon–nitrogen cross-coupling reactions.15–20
Biaryls and cinnamic acid derivatives are two classes of organic compounds synthesized by two-component condensation reactions, such as cross-coupling. These units are increasingly important in organic chemistry due to their presence in the structures of various natural products and bioactive compounds.2,21,22 As a result, several studies have been carried out on synthesizing various organic materials containing these moieties using the Suzuki and Heck reactions. Due to the application of different heterogeneous and homogeneous catalysts, bioactivity, and structural properties of Suzuki and Heck coupling compounds, several specific processes have been developed (Table 1).23–25
Table 1 Mizoroki–Heck cross-coupling reactions in the pharmaceutical industry
Structure |
Company |
Usage |
Scale |
Catalyst/reaction conditions |
Reference |
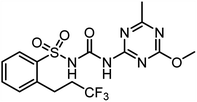 |
Novartis |
Herbicide |
Multi – ton |
Pd(dba)2 25 °C ton 200; TOF ca. 50 h−1 |
24 and 25 |
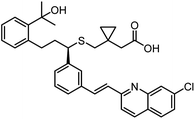 |
Merck |
Asthma drug |
— |
Pd(OAC)2 Et2N, MeCN 85 °C |
26 |
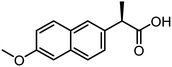 |
Albermarle, Hoechst AG |
Non-steroidal antiinflammatory drug |
Multi – ton |
PdCl2, CuCl2 |
24 and 27–29 |
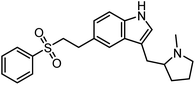 |
Pfizer |
Migraines |
Multi-kg |
Pd(OAC)2/P(o-tolyl)3 Et3N, DMF, reflux 80% yield |
30 |
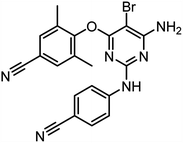 |
Johnson & Johnson |
HIV |
6k L |
Pd(OAc)2/tri-o-toly-phosphine |
25–31 |
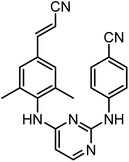 |
Johnson & Johnson |
HIV |
6k L |
Pd(OAc)2, P(o-tol)3, Et3N, CH3CN, 150 °C |
26–31 |
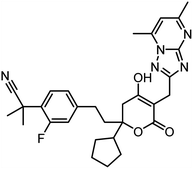 |
Pfizer |
Hepatitis C polymerase inhibitor |
40 kg scale |
Pd(OAC)2 LiCl LiOAc Et3N |
32 |
Despite reported synthetic methods for these catalysts, it is believed that much research is still needed in this area. Therefore, there is a need to develop additional environmentally friendly, atomically efficient, sustainable, and high-yielding methods.
In this study, a novel two-dimensional Covalent Organic Nanosheet (CON-KEY1) based on synthetic polyamide compounds was designed and fabricated. A carbonyldiimidazole (CDI) activator compound activated the carboxylic acid groups. In the next step, we synthesized a new CON-KEY1 and then used it to support preparing organopalladium nanocatalysts. In this paper, we report the preparation of new CON-KEY1 based on polyamide links as an effective heterogeneous catalyst for Suzuki–Miyaura coupling reaction of aryl boronic acids with aryl halides and Heck reaction of aryl halides with acrylate derivatives or styrene and excellent results have been obtained with high efficiency.
2. Experimental
2.1. Synthesis of CON-KEY1 (5)
COF-KEY1 5 was synthesized by reacting CDI 2, [2,2′-bipyridine]-5,5′-diamine (BDA) 4 and 1,3,5-tris(4-carboxyphenyl)benzene 1 (TCB). For this purpose, TCB 1 (29.0 mmol) and CDI 2 (30.0 mmol) were dissolved in DMF (50 mL) and sonicated for 30 min. The reaction was then shaken at 60 °C for 6 h. BDA 4 was then added and shaken for 24 h. The solid that formed was filtered and washed with dichloromethane. The CON 5 thus produced was then placed in dichloromethane (25 mL), and the DMF was removed for 24 h. Finally, the prepared CON 5 was rinsed with dichloromethane and dried in an oven at 50 °C for 6 h (Scheme 1).26
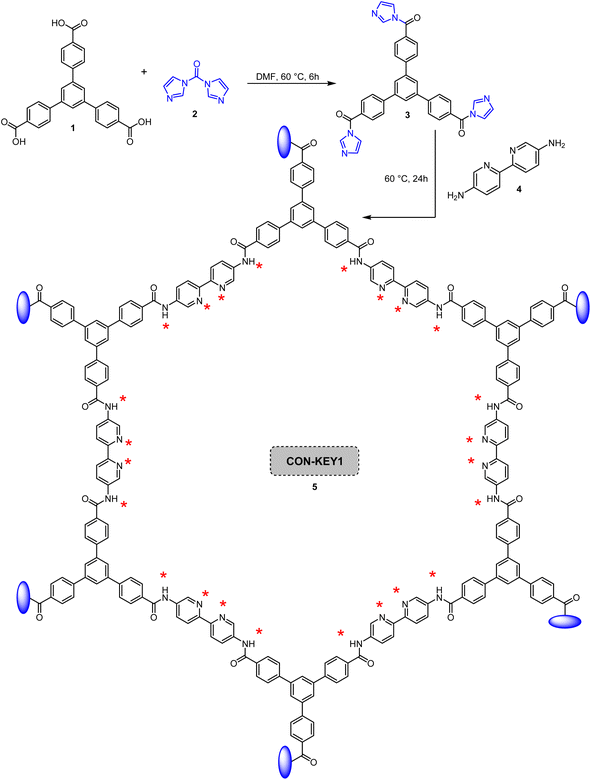 |
| Scheme 1 Preparation of CON-KEY1 5. | |
2.2. Preparation of Pd/CON-KEY1 6
2.0 g of CON-KEY1 5 was poured into a beaker, and 60 mL of acetonitrile was added and sonicated for 1 h until wholly dispersed. 50 mg of the palladium chloride salt was then dispersed in 10 mL of acetonitrile and added to the nanoparticles 5 dispersion, and the reaction mixture was refluxed at 50 °C for 24 h. Finally, the Pd/CON-KEY1 6 was separated by centrifugation, washed with ethanol, and dried in an oven at 50 °C for 12 h. The Pd loading of the material was estimated to be 3.14 mmol g−1 by the ICP-OES technique (Scheme 2).
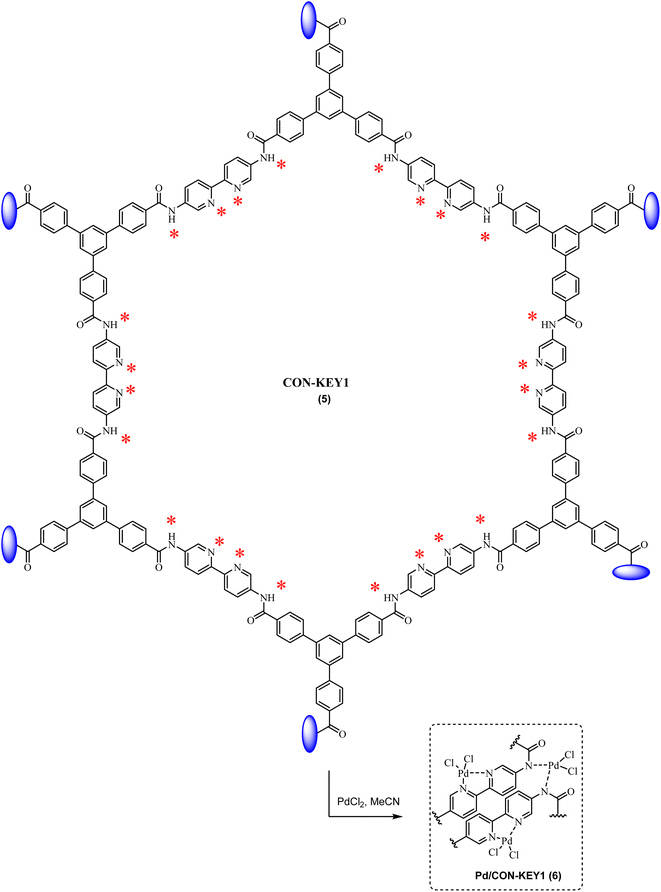 |
| Scheme 2 Preparation of Pd/CON-KEY1 6. | |
2.3. General procedure for the Mizoroki–Heck reaction
An oven-dried 25 mL round bottom flask with a magnetic stirrer bar was charged Pd/CON-KEY1 6 (30 mg) in DMF (5 mL). The reaction mixture was stirred for 3 min under argon. To this reaction mixture, base K2CO3 (2 mmol), aryl iodide (1 mmol), olefin (1–2.5 mmol), and DMF (5 mL) were added, and the reaction mixture was stirred at the indicated temperature and time. TLC monitored the reaction. The reaction mixture was quenched with water (10 mL) and extracted with ethyl acetate (10 mL). The organic layer was washed with brine, dried over Na2SO4, and concentrated under vacuum. The residue was purified by column chromatography on silica gel to give the desired product.
2.4. General procedure for the catalysis of the Suzuki–Miyaura coupling
To a solution of aryl halide (0.078 g, 0.5 mmol), aryl boronic acid (0.073 g, 0.6 mmol), K3PO4 (0.127 g, 0.6 mmol) in DMF or dioxane and water (1
:
1, 10 mL), was added Pd/CON-KEY1 6 (50 mg). The resulting mixture was heated by stirring at 100 °C under argon for 2–6 h. The reaction mixture was quenched by adding water and extracted with ethyl acetate (3 × 20 mL). The combined extract was washed with saturated brine and dried over anhydrous Na2SO4. The organic layer was concentrated under vacuum, and the crude product was purified by column chromatography on silica gel (100–200 mesh, petroleum ether: 2% ethyl acetate) to give the pure desired product.
2.5. Spectral information of some reaction products
2.5.1 2-Propenoic acid-3-phenyl, ethyl ester. Obtained as a colorless oil, 1H NMR (400 MHz, CDCl3, δ): 7.65–7.73 (d, J = 16 Hz, 1H), 7.33–7.58 (m, 5H), 6.45 (d, J = 16 Hz, 1H), 4.23 (q, J = 7.2 Hz, 2H), 1.33 (t, J = 7.1 Hz, 3H); 13C NMR (100 MHz, CDCl3, δ): 168.3, 146.5, 135.3, 131.3, 126.8, 127.7, 119.2, 61.5, 15.2.
2.5.2 (E)-1,2-Diphenylethene. Obtained as a white solid, mp: 123.5–124.5 °C, 1H NMR (400 MHz, CDCl3, δ) 7.55–7.3 (m, 10H), 7.13 (s, 2H); 13C NMR (100 MHz, CDCl3, δ) 138.3, 129.7, 127.5, 126.5.
2.5.3 Ethyl (E)-3-(4-methoxyphenyl)acrylate. Obtained as a colorless oil, 1H NMR (400 MHz, CDCl3, δ) 7.63 (d, J = 15.9 Hz, 1H), 7.47 (d, J = 8.7 Hz, 2H), 6.89 (d, J = 8.9 Hz, 2H), 6.30 (d, J = 16 Hz, 1H), 4.25 (q, J = 7 Hz, 2H), 3.83 (s, 3H), 1.31 (t, J = 7.2 Hz, 3H); 13C NMR (100 MHz, CDCl3, δ) 167.33, 161.3, 144.2, 129.6, 127.1, 115.7, 114.3, 60.3, 55.32, 14.3.
2.5.4 (E)-1-Methoxy-4-styrylbenzene. Obtained as a white solid, mp: 135–136 °C, 1H NMR (400 MHz, CDCl3, δ) 7.53–7.24 (m, 7H), 7.04 (d, J = 7.45 Hz, 2H), 6.91 (d, J = 8.45 Hz, 2H), 6.32 (d, J = 16 Hz, 1H), 4.25 (q, J = 7.2 Hz, 2H), 3.85 (s, 3H), 1.33 (t, J = 7.2 Hz, 3H); 13C NMR (100 MHz, CDCl3, δ) 158.3, 138.2, 131.1, 129.1, 128.1, 127.7, 127.1, 126.5, 126.3, 114.1, 55.3.
2.5.5 2-Propenoic acid, 3-(4-methyl phenyl)-ethyl ester. Obtained as a colorless oil, 1H NMR (400 MHz, CDCl3, δ): 7.55 (d, J = 16.0 Hz, 1H), 7.33 (d, J = 8.0 Hz, 2H), 7.08 (d, J = 7.8 Hz, 2H), 6.30 (d, J = 15.9 Hz, 1H), 4.14 (q, J = 7.1 Hz, 2H), 2.27 (s, 3H), 1.25 (t, J = 7.1 Hz, 3H); 13C NMR (100 MHz, CDCl3, δ): 168.1, 145.4, 141.3, 130.9, 129.5, 128.1, 118.3, 76.7, 76.5, 61.5, 21.5, 14.5.
2.5.6 2-Propenoic acid, 3-(4-fluorophenyl)-ethyl ester. Obtained as a colorless oil, 1H NMR (400 MHz, CDCl3, δ) 7.65 (d, J = 16 Hz, 1H), 7.55–7.46 (m, 2H), 7.30–7.05 (m, 2H), 6.37 (d, J = 16.17 Hz, 1H), 4.27 (q, J = 7.20 Hz, 2H), 4.10 (q, J = 7.07 Hz, 2H, E
:
Z ratio 8.6
:
1), 1.34 (t, J = 7.07 Hz, 3H), 1.15 (t, J = 7.2 Hz, 3H, E
:
Z ratio 8.8
:
1); 13C NMR (100 MHz, CDCl3, δ) 167.7, 167.2–162.3 (d, JC–F = 252.6 Hz), 144.1, 132.1, 131.0, 130.5, 129.7, 129.5, 118.7, 116.1, 115.5, 115.3, 114.6, 60.2, 60.0, 15.1, 13.9.
2.5.7 4-Chloro-1,1′-biphenyl. Obtained as a white solid, mp: 77–78.5 °C, 1H NMR (400 MHz, CDCl3, δ) −7.61–7.38 (m, 9H); 13C NMR (100 MHz, CDCl3, δ) – 140.0, 139.7, 134.3, 129.7, 128.3, 126.8.
2.5.8 1,1-Biphenyl. Obtained as a white solid, mp: 67–68 °C, 1H NMR (200 MHz, CDCl3, δ) 7.62–7.56 (m, 4H), 7.49–7.3 (m, 6H).
2.5.9 4-Chloro-4-methyl-1,1-biphenyl. Obtained as a white solid, mp: 121–123 °C, 1H NMR (400 MHz, CDCl3, δ) 7.55–7.43 (m, 6H), 7.25–7.24 (m, 2H), 2.44 (s, 3H); 13C NMR (100 MHz, CDCl3, δ) 144.1, 142.5, 141.3, 138.4, 131.5, 129.8, 128.4, 128.1, 127.7, 127.5, 127.4, 127.3, 126.6, 115.5, 87.5, 83.3, 68.9, 65.2, 64.3.
2.5.10 1-(4-Methoxyphenyl)-naphthalene. Obtained as a white solid, mp: 114–115 °C, 1H NMR (400 MHz, CDCl3, δ) 7.95–7.83 (m, 3H), 7.55–7.42 (m, 6H), 7.06–7.02 (d, J = 8.84 Hz, 2H), 3.93 (s, 3H); 13C NMR (100 MHz, CDCl3, δ) 132.1, 128.3, 127.4, 126.8, 126.1, 125.6, 125.87 125.4, 114.1, 55.2.
2.5.11 4-Methoxy-1,1-biphenyl. Obtained as a white solid, mp: 89–91 °C, 1H NMR (400 MHz, CDCl3, δ) 7.58–7.51 (m, 4H), 7.48–7.32 (m, 3H), 7.00 (d, J = 8.84 Hz, 2H), 3.87 (s, 3H); 13C NMR (100 MHz, CDCl3, δ) 129.1, 128.1, 126.7, 126.3, 112.2, 55.3.
2.5.12 4-Methyl-1,1′-biphenyl. Obtained as a white solid, mp:−49 °C, 1H NMR (400 MHz, CDCl3, δ): 7.17–7.63 (m, 9H), 2.39 (s, 3H).
2.6. Computational details
The structures of CON-KEY1 5, Pd–N/CON-KEY1 6, and Pd–O/CON-KEY1 6 were investigated by DFT calculations using Gaussian16 at B3LYP/lanl2dz level of theory, and initial optimizations were performed by HyperChem 8. Besides, the optimized structures binding energies, bond lengths, and bond angles were evaluated while not displaying any imaginary frequency. NBO analyses were done to indicate the frontier orbitals, band gaps, HOMO–LUMO representations, and the global descriptors.
3. Result and discussion
3.1. Catalyst characterization
Fig. 1 illustrates the Fourier transform infrared (FT-IR) spectra of CON-KEY1 5 and Pd/CON-KEY1 6 nanocomposites. Fig. 1 – blue displays the CON-KEY1s 5 characteristic IR peaks at 1654 and 3420 cm−1, attributed to C
O and N–H stretching, respectively. These peaks prove the establishment of the amide-based CON (Fig. 1-blue). The FT-IR spectrum of the synthesized catalyst shows a peak shift in the region of 1654 to 1668 cm−1, which is due to the binding of palladium metal and the formation of an organopalladium complex with a nanocomposite structure27–30 (Fig. 1-orange).
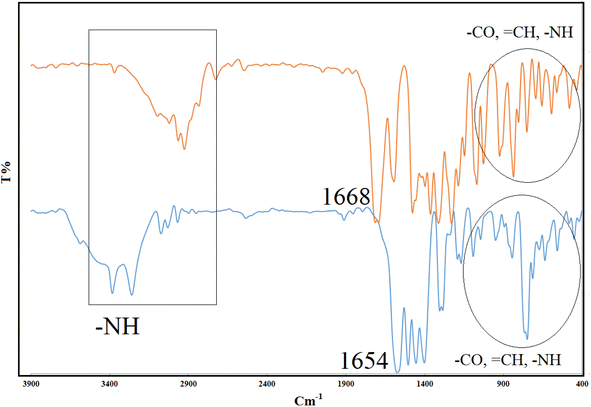 |
| Fig. 1 FT-IR spectrum of (blue) CON-KEY1 5, (orange) Pd/CON-KEY1 6. | |
Fig. 2 illustrates the TGA of the prepared CON-KEY1 5 (A) and Pd/CON-KEY1 6 (B). Fig. 2 shows considerable weight loss occurs at 130–250 °C for CON-KEY1 5 (thermogram A) and Pd/CON-KEY1 6 (thermogram B). This weight loss is attributed to the loss of organic moieties. The weight loss observed at a temperature of less than 250 °C is related to the evaporation of the trapped solvents in the synthesized nanocomposite structure. The decreasing slope in the 250 to 420 °C temperature range is due to removing functional groups in the corresponding nanocomposite structure. These results mean high thermal stability for this nanocatalyst and show the usefulness of using these structures as metal ions support.
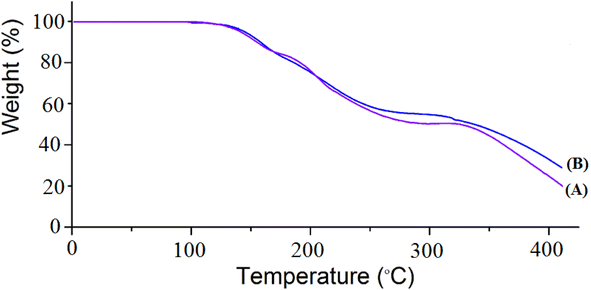 |
| Fig. 2 TGA plots of the (A) CON-KEY1 5 and (B) Pd/CON-KEY1 6. | |
The EDX analysis of Pd/CON-KEY1 6 (Fig. 3) shows some signals related to the C, O, N, Cl, and Pd atoms in the structures, which agree with our predicted pattern of the synthesized structures. Regarding the palladium ions, the nanocatalyst showed that it contains 9.36% palladium ions in the ICP test report, which indicates good compatibility with the structure.31,32
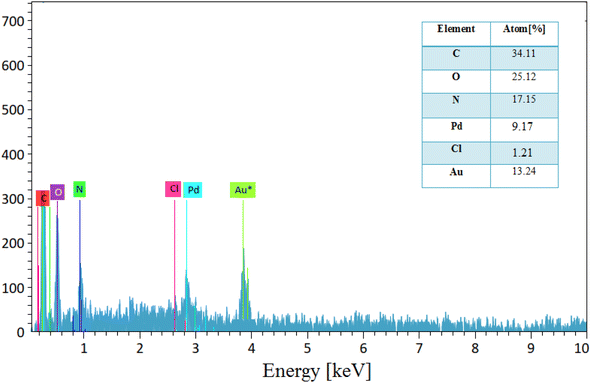 |
| Fig. 3 EDX spectrum of Pd/CON-KEY1 6. | |
The SEM photos of CON-KEY1 5 and Pd/CON-KEY1 6 (Fig. 4A and B) demonstrated the layered structures and morphology, indicating no specific changes after stabilizing palladium ions on this nanocomposite. Fig. 4A and B shows the excellent dispersion of palladium ions in the CON-KEY1 5 matrix. This nanocomposite must prevent the uniform accumulation of nanoparticles by trapping metal ions between the layers or inside its cavities.
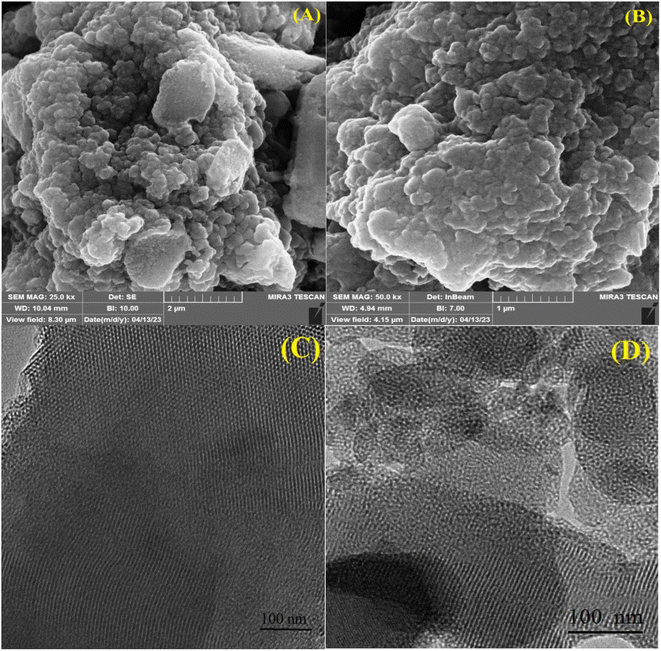 |
| Fig. 4 SEM images of (A and B) Pd/CON-KEY1 6, and (C and D) TEM images of Pd/CON-KEY1 6. | |
SEM and TEM images of Pd/CON-KEY1 6 (Fig. 4A–D) show the nature of the layered and porous structure of the catalytic nanocomposite. In the TEM images, the darker parts of the holes are due to the binding of palladium ions in the lattice structures of this nanocomposite.
As shown in the PXRD patterns (Fig. 5), the peak intensities of CON -KEY1 were decreased when PdCl2 was embedded. Considering that COFs are composed of light elements, like C, H, N, etc., the decreased intensities of the XRD peaks do not mean that the crystal structure of CON-KEY1 was destroyed after mixing with PdCl2. Similar observations have been reported for other COFs caused by amorphous alkyl chains on the walls or guest molecules incorporated within the pores of the COFs. Some different new diffraction peaks appeared related to palladium chloride attached to the structure.
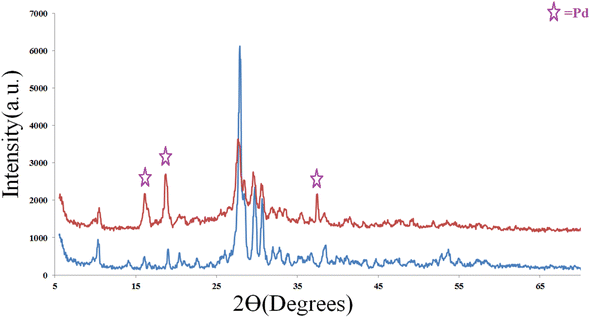 |
| Fig. 5 X-ray diffraction (PXRD) for (blue) CON-KEY1 5 and (red) Pd/CON-KEY1 6. | |
We applied elemental mapping to complete a study on the elemental composition and distribution of Pd/CON-KEY1 6 (Fig. 6). Moreover, the elemental mapping results agree with the EDX patterns. The elemental map image shows a well-uniform distribution of the exponential elements (N, O, C, Cl, and Pd) in the Pd/CON-KEY1 6 catalyst, which confirms the heterogenized Pd/CON-KEY1 6 complex formation. Furthermore, the distribution of Pd elements reveals the coordination of the resulting framework to the amide groups and confirms the formation of the catalyst support. Moreover, the distribution of C, N, O, Cl, and Pd species indicates that the final metal catalyst is uniformly coordinated to the poly amide and nitrogen inside ring aromatic ligands, forming a Pd complex on the catalyst support.
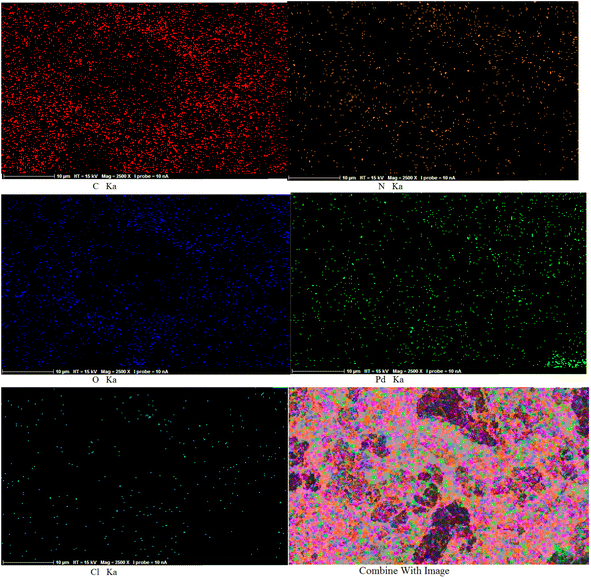 |
| Fig. 6 X-ray mapping images of Pd/CON-KEY1 6. | |
The N2 adsorption/desorption technique was used to determine surface structural parameters. The results of N2 adsorption/desorption were plotted in Fig. 7. The obtained surface area based on BET isotherm is 26.60 m2 g−1 for CON-KEY1 and 18.84 m2 g−1 for Pd/CON-KEY1 6. The total pore volume of the composite polymer is 1.49 cm3 g−1 for CON-KEY1 5 and 0.52 cm3 g−1 for Pd/CON-KEY1 6. Also, for studying the textural properties of Pd/CON-KEY1 6, the N2 adsorption–desorption isotherms were used (Fig. 7). The adsorption isotherm is type III, and the appearance of hysteresis loop shows the presence of mesoporous in the sample. The pore size distribution of CON-KEY1 5 and Pd/CON-KEY1 6 based on the BJH method is shown.
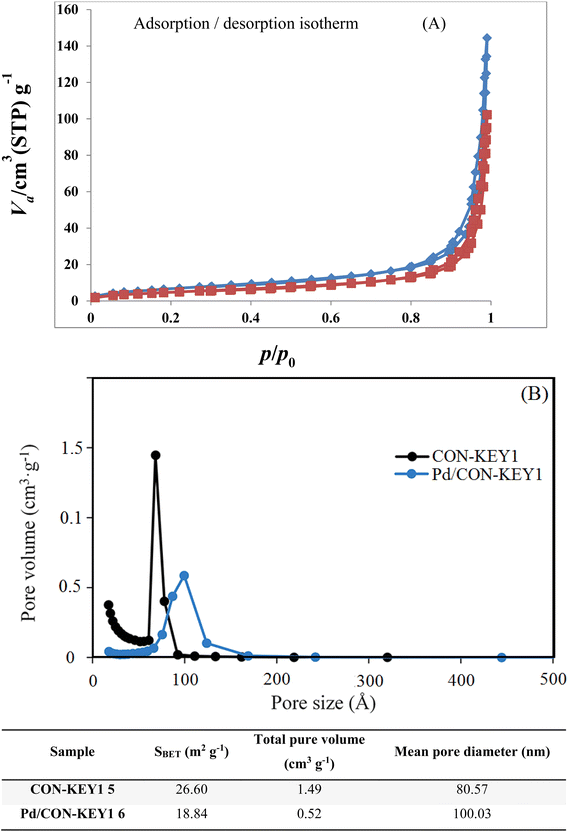 |
| Fig. 7 N2 adsorption–desorption isotherms (A) and the pore size distribution analysis based on the NLDFT model (B). | |
3.1.1 Geometric shape optimization. Initial optimization was performed to guess the geometry of CON-KEY1 5. Then full optimizations were done for the repeat units of CON-KEY1 5 and Pd/CON-KEY1 6. Two forms for Pd/CON-KEY1 6 were considered: One is a form with Pd–N bonds, and the other consists of Pd–O bonds (Fig. 8). According to the equation ΔEbinding = [Ecomplex − (ECON-KEY1 + EPdCl2)], the binding energies of the compounds were calculated in kcal mol−1 (Table 2). The results showed that the structure having Pd–N bonds in the bridge state is ∼48
658 kcal mol−1 more stable than the one with Pd–O bonds, and the priority to form complex is the coordination of Pd to N atoms. Based on the bond angle results, in each structure, there are two positions in the layers for Pd to make a square planner complex with pyridine moieties of CON-KEY1 5 in which their Pd–N and Pd–Cl bond lengths, as well as their bond angles, are almost the same. The bridge positions Pd–N and Pd–O bond lengths are 2.23 Å and 2.20–2.26 Å, respectively. In Pd–N/CON-KEY1 6a, the Pd–N distance in the layer (2.06 Å) is shorter than in the bridge position (2.23 Å). The same result happens for the Pd–Cl distances in both structures. Hence, the tetrahedral geometry in Pd–O/CON-KEY1 6b is slightly more distorted than Pd–N/CON-KEY1 6a.33
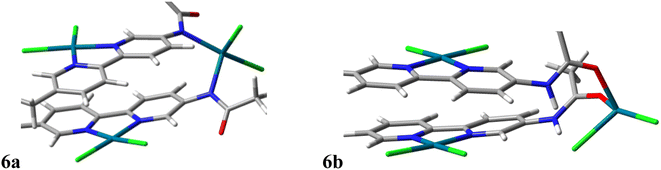 |
| Fig. 8 The optimized structures of (a) Pd–N/CON-KEY1 6a, and (b) Pd–O/CON-KEY1 6b at B3LYP/lanl2dz level of theory. | |
Table 2 Calculated ΔEbinding (kcal mol−1), selected bond lengths (Å), bond angles (°), and global descriptors of Pd–N/CON-KEY1 6a and Pd–O/CON-KEY1 6b
System |
Bond length/bond angles |
Global descriptors |
Pd–N/CON-KEY1 6a |
Pd–N(bridge) 2.23 |
EHOMO |
−0.030 |
Pd–Cl(bridge) 2.51, 2.55 |
ELUMO |
0.0595 |
Pd–N(layer) 2.06 |
Eg |
0.089 |
Pd–Cl(layer) 2.39–2.42 |
μ |
0.015 |
N–Pd–N (bridge) 98.7 |
η |
0.045 |
ΔEbinding = −8220.05 |
Cl–Pd–Cl(bridge) 110.0 |
S |
22.35 |
Cl–Pd–N(bridge) 102.0 |
ω |
0.0025 |
N–Pd–N(layer) 80.2 |
|
|
Cl–Pd–Cl(layer) 90.8 |
|
|
Cl–Pd–N(layer) 93.0 |
|
|
Pd–O/CON-KEY1 6b |
Pd–O 2.20, 2.26 |
EHOMO |
−0.241 |
Pd–Cl(bridge) 2.39, 2.53 |
ELUMO |
−0.120 |
Pd–N(layer) 2.06 |
Eg |
0.12 |
Pd–Cl(layer) 2.38–2.40 |
μ |
−0.181 |
O–Pd–O 85.6 |
η |
0.061 |
ΔEbinding = 40 438.18 |
Cl–Pd–Cl(bridge) 107.0 |
S |
16.53 |
Cl–Pd–O 106.0 |
ω |
0.269 |
N–Pd–N 80.1 |
|
|
Cl–Pd–Cl 91.2 |
|
|
Cl–Pd–N 94.1 |
|
|
In an attempt to find the reactivity and chemical potential of the systems, was done the NBO analysis. In addition, the energies of HOMO and LUMO frontier orbitals and the quantum molecular descriptors were calculated via Koopman's procedure.34 In CON-KEY1 5, HOMO is located on the pyridine rings and C
O parts, while LUMO is on the other aromatic rings (Fig. 9). The energy gaps (Eg) between HOMO and LUMO are given in Table 2. The more decrease the Eg, the more facilitates the release of electrons from HOMO and the addition of electrons to LUMO, and the more reactive the compound. Other criteria for the reactivity or stability of a compound are μ (chemical potential) and η (hardness). Pd–N/CON-KEY1 6a has larger μ (0.015 eV) and smaller Eg (0.089 eV) and η (0.045 eV) compared to Pd–O/CON-KEY1 6b. So it is inferred that Pd–N/CON-KEY1 6a shows more reactivity and electron conductivity. Hence, charge transfer readily occurs within the system. Also, the softness (S) of Pd–N/CON-KEY1 6a is 22.35 eV which is high compared to Pd–O/CON-KEY1 6b (16.53 eV). So the former is more polarizable than the latter.35 The systems electrophilicity index (ω) indicates the strong nucleophilic behavior of the Pd–N/CON-KEY1 6a contrasted with Pd–O/CON-KEY1 6b.
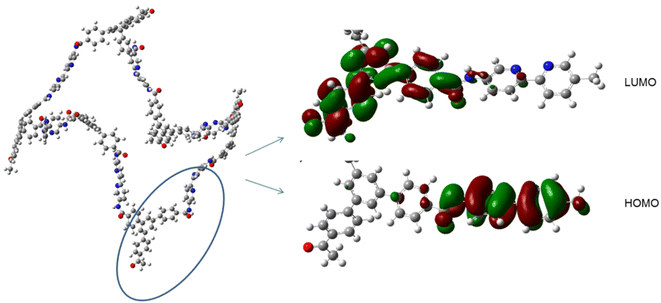 |
| Fig. 9 The calculated HOMO and LUMO frontier orbitals of CON-KEY1 5. | |
3.2. Catalytic application of Pd/CON-KEY1 6
To optimize the parameters involved in the Mizoroki–Heck reaction, a model reaction was carried out with iodobenzene 7a and styrene 8a, and the results are shown in Table 3.
Table 3 Optimization Screenings

|
Entry |
Catalyst 6 (mg) |
Solvent |
Base |
T (°C) |
Time (h) |
Yield (%) |
1 |
30 |
DMF |
K2CO3 |
120 |
6 |
96 |
2 |
30 |
Toluene |
K2CO3 |
120 |
6 |
69 |
3 |
30 |
DMSO |
K2CO3 |
120 |
6 |
65 |
4 |
30 |
NMP |
K2CO3 |
120 |
6 |
58 |
5 |
30 |
DMF |
Na2CO3 |
120 |
6 |
86 |
6 |
30 |
DMF |
KOH |
120 |
6 |
46 |
7 |
30 |
DMF |
NaOH |
120 |
6 |
39 |
8 |
30 |
DMF |
K2CO3 |
100 |
6 |
85 |
9 |
30 |
DMF |
K2CO3 |
50 |
6 |
55 |
10 |
5 |
DMF |
K2CO3 |
120 |
6 |
26 |
11 |
10 |
DMF |
K2CO3 |
120 |
6 |
61 |
12 |
20 |
DMF |
K2CO3 |
120 |
6 |
73 |
13 |
40 |
DMF |
K2CO3 |
120 |
6 |
96 |
14 |
30 |
DMF |
K2CO3 |
120 |
2 |
55 |
15 |
30 |
DMF |
K2CO3 |
120 |
4 |
75 |
16 |
30 |
DMF |
K2CO3 |
120 |
8 |
96 |
The results of the Heck cross-coupling reactions are shown in Scheme 3. The highly efficient substituted product E is readily obtained from various aryl iodides and Pd/CON-KEY1 6 as a catalyst under standard Heck cross-coupling reaction conditions. High efficiency and spatial selectivity (trans products 9a–f) are notable features of this reaction.
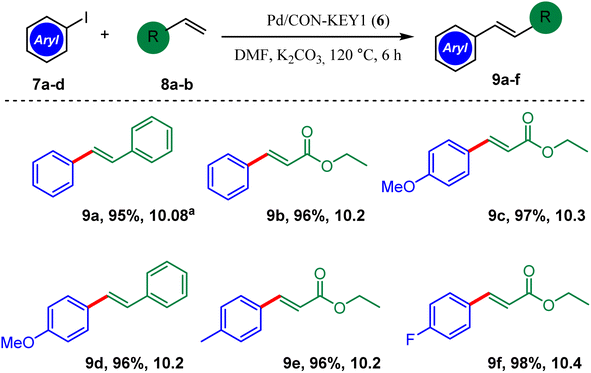 |
| Scheme 3 Heck cross-coupling reactions of aryl iodide with acrylate derivatives and styrene. aTON = yield/amount of catalyst (mol). | |
To optimize the parameters involved in the Suzuki–Miyaura coupling reaction, a model reaction was carried out with iodobenzene 7a and phenyl boronic acid 10a, and the results are shown in Table 4 (Table 4, entries 1–15).
Table 4 Optimization Screenings

|
Entry |
Catalyst 6 (mg) |
Solvent |
Base |
T (°C) |
Time (h) |
Yield (%) |
1 |
30 |
DMF |
K3PO4 |
120 |
6 |
95 |
2 |
30 |
Toluene |
K3PO4 |
120 |
6 |
60 |
3 |
30 |
DMSO |
K3PO4 |
120 |
6 |
60 |
4 |
30 |
NMP |
K2CO3 |
120 |
6 |
52 |
5 |
30 |
DMF |
Na2CO3 |
120 |
6 |
76 |
6 |
30 |
DMF |
KOH |
120 |
6 |
44 |
7 |
30 |
DMF |
NaOH |
120 |
6 |
41 |
8 |
30 |
DMF |
K3PO4 |
100 |
6 |
85 |
9 |
30 |
DMF |
K3PO4 |
50 |
6 |
53 |
10 |
10 |
DMF |
K3PO4 |
120 |
6 |
61 |
11 |
20 |
DMF |
K3PO4 |
120 |
6 |
65 |
12 |
40 |
DMF |
K3PO4 |
120 |
6 |
95 |
13 |
30 |
DMF |
K3PO4 |
120 |
2 |
56 |
14 |
30 |
DMF |
K3PO4 |
120 |
4 |
65 |
15 |
30 |
DMF |
K3PO4 |
120 |
8 |
95 |
Scheme 4 shows the catalytic role of Pd/CON-KEY1 6 in the production of biaryl compounds 11a–l under optimized reaction conditions. As expected, the aryl iodides afforded the desired biaryls with high reaction yields. For example, aryl iodide containing m-substituted NO2 was converted to the desired m-nitro biphenyl yielding 94%. C–C coupling reaction yield of p-iodoanisole was 95%. For aryl iodide bearing p-Me, the yield was 86%. More importantly, it was observed that excellent product yields were attained when aryl bromides were used as an aryl halide source. 98% and 95% yields were observed in the C–C reaction of p-bromobenzonitrile and p-bromonitrobenzene, respectively. The desired biphenyl with a 93% yield was reached for m-bromonitrobenzene. Pd/CON KEY1 successfully catalyzed the C–C reaction of p-bromotoluene with a yield of 88%.
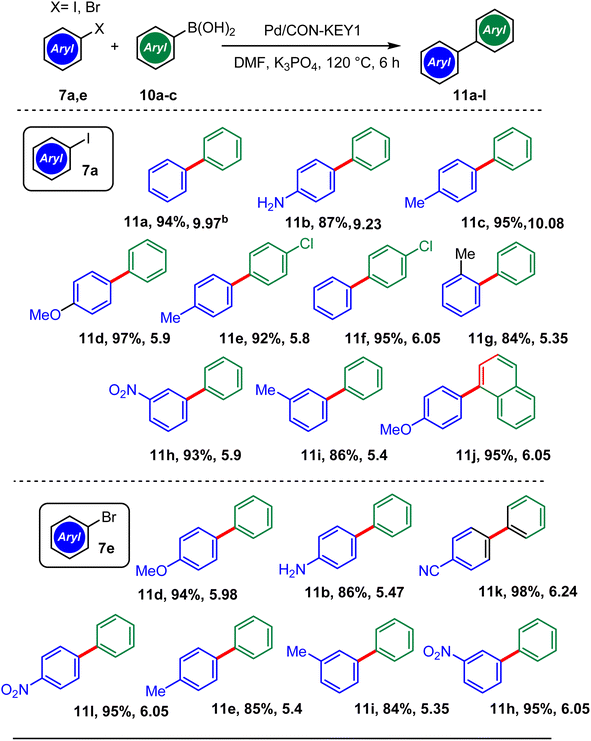 |
| Scheme 4 Pd/CON-KEY1 catalyzed Suzuki–Miyaura coupling reaction.a aReaction conditions: Ar-X 7 (0.5 mmol), ArB(OH)2 10 (0.6 mmol), K3PO4 (0.6 mmol) and Pd/CON-KEY1 6 catalyst (30 mg). bTON = yield/amount of catalyst (mol). | |
3.3. Comparison activity of Pd/CON-KEY1 with other catalysts
The advantages of the produced nanocatalyst are summarized in Tables 5 and 6, in which its properties are compared with various catalytic systems. Accordingly, the catalytic system presented in this study offers higher efficiency than other systems for this reaction regarding the reaction yield and time. The advantages of Pd/CON-KEY1 6 as the catalyst include having a higher yield, a less intensive process, and easier separability and recyclability several times (entry 10).
Table 5 Comparison of the activity of some catalysts with Pd/CON-KEY1 6 for the Suzuki–Miyaura reaction among phenyl iodide and benzene boronic acid
Entry |
Catalyst |
Conditions |
Time (h) |
Yield (%) |
Ref. |
1 |
Pd imino-Py-γ-Fe2O3 |
Et3N, DMF, 100 °C |
0.5 |
95 |
36 |
2 |
γ-Fe2O3-acetamidine-Pd |
Et3N, DMF, 100 °C |
0.5 |
96 |
37 |
3 |
Pd-Py-MCM-41 |
Na2CO3, PEG, 80 °C |
2 |
97 |
38 |
4 |
LDH-Pd(0) |
K2CO3, 1,4-dioxane-H2O, 80 °C |
10 |
96 |
39 |
5 |
MCM-41-S-Pd(0) |
K2CO3, DMF-H2O, 80 °C |
6 |
98 |
40 |
6 |
Pd-BIP-γ-Fe2O3@SiO2 |
Et3N, DMF, 100 °C |
1 |
98 |
41 |
7 |
Fe3O4-Bpy-Pd(OAc)2 |
K2CO3, toluene, 80 °C |
6 |
99 |
42 |
8 |
Fe3O4@SiO2@mSiO2-Pd(II) |
K2CO3, EtOH, 80 °C |
3 |
98 |
43 |
9 |
Pd(II)–NHC complex |
Cs2CO3, DMF, 100 °C |
24 |
99 |
44 |
10 |
Pd/CON-KEY1 6 |
K3PO4, DMF, 120 °C |
6 |
97 |
This work |
Table 6 Comparison of the activity of some catalysts with Pd/CON-KEY1 for the Heck cross-coupling between phenyl iodide and ethyl acrylate
Entry |
Catalyst |
Conditions |
Time (h) |
Yield (%) |
Ref. |
1 |
Fe3O4@SiO2/Schiff base/Pd(II) |
K2CO3, DMF, 110 °C |
0.75 |
97 |
45 |
2 |
Pd-NPs |
Et3N, IL, 100 °C |
3 |
87 |
46 |
3 |
Pd/Fe3O4 |
K2CO3, NMP, 130 °C |
5 |
99 |
47 |
4 |
Pd/6c complex |
K2CO3, DMAc, 120 °C |
2 |
97 |
48 |
5 |
Fe3O4/DAG/Pd |
Et3N, DMF, 110 °C |
0.5 |
96 |
49 |
6 |
NHC–Pd(II) |
Na2CO3, DMA, 160 °C |
18 |
99 |
50 |
7 |
Pd(OAc)2@MNP |
Et3N, DMF, 100 °C |
1 |
97 |
51 |
8 |
Pd-isatin Schiff base-γ-Fe2O3 |
Et3N, solvent-free, 100 °C |
0.5 |
95 |
52 |
9 |
SiO2@Fe3O4–Pd |
K2CO3, DMF, 100 °C |
8 |
97 |
53 |
10 |
Pd/CON-KEY1 6 |
K2CO3, DMF, 120 °C |
2–6 |
98 |
This work |
3.4. Reusability of Pd/CON-KEY1 6 nanocatalyst
Finally, it is worth mentioning that recycling and reusing the catalysts have become a prospect for the synthesis of longer-lasting catalysts due to their high manufacturing costs and environmental conditions. Once the reaction is completed, the Pd/CON-KEY1 6 catalyst can be easily separated from the reaction mixture via filtration and reused in subsequent reactions after being washed with water and ethanol and dried in a vacuum. Significantly, more than an average of 98% of catalysts can be recovered each time (Fig. 10).
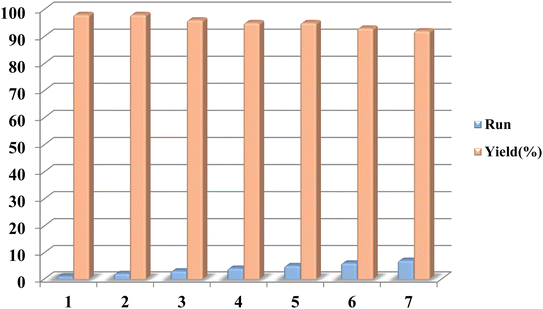 |
| Fig. 10 Recycling experiment of the Pd/CON-KEY1 6 for the reaction of phenyl iodide and ethyl acrylate. | |
This study's Pd/CON-KEY1 6 catalyst recovery indicates a high-efficiency sequential application. A survey of the FT-IR spectrum(Fig. 11) & X-ray diffraction (PXRD) (Fig. 12) for the Pd/CON-KEY1 6 catalyst after recovery of the synthesized nanocatalyst shows the stability of this catalyst.
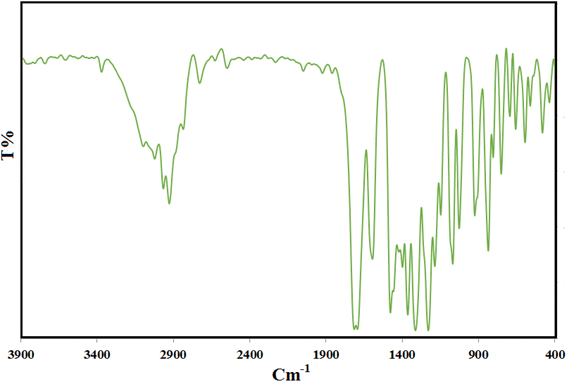 |
| Fig. 11 FT-IR spectrum for Pd/CON-KEY1 6 catalyst after recovery. | |
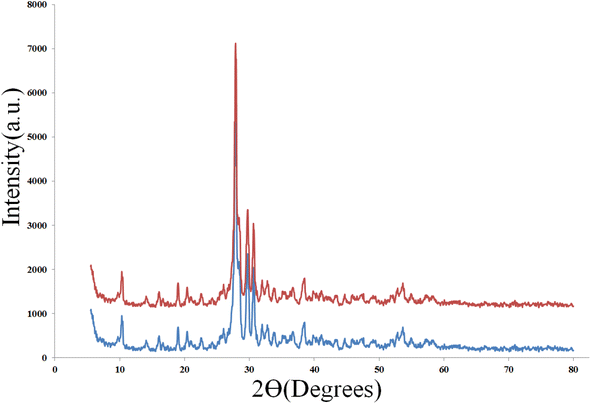 |
| Fig. 12 X-ray diffraction (PXRD) of (blue) fresh and (red) reused Pd/CON-KEY1 6 catalyst after recovery. | |
After theoretical and experimental studies on these modified nano polymers and proving their stability in reactions, it is necessary to use this class of compounds more than before.
4. Conclusion
Here we reported the facile synthesis of a novel amide-based CON through the reaction of CDI, [2,2′-bipyridine]-5,5′-diamine(BDA) and 1,3,5-tris(4-carboxyphenyl)benzene (TCB). Loading the obtained CON(CON-KEY1) with palladium chloride resulted in an efficient catalyst for Suzuki and Heck coupling reactions. The CON-Pd/CON-KEY1 catalyst was characterized by ICP-OES, TG-DTA, FT-IR, EDX, XRD, TEM, XPS, Element mapping and N2 isotherms. Theoretical studies proved the formation of the catalyst in an eclipsed-layered structure. This product was a heterogeneous active catalyst for Heck and Suzuki reactions. Other contributors are the cooperative effect between the amide sites and the large surface area and high porosity of CON. In addition, Pd/CON-KEY1 catalyst was found to be a multifunctional CON with robustness and stability under reaction conditions. Accordingly, it can be reused up to eight times without losing its catalytic activity and structural integrity. The other benefits of the proposed mesoporous catalyst include high yield, facile separation of the catalyst from the mixture, great TOF, and a clean reaction profile. This research portrays a bright future in using porous CONs and their functionalized analogs as multifunctional catalysts.
Conflicts of interest
The authors declare that they have no competing interests.
Acknowledgements
Any funding source had no role in the design of this study and will not have any role during its execution, analyses, interpretation of the data, or decision to submit results. The authors express appreciation to the Bu-Ali Sina University for supporting this investigation.
References
- H. Keypour, et al., Facile synthesis of a new covalent organic framework (COF-AYLIN) based on polyamide links and their application in CN coupling reaction, Inorg. Chim. Acta, 2023, 552, 121494 CrossRef CAS.
- J. Kouhdareh, et al., Pd (II)-immobilized on a novel covalent imine framework (COF-BASU1) as an efficient catalyst for asymmetric Suzuki coupling, J. Mol. Struct., 2023, 1273, 134286 CrossRef CAS.
- K. T. Tan, et al., Covalent organic frameworks, Nat. Rev. Methods Primers, 2023, 3(1), 1–19 CrossRef CAS.
- X. Feng, X. Ding and D. Jiang, Covalent organic frameworks, Chem. Soc. Rev., 2012, 41(18), 6010–6022 RSC.
- R. K. Sharma, et al., Recent development of covalent organic frameworks (COFs): synthesis and catalytic (organic-electro-photo) applications, Mater. Horiz., 2020, 7(2), 411–454 RSC.
- K. Geng, et al., Covalent organic frameworks: design, synthesis, and functions, Chem. Rev., 2020, 120(16), 8814–8933 CrossRef CAS PubMed.
- H. R. Abuzeid, A. F. EL-Mahdy and S.-W. Kuo, Covalent organic frameworks: Design principles, synthetic strategies, and diverse applications, Giant, 2021, 6, 100054 CrossRef CAS.
- W. Zhang, et al., Reconstructed covalent organic frameworks, Nature, 2022, 604(7904), 72–79 CrossRef CAS PubMed.
- D. Ma, et al., Covalent organic frameworks: promising materials as heterogeneous catalysts for CC bond formations, Catalysts, 2018, 8(9), 404 CrossRef.
- S.-Y. Ding, et al., Construction of covalent organic framework for catalysis: Pd/COF-LZU1 in Suzuki–Miyaura coupling reaction, J. Am. Chem. Soc., 2011, 133(49), 19816–19822 CrossRef CAS PubMed.
- J. Liu, N. Wang and L. Ma, Recent advances in covalent organic frameworks for catalysis, Chem. – Asian J., 2020, 15(3), 338–351 CrossRef CAS PubMed.
- V. Sharma, M. Nemiwal and D. Kumar, Catalytic applications of recent and improved covalent organic frameworks, Mini-Rev. Org. Chem., 2022, 19(7), 815–825 CrossRef CAS.
- J. Kouhdareh, et al., Immobilization of Ag and Pd over a novel amide based covalent organic framework (COF-BASU2) as a heterogeneous reusable catalyst to reduce nitroarenes, Inorg. Chim. Acta, 2023, 545, 121251 CrossRef CAS.
- Y. Zhi, et al., Recent progress in metal-free covalent organic frameworks as heterogeneous catalysts, Small, 2020, 16(24), 2001070 CrossRef CAS PubMed.
- R. Karimi-Nami, et al., Pd Immobilization Phenanthroline-2, 9-Dicarbaldehyde Modified Magnetic CuBDC MOF as a Reusable Heterogeneous Catalyst for Suzuki-Miyaura Cross-Coupling Reactions, Polycyclic Aromat. Compd., 2023, 1–16 Search PubMed.
- M. Aghayee, et al., Synthesis and characterization of a novel magnetic nano-palladium Schiff base complex: application in cross-coupling reactions, Appl. Organomet. Chem., 2016, 30(8), 612–618 CrossRef CAS.
- H. Keypour, et al., Synthesis of magnetically recyclable Fe3O4@[(EtO) 3Si–L1H]/Pd (II) nanocatalyst and application in Suzuki and Heck coupling reactions, Appl. Organomet. Chem., 2017, 31(2), e3558 CrossRef.
- M. Balali, et al., Palladium Supported on Schiff Base Functionalized Magnetite Nanoparticles as an Efficient Catalyst for Coupling Reactions, Inorg. Chem. Res., 2021, 5(1), 82–93 Search PubMed.
- H. K. Jamal Kouhdareh and S. Alavinia, Anchorage of pd into modified isoreticular metal–organic framework-3 as a heterogeneous catalyst for mizoroki–heck cross-coupling reactions, Acta Chem. Malays., 2022, 6(1), 35–42 Search PubMed.
- J. K. Hassan Keypour, S. Alavinia and M. Taher Rezaei, Synthesis of magnetic nano catalyst using dopamine functionalized magnetic nano particles and investigation of its properties in mizoroki–heck cross-coupling reactions, Acta Chem. Malays., 2023, 7(1), 16–22 Search PubMed.
- R. Karimi-Nami, et al., Pd Immobilization Phenanthroline-2, 9-Dicarbaldehyde Modified Magnetic CuBDC MOF as a Reusable Heterogeneous Catalyst for Suzuki-Miyaura Cross-Coupling Reactions, Polycyclic Aromat. Compd., 2023, 1–16 Search PubMed.
- B. Agrahari, et al., Synthesis, crystal structures, and application of two new pincer type palladium (II)-Schiff base complexes in CC cross-coupling reactions, Inorg. Chim. Acta, 2018, 471, 345–354 CrossRef CAS.
- S. A. Jasim, et al., Nanomagnetic Salamo-based-Pd (0) Complex: an efficient heterogeneous catalyst for Suzuki–Miyaura and Heck cross-coupling reactions in aqueous medium, J. Mol. Struct., 2022, 1261, 132930 CrossRef CAS.
- Y. Thopate, et al., Cascade Multicomponent Reaction Involving Unprecedented Gould Jacobs–Heck/Suzuki Coupling-Hydrolysis-Decarboxylation Reactions in One Pot: Rapid Synthesis of Hybrid Heterocyclic
Molecules, Asian J. Org. Chem., 2022, 11(8), e202200343 CrossRef CAS.
- R.-X. Liang and Y.-X. Jia, Aromatic π-components for enantioselective Heck reactions and Heck/anion-capture domino sequences, Acc. Chem. Res., 2022, 55(5), 734–745 CrossRef CAS PubMed.
- J. Kouhdareh, et al., Immobilization of Ag and Pd over a novel amide based covalent organic framework (COF-BASU2) as a heterogeneous reusable catalyst to reduce nitroarenes, Inorg. Chim. Acta, 2022, 121251 Search PubMed.
- Z. Mohammadkhani, et al., Synthesizing and post-synthetically modifying metal-organic frameworks (Co (BDC)-NH2) for carbonylative sonogashira coupling reaction, J. Organomet. Chem., 2023, 999, 122822 CrossRef CAS.
- H. Keypour, et al., Stabilization of Au-Nanoparticle-Decorated Postsynthesis-Modified Cu (BDC-NH2) MOF as a Reusable Heterogeneous Catalyst in an Intermolecular Hydroamination of Allenamides with Arylamines, J. Organomet. Chem., 2023, 122873 CrossRef CAS.
- H. Keypour, et al., An Efficient Method for Enhancement in the catalytic activity of Dual-Porous Co (BDC-NH2) MOF Au-Decorated Nanoparticle through Post-Synthetic Modification, J. Organomet. Chem., 2023, 122778 CrossRef CAS.
- H. Keypour, et al., Development of sustainable green catalysts for oxidation of alcohols via decorated palladium nanoparticles on magnetic carbon nanotube/MOF, J. Mol. Struct., 2023, 1294, 136444 CrossRef CAS.
- J. Kouhdareh, et al., Synthesis of a Au/Au NPs-PPy/l-CYs/ZIF-8 nanocomposite electrode for voltammetric determination of insulin in human blood, RSC Adv., 2023, 13(35), 24474–24486 RSC.
- H. Keypour, et al., Pd-Coordinated Salinidol-Modified Mixed MOF: An Excellent Active Center for Efficient Nitroarenes Reduction and Selective Oxidation of Alcohols, ACS Omega, 2023, 8(24), 22138–22149 CrossRef CAS PubMed.
- Z. Shariatinia and M. Abdollahi-Moghadam, DFT computations on surface physical adsorption of hydrocarbons produced in the Fischer-Tropsch synthesis on a CNT/Co nanocatalyst, J. Saudi Chem. Soc., 2018, 22(7), 786–808 CrossRef CAS.
- R. Hussain, et al., Density functional theory study of palladium cluster adsorption on a graphene support, RSC Adv., 2020, 10(35), 20595–20607 RSC.
- M. Abdollahi-Moghadam, et al., An experimental and theoretical study of a new sensitive and selective Al3+ Schiff base fluorescent chemosensor bearing a homopiperazine moiety, J. Mol. Struct., 2023, 1273, 134289 CrossRef CAS.
- Y. Leng, et al., Generally applicable and efficient oxidative Heck reaction of arylboronic acids with olefins catalyzed by cyclopalladated ferrocenylimine under base-and ligand-free conditions, Tetrahedron, 2010, 66(6), 1244–1248 CrossRef CAS.
- L. Wang, H. Li and P. Li, Task-specific ionic liquid as base, ligand and reaction medium for the palladium-catalyzed Heck reaction, Tetrahedron, 2009, 65(1), 364–368 CrossRef CAS.
- S. Sobhani, et al., Palladium-Schiff base complex immobilized covalently on magnetic nanoparticles as an efficient and recyclable catalyst for Heck and Suzuki cross-coupling reactions, Catal. Lett., 2016, 146(1), 255–268 CrossRef CAS.
- S. Sobhani, et al., Acetamidine–palladium complex immobilized on γ-Fe2O3 nanoparticles: a novel magnetically separable catalyst for Heck and Suzuki coupling reactions, RSC Adv., 2014, 4(83), 44166–44174 RSC.
- M. Nikoorazm, A. Ghorbani-Choghamarani and A. Jabbari, A facile preparation of palladium Schiff base complex supported into MCM-41 mesoporous and its catalytic application in Suzuki and Heck reactions, J. Porous Mater., 2016, 23(4), 967–975 CrossRef CAS.
- S. Singha, M. Sahoo and K. Parida, Highly active Pd nanoparticles dispersed on amine functionalized layered double hydroxide for Suzuki coupling reaction, Dalton Trans., 2011, 40(27), 7130–7132 RSC.
- L. Zhou, et al., Recent advances in non-metal modification of graphitic carbon nitride for photocatalysis: a historic review, Catal. Sci. Technol., 2016, 6(19), 7002–7023 RSC.
- S. Sobhani, Z. Zeraatkar and F. Zarifi, Pd complex of an NNN pincer ligand supported on γ-Fe2O3@ SiO2 magnetic nanoparticles: a new catalyst for Heck, Suzuki and Sonogashira coupling reactions, New J. Chem., 2015, 39(9), 7076–7085 RSC.
- Y.-Q. Zhang, X.-W. Wei and R. Yu, Fe3O4 nanoparticles-supported palladium-bipyridine complex: effective catalyst for Suzuki coupling reaction, Catal. Lett., 2010, 135(3), 256–262 CrossRef CAS.
- M. Nasrollahzadeh, S. M. Sajadi and M. Maham, Green synthesis of palladium nanoparticles using Hippophae rhamnoides Linn leaf extract and their catalytic activity for the Suzuki–Miyaura coupling in water, J. Mol. Catal. A: Chem., 2015, 396, 297–303 CrossRef CAS.
- Q. Xu, et al., A novel cis-chelated Pd
(II)–NHC complex for catalyzing Suzuki and Heck-type cross-coupling reactions, Tetrahedron, 2005, 61(47), 11225–11229 CrossRef CAS.
- M. Esmaeilpour and J. Javidi, Magnetically-recoverable Schiff Base Complex of Pd (II) Immobilized on Fe3O4@ SiO2 Nanoparticles: An Efficient Catalyst for Mizoroki–Heck and Suzuki–Miyaura Coupling Reactions, J. Chin. Chem. Soc., 2015, 62(7), 614–626 CrossRef CAS.
- D. S. Gaikwad, Y. Park and D. M. Pore, A novel hydrophobic fluorous ionic liquid for ligand-free Mizoroki–Heck reaction, Tetrahedron Lett., 2012, 53(24), 3077–3081 CrossRef CAS.
- X. Liu, et al., 2D phosphorene nanosheets, quantum dots, nanoribbons: synthesis and biomedical applications, Biomater. Sci., 2021, 9, 2768–2803 RSC.
- A.-E. Wang, et al., Triaryl phosphine-functionalized N-heterocyclic carbene ligands for Heck reaction, Tetrahedron, 2005, 61(1), 259–266 CrossRef CAS.
- H. Veisi, A. Sedrpoushan and S. Hemmati, Palladium supported on diaminoglyoxime-functionalized Fe3O4 nanoparticles as a magnetically separable nanocatalyst in Heck coupling reaction, Appl. Organomet. Chem., 2015, 29(12), 825–828 CrossRef CAS.
- T. Chen, J. Gao and M. Shi, A novel tridentate NHC–Pd (II) complex and its application in the Suzuki and Heck-type cross-coupling reactions, Tetrahedron, 2006, 62(26), 6289–6294 CrossRef CAS.
- Q. Zhang, et al., Covalently anchored tertiary amine functionalized ionic liquid on silica coated nano-Fe3O4 as a novel, efficient and magnetically recoverable catalyst for the unsymmetrical Hantzsch reaction and Knoevenagel condensation, RSC Adv., 2017, 7(85), 53861–53870 RSC.
|
This journal is © The Royal Society of Chemistry 2023 |
Click here to see how this site uses Cookies. View our privacy policy here.