DOI:
10.1039/D3RA05750J
(Paper)
RSC Adv., 2023,
13, 32457-32472
AgBr nanoparticle surface modified SnO2 enhanced visible light catalytic performance: characterization, mechanism and kinetics study
Received
23rd August 2023
, Accepted 23rd October 2023
First published on 3rd November 2023
Abstract
In this study, a simple hydrothermal procedure and in situ precipitation method were used to prepare SnO2–AgBr composites, where the molar ratios of SnO2 and AgBr were 1
:
1, 1
:
2 and 2
:
1. Characterization results showed that the composites had excellent dispersion, crystallinity, and purity. A photocatalytic degradation experiment and first-order kinetic model indicate that SnO2–AgBr (1
:
1) had the best photocatalytic performance, and the degradation rates of 30 mg L−1 simulated MO and MG wastewater reached 96.71% and 93.36%, respectively, in 150 min, which were 3.5 times those of SnO2. The degradation rate of MO and MG increases with the dosage. Humic acid inhibited the degradation of MG, while a low concentration of humic acid promoted the degradation of MO, and the composite has good stability with pH. A free radical trapping experiment shows that ·OH and ·O2− were the main active substances, and h+ was the secondary one. According to the results of the characterization and photocatalysis experiments, a Z-scheme mechanism for the SnO2–AgBr composite was proposed, and the degradation pathway of target pollutants was speculated upon. This study has conceived novel methods for the development of a mature Z-scheme mechanism and in doing so has provided new approaches for the development of photocatalysis for water pollution control.
1. Introduction
With the rapid development of society and the economy, the world produces about 800
000 tons of synthetic dyes every year, of which about 10–15% are transferred to the natural environment by human activities1,2 This pollutant will not only cause serious pollution to the water environment, affecting the growth metabolism of aquatic creatures, but is also a potential threat to human health.3–6 Numerous studies have focused on degradation methods for synthetic dyes, such as adsorption,7 microbial degradation,8 Fenton reaction,9 photocatalysis,10 and electrochemistry.11 Photocatalytic technology as a green sustainable method that uses solar energy to solve the problem of environmental pollution has received widespread attention due to its low cost and environmentally friendly processing. The strong oxygen reducing active substance produced by the photocatalyst in sunlight can degrade pollutants into intermediate products that are prone to biodegradation in a short period of time, or directly mineralized into H2O and CO2.12–15
Tin dioxide (SnO2) is a typical semiconductor material, which has the advantages of non-toxicity, excellent photoelectric performance and low cost, but because of its wide band gap (3.6 eV), it has almost no response to visible light, which greatly limits its application as a photocatalytic material.16,17 Elemental mixing and semiconductor compounding are the two most commonly used modification methods for SnO2. Elemental mixing is mainly used in the field of battery research, and different types of elements can achieve different modification effects: Sb18 and Yb19 can improve the conductivity, Ag20 can improve the arc erosion performance, and Er21 and Pb22 can improve the optical emission performance. A semiconductor composite is commonly used in the field of photocatalysis, such as TiO2,23 Bi12O15Cl6,24 g-C3N4 (ref. 25) and other heterojunctions formed with SnO2 which reduce the photogenerated hole–electron recombination rate, and broaden the photocatalytic performance in the visible light range. Metal sulfides are important narrow-band-gap semiconductor materials which typically have excellent visible light absorption ability.26 One of them, silver bromide (AgBr), is a good candidate for SnO2 photosensitization due to its fast charge exchange properties, narrow band gap and high stability as a non-toxic semiconductor.
This study uses a hydrothermal procedure and an in situ precipitation method to prepare an SnO2–AgBr composite photocatalyst that responds to visible light. It is known that the composite molar ratio between SnO2 and AgBr is one of the main factors affecting its optical catalytic activity, so using methyl orange (MO) and malachite green (MG) as target pollutants, the effect of a high molar ratio on photocatalytic activity was investigated. Through the influence of composite dosage, pH and humic acid concentration on degradation efficiency, the stability of the photocatalyst to a water environment was demonstrated, and a Z-scheme mechanism of the SnO2–AgBr composite was proposed, and the degradation pathways of MO and MG were speculated upon.
2. Experiment
2.1 Preparation of SnO2
All chemicals were of analytical purity and used without further purification. A certain amount of SnCl4·5H2O was dissolved in 40 mL of deionized water and magnetically stirred for 60 min. NaOH was added to adjust the pH to 10 and then stirring continued for 30 min. The final product was obtained by hydrothermal reaction at 180 °C for 10 h. The SnO2 crystals were washed alternately with deionized water and anhydrous ethanol 3 times, and baked at 90 °C until dry.
2.2 Preparation of SnO2–AgBr
0.46 g of SnO2 was dissolved in 40 mL of deionized water; then 30 mL of 0.1 mol L−1 AgNO3 solution was added, and stirred for 30 min. 0.36 g of KBr was dissolved in 40 mL of deionized water, and then the KBr solution was slowly added to the above mixture and stirred away from light for 3 h. After the sediment was filtered and washed with deionized water and heated at 130 °C for 20 h, an SnO2–AgBr composite with a molar ratio of 1
:
1 could be obtained, which is denoted SnO2–AgBr (1
:
1). The above steps were repeated to prepare composites with molar ratios of SnO2 and AgBr of 1
:
2 and 2
:
1, denoted SnO2–AgBr (1
:
2) and SnO2–AgBr (2
:
1), respectively.
2.3 Characterization and instrumental parameters
In this experiment, the crystallinity of the sample was characterized with a D8 ADVANCE X-ray diffractometer (XRD), Kα radiation (λ = 1.5406 A) Cu target; the diffraction angle ranged from 10° to 80°. An SU8220 cold field emission scanning electron microscope (SEM) was used to characterize the structure and composition of the samples. The sample crystal was analyzed with a Talos F200S transmission electron microscope (TEM), and the chemical bond of the sample was analyzed by ESCALAB 250 X-ray photoelectron spectroscopy (XPS), where the binding energy in the spectrum was calibrated by C1s (284.62 eV). A 1290II-6460 liquid mass coupling instrument (HPLC-MS) was used to analyze the composition of the samples, in which the current was 10 μA and the voltage was 15 kV. An F-7100 Spectrometer (PL) tested the photoluminescence spectra. The light absorption properties of the materials were analyzed with a UV-3600 type UV-visible spectrophotometer. The electrochemical impedance spectroscopy (EIS) was measured at Voc one-sun illumination with 0.1 M Na2SO4 electrolyte between a Pt-coated film counter electrode and sample film coated on fluorine-doped tin oxide (FTO) as a working electrode, held by two heavy-duty clips.
2.4 Photocatalytic experiments
A 500 W tungsten halogen lamp (λ > 420 nm) was used as the visible light source, and 100 mL (30 mg L−1) of the MO/MG solution were added into the reactor. The temperature of the reaction solutions was kept constant by the actions of a magnetic blender and a cooling system. The SnO2–AgBr composites were weighed (0.1 g) and evenly dispersed in the solution. The light source was turned off for the first 30 min so that the catalyst and the target pollutants could achieve adsorption equilibrium. A DR6000 ultraviolet spectrophotometer was used to test the absorbance of MO and MG at 464 nm and 618 nm, respectively, and the concentrations at different times were calculated according to the standard curves of MO and MG, to calculate their degradation rates.
2.5 Cycling experiments
The stability of the catalyst was tested by cycling experiments. The same SnO2–AgBr (1
:
1) compounds were used to degrade MO/MG for four runs. After the degradation reaction the catalyst was collected, washed alternately with anhydrous ethanol and pure water four times, and then fully dried in a constant temperature drying oven at 90 °C before use.
3. Results and discussion
3.1 Material characterization
3.1.1 XRD analysis. The XRD of SnO2–AgBr composites with different molar ratios are shown in Fig. 1, in which the crystallinity and purity of the composites can be seen. The main diffraction peaks at 26.58°, 33.88°, 51.75° correspond to the tetragonal SnO2 (110), (101), (211) crystal faces (PDF#41-1445), and the diffraction peaks at 30.96°, 44.35° correspond to the cubic phase AgBr (200), (220) crystal face (PDF#06-0438).27,28 There are no other peaks, indicating that SnO2 and AgBr have high purity. The diffraction peaks position of SnO2 with different molar ratios did not change, indicating that AgBr did not change the crystal shape of SnO2 through surface loading. The diffraction peak intensity of AgBr increases with its loading capacity, but excessive AgBr led to an increase in the photogenerated hole–electron pair recombination rate and a decrease in photocatalytic degradation activity.29 The XRD results showed that SnO2 and AgBr were successfully prepared.
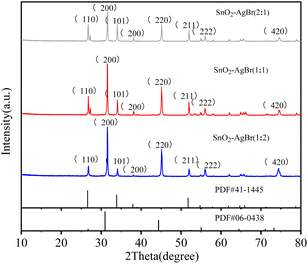 |
| Fig. 1 XRD of SnO2–AgBr composites with different mole ratios. | |
3.1.2 SEM and TEM analysis. The morphology and structure of the sample can be observed through SEM (Fig. 2a and b), showing that SnO2 consists mainly of a porous structure composed of irregular ellipsoids with different particle sizes, and the uneven gully structure on the surface provides support for the load of AgBr. As can be seen from Fig. 2c–e, there is local agglomeration of SnO2–AgBr (1
:
2) due to excessive AgBr, but no significant agglomeration of SnO2–AgBr (1
:
1) or SnO2–AgBr (2
:
1) can be observed due to the small size, small load and high dispersion of AgBr.
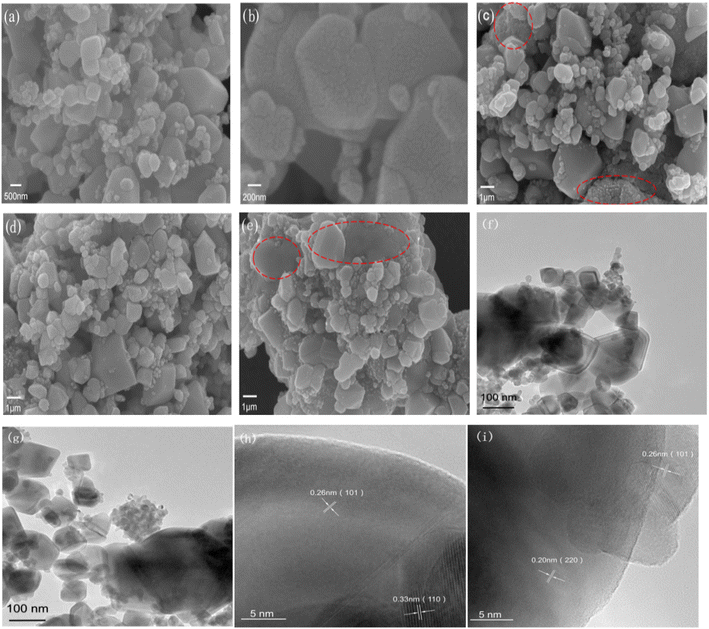 |
| Fig. 2 SEM and TEM of SnO2 and SnO2–AgBr: SEM of (a and b) AgBr, (c) SnO2–AgBr (1 : 2), (d) SnO2–AgBr (1 : 1), and (e) SnO2–AgBr (2 : 1); (f–i) TEM of SnO2–AgBr (1 : 1). | |
SnO2–AgBr (1
:
1) TEM (Fig. 2f–i) proved that SnO2 and AgBr were successfully combined, but there was a small amount of agglomeration in AgBr (Fig. 3f and g), which was an objective result of the experimental conditions. The lattice spacings of 0.26 nm and 0.33 nm correspond to the (101) and (110) crystal faces of SnO2, respectively (Fig. 2h); the lattice spacings of 0.26 nm and 0.20 nm correspond to the crystal faces of SnO2 (101) and AgBr (220), respectively (Fig. 2i).30–32
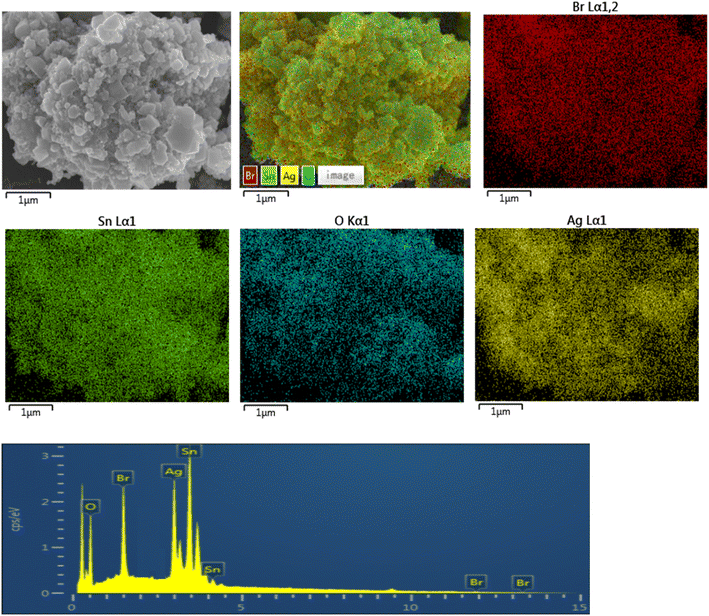 |
| Fig. 3 EDS of the SnO2–AgBr (1 : 1) composite. | |
3.1.3 EDS analysis. In order to understand the chemical composition and elemental distribution of the composite, EDS of SnO2–AgBr (1
:
1) was performed (Fig. 3). The results showed that the composite was composed of Sn, O, Ag and Br, and no other elements were detected. AgBr particles are evenly distributed on the surface of SnO2, and there is no large-scale agglomeration. The theoretical percentage of Ag and Br atoms is relatively low, which may be caused by the existence of a small amount of AgBr agglomeration during the preparation process.
3.1.4 XPS analysis. In order to investigate the elemental composition and valence state of the SnO2–AgBr composite, as well as the binding state of AgBr and SnO2 crystals, XPS of SnO2 and SnO2–AgBr (1
:
1) was performed; all spectra were based on C1s (284.4 eV). Fig. 4a shows the full spectra of SnO2 and SnO2–AgBr (1
:
1), where one can clearly see the binding energies of Sn 3d, O 1s, Ag 3d and Br 3d. The binding energies at 488.1 eV and 496.5 eV correspond to Sn 3d5/2 and Sn 3d3/2, respectively, and the pyrolysis energy difference is 8.4 eV, which proves that Sn is Sn4+ in the composite.33,34 After loading AgBr, the binding energies of Sn 3d are slightly shifted compared with SnO2, which may be because the electron cloud density of Sn is changed when AgBr and SnO2 combine with each other, which affects the electronic structure of SnO2.35 The binding energy of O1s at 529.6 eV corresponds to the lattice oxygen of Sn–O, and that at 530.9 eV corresponds to water species adsorbed on the surface of the as-prepared sample. These two reactive oxygen species will promote the formation of ·OH and ·O2− and facilitate the oxidative decomposition of organic matter.36,37 After loading AgBr, the binding energies of O 1s are shifted in the high-energy direction relative to SnO2, indicating that the composite has more oxygen vacancies, which can improve the separation of electron–hole pairs and increase the photocatalytic efficiency.38 Binding energies at 367.8 eV and 373.9 eV correspond to Ag3d5/2 and Ag3d3/2, respectively.39,40 In a summary of previous reports,41,42 binding energies at 367.3 eV and 374.2 eV are attributed to Ag0; those at 367.8 eV and 373.8 eV are attributed to Ag+. Therefore, the valence state of Ag in the composite is Ag+. The binding energies at 67.8 eV and 68.8 eV correspond to Br 3d5/2 and Br 3d3/2, respectively, indicating that the valence state of Br in the composite is Br−.43 In summary, SnO2 and AgBr were successfully bonded by chemical bonds.
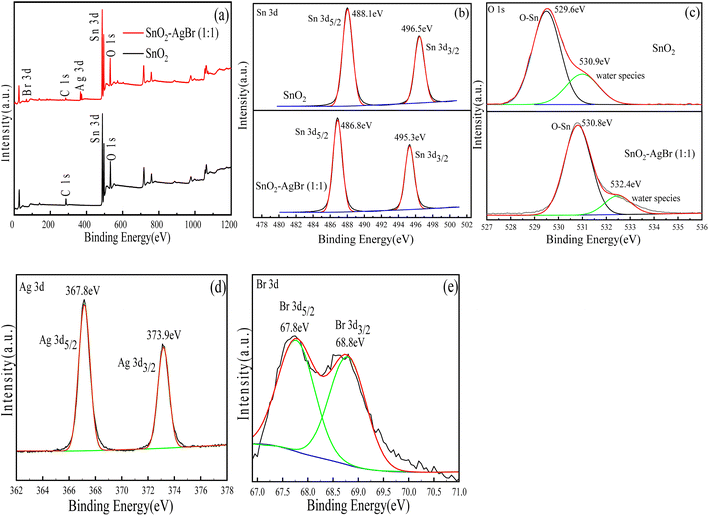 |
| Fig. 4 XPS of SnO2 and SnO2–AgBr (1 : 1): (a) full survey spectra, (b) Sn 3d, (c) O 1s, (d) Ag 3d, and (e) Br 3d. | |
3.1.5 UV-vis and PL analysis. The degree of response of the composite to light and the recombination efficiency of photogenerated electron–hole pairs are two main factors with which to measure the efficiency of photocatalytic materials. The light absorption range of the composite is reflected by UV-vis, and the photogenerated hole–electron recombination rate by PL emission spectroscopy. The absorption edge of SnO2 is around 340 nm, and it hardly responds to visible light. The absorption curve of AgBr does not decrease significantly in the range of 200–800 nm, and it still maintains a high absorption intensity (Fig. 5a). Compared with SnO2–AgBr with different molar ratios, the absorption curve of SnO2 is obviously redshifted towards the visible region, indicating that the load of AgBr effectively improves the ability of SnO2 to respond to visible light.44 The absorption intensity of the composite for visible light increased first and then decreased with AgBr load, and SnO2–AgBr (1
:
1) showed the best light absorption intensity and range. The results indicate that visible light can be used more effectively and more photogenerated electron–hole pairs can be generated, which are beneficial to the enhancement of photocatalytic activity.45,46 The band-gap width is calculated (Fig. 5b according to the Kubelka–Munk function). The band-gap widths of SnO2, AgBr, SnO2–AgBr (1
:
2), SnO2–AgBr (1
:
1) and SnO2–AgBr (2
:
1) are 3.51 eV, 2.6 eV, 2.75 eV, 2.62 eV and 2.80 eV, respectively.
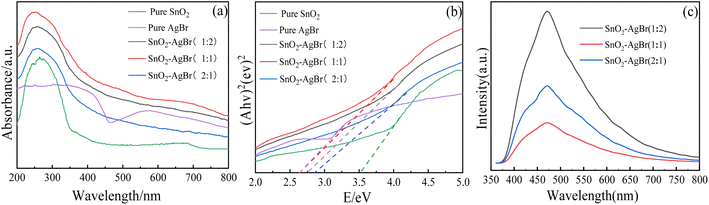 |
| Fig. 5 (a) UV-vis and (b) band-gap width of SnO2 and SnO2–AgBr composite; (c) PL of SnO2–AgBr composite. | |
PL results show that SnO2–AgBr with different molar ratios have diffraction peaks at 460 nm (Fig. 5c), but the SnO2–AgBr (1
:
1) composite has the lowest diffraction peak intensity, indicating the lowest electron–hole recombination efficiency and the longest photogenerated carrier lifetime.47,48 In summary, SnO2–AgBr (1
:
1) has the best photocatalytic performance compared with other molar ratio composites.
3.1.6 Zeta potential test. In order to investigate the charging property of the composite surface and its dispersion in water, a particle size analyzer was used to detect the zeta potential of the SnO2–AgBr composite, and the results are shown in Fig. 6a. The zero points of SnO2–AgBr (1
:
2), SnO2–AgBr (1
:
1) and SnO2–AgBr (2
:
1) are 4.61, 5.22 and 6.18, respectively. Under neutral conditions, the surface of SnO2–AgBr is electronegative, which is theoretically more conducive to attracting cationic MB, and has an electrostatic repulsive effect on anionic MO.49,50
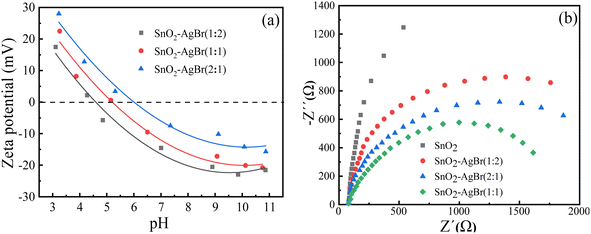 |
| Fig. 6 Zeta potential (a) and electrochemical impedance curve (b) of the SnO2–AgBr composite. | |
Electrochemical impedance spectroscopy (EIS) analysis was carried out to determine the electron transport properties of SnO2–AgBr that eventually influenced the performance of SnO2–AgBr. It can be seen from Fig. 6b that the impedance radius of SnO2 is the largest and that of SnO2–AgBr (1
:
1) is the smallest, indicating that SnO2–AgBr (1
:
1) has the smallest charge transfer resistance and the highest separation efficiency, which is consistent with the PL result.
3.2 Photocatalytic degradation experiment
3.2.1 Degradation analysis of target pollutants. As shown in Fig. 7a and b, the dark adsorption of the composite with different molar ratios for MO and MG is about 10%, which contradicts the conclusion of the zeta potential analysis. Therefore, it is speculated that electrostatic effect does not play a major role in the adsorption process. In the photoreaction phase, SnO2 had little degradation effect on MO or MG, which was consistent with the UV-vis analysis. The photocatalytic degradation rate of SnO2–AgBr was significantly increased because of the formed heterojunction. SnO2, as a photogenerated electron acceptor, could inhibit the photogenerated electron–hole recombination rate and improve the contact between active substances and pollutants.51,52 SnO2–AgBr (1
:
1) had the best degradation effect on MO and MG, and the 150 min treatment rates were 96.74% and 93.36%, respectively. A dynamic analysis of degradation rate (Fig. 7c and d) showed that the k of MO and MG were 2.56 × 10−2 min−1 and 2.01 × 10−2 min−1, respectively, which were 1.83 and 1.77 times those of SnO2–AgBr (2
:
1), 1.53 and 1.22 times those of SnO2–AgBr (1
:
2). The results indicated that SnO2–AgBr (1
:
1) had the fastest degradation rate for the two pollutants.53 The photocatalytic degradation rate of SnO2–AgBr (1
:
2) decreased because the active site on the surface of the composite was shielded due to excessive AgBr attachment to the surface of SnO2. At the same time, the light penetration was weakened and the absorption of visible light was affected by the composite.54–56 UV-vis absorption spectra of MO and MG (Fig. 7e and f) reflect the photocatalytic degradation process. The intensity of the absorption peaks at 464 nm and 618 nm gradually decreased and eventually disappeared with reaction time, indicating that the pollutants were degraded.
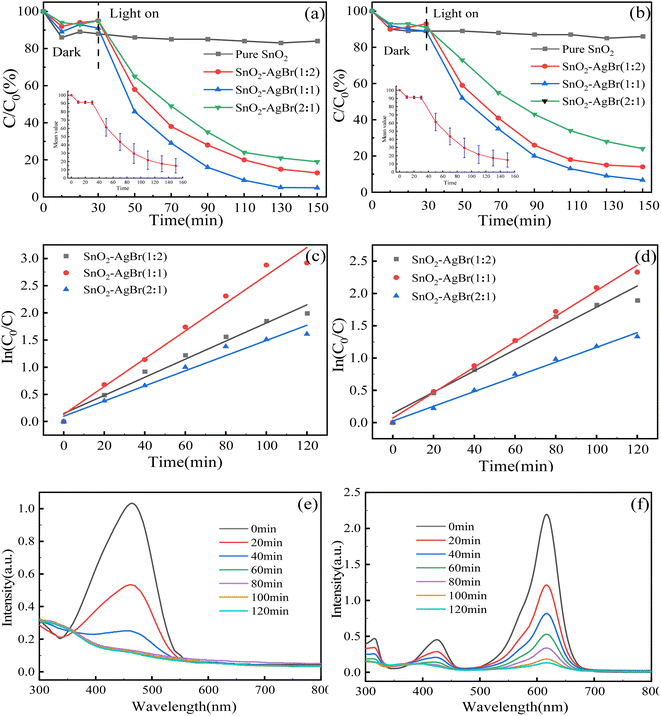 |
| Fig. 7 Photocatalytic degradation by the composite with different molar ratios for (a) MO and (b) MG; first-order kinetics of (c) MO and (d) MG; UV-vis absorption spectra of (e) MO and (f) MG. | |
3.2.2 The influence of composite dosage. In the treatment of wastewater, the catalyst is often one of the main costs in the advanced stage of water treatment. Under the condition of pH = 7, 100 mL MO and MG solution with a concentration of 30 mg L−1 were added with 0.05 g, 0.08 g, 0.10 g, 0.12 g and 0.15 g SnO2–AgBr (1
:
1) composite. The relationship between the dosage of the composite and the treatment efficiency was investigated within 150 min. As shown in Fig. 8, when the dosage increased from 0.5 g L−1 to 1.5 g L−1, the degradation rates of MO and MG increased from 54.42% and 59.85% to 97.76% and 96.32%, and the k increased by 4.58 and 3.65 times, respectively. In this experiment, there was no phenomenon where photocatalytic activity decreased due to excessive composite addition, which affected the transparency of the solution.57,58
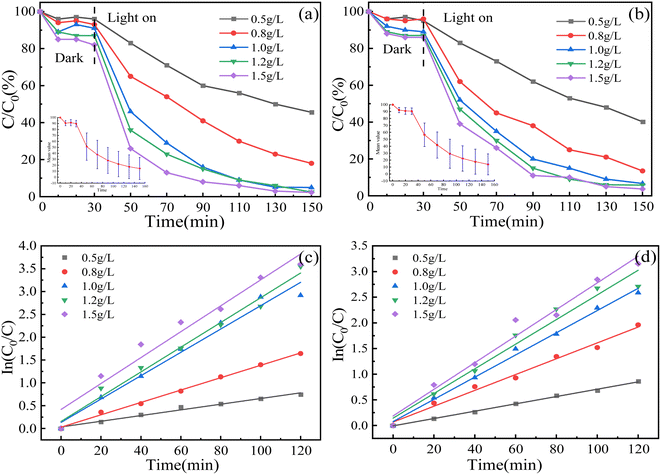 |
| Fig. 8 Effect of composite dosage on degradation rate of (a) MO and (b) MG; first-order kinetics of (c) MO and (d) MG. | |
3.2.3 Influence of humic acid concentration. Humic acid (HA) is produced by the decomposition of natural organic compounds and exists in all sewage, so it has a great influence on the form of existence and migration performance for some pollutants in water.59,60 In order to investigate the effect of HA concentration on catalyst degradation efficiency, HA concentrations were set at 0 mg L−1 (blank control), 5 mg L−1, 10 mg L−1 and 20 mg L−1. The experimental results are shown in Fig. 9. When the concentration of HA increased from 0 mg L−1 to 5 mg L−1, it promoted the degradation rate of MO, and inhibited it as the concentration continued to increase. However, it has consistently shown inhibitory effects on MG. The reason is that HA can be used as a photosensitizer to promote the production of active substances, thereby improving the degradation rate of MO and MG. However, it also forms a competitive relationship with MO and MG for active substances, or becomes a quenching agent, reducing the content of active substances.61–63 In this experiment, the two target pollutants show different experimental results, which is the competitive effect of HA.
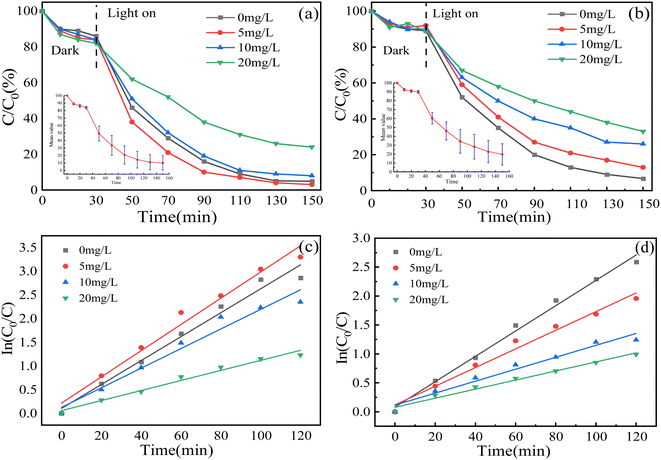 |
| Fig. 9 Effect of humic acid on degradation rate for (a) MO and (b) MG; first-order kinetics of (c) MO and (d) MG. | |
3.2.4 Influence of pH. pH is a very important parameter in water treatment, which can affect the efficiency of water treatment by changing the surface adsorption capacity of a catalyst or the hydrolyzed form of pollutants.64,65 The relationship between pH and degradation efficiency was investigated when the pH was 3, 5, 7, 9 and 11, and the dosage of the composite was 0.1 g. The results are shown in Fig. 10: with an increase in pH, the photocatalytic degradation rate of MO and MG has little effect, but the k of MO decreases continuously. The reason is that under neutral and alkaline conditions, MO is an azo structure containing N
N double bonds with a bond energy of 456 kJ mol−1, while under acidic conditions, the N
N double bonds are transformed into N–N single bond quinone structures with a bond energy of 159 kJ mol−1. Therefore, the reduction in bond energy under acidic conditions leads more easily to the decomposition of MO.66,67
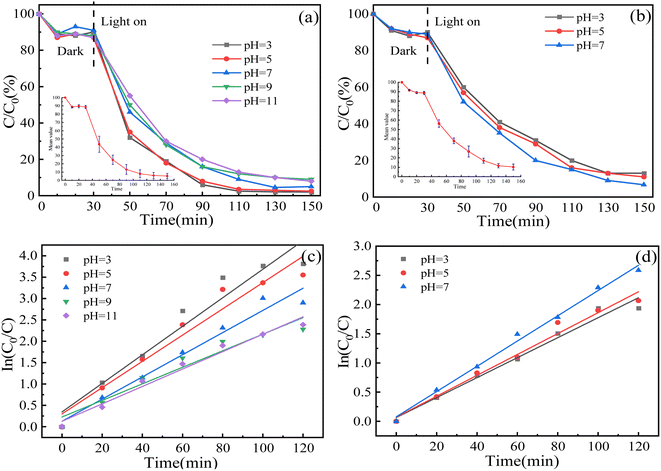 |
| Fig. 10 Effect of pH on degradation rate for (a) MO and (b) MG; first-order kinetics of (c) MO and (d) MG. | |
It was found that under alkaline conditions, MG was converted to colorless tertiary alcohol, resulting in the solution rapidly fading from blue-green to colorless.68,69 Due to the limitations of the experimental conditions, absorbance cannot be tested, so only acid and neutral conditions were investigated. The degradation effect of MG under acidic conditions is slightly decreased compared with that under neutral conditions, because H+ consumes the active substance ·OH or occupies the active site under acidic conditions.70 At the same time, with a change in pH, the adsorption properties of the composite for MG and MO showed almost no change, which proved that the adsorption process was not dominated by electrostatic action, but by the hydraulic action of surface complexation or magnetic agitation.
3.3 Repeated experiments
The stability of the SnO2–AgBr (1
:
1) composite is related to its practicability and is also an important factor to measure its photocatalytic performance.71,72 After four cycles of experiment, the degradation rates of MO and MG decreased to 66.42% and 70.86%, respectively (Fig. 11a and b). The samples collected after four cycles were tested by XRD, and the characterization results are shown in Fig. 11c and d. The position and intensity of the diffraction peak of SnO2–AgBr (1
:
1) did not change significantly, but the new diffraction peak at 38.25° corresponds to Ag0.73 AgBr is corroded by light and some Ag+ is restored to Ag0, which covers part of the active site. This is the main reason for the continuous decline in photocatalytic activity with repeated experiments.74,75
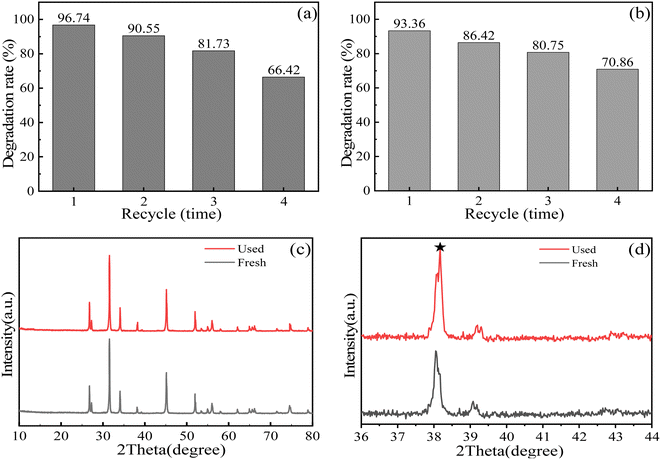 |
| Fig. 11 Composite repeated experiments for (a) MO and (b) MG; (c and d) XRD of composite after repeated experiments. | |
3.4 Free radical trapping experiment
The main active substances were determined by a free radical trapping experiment, and the degradation mechanism of SnO2–AgBr (1
:
1) was further studied. Isopropyl alcohol (IPA), p-benzoquinone (BQ) and ammonium oxalate (AO) were used as inhibitors of ·OH, ·O2− and h+, respectively.76,77 The dosage of BQ was 1 mmol L−1; those of IPA and AO were 10 mmol L−1. The results are shown in Fig. 12. The three inhibitors of active species all reduced the degradation efficiency to varying degrees, indicating that ·O2−, ·OH and h+ were involved in photocatalytic degradation. However, after the addition of BQ and IPA, the degradation rate seriously decreased, indicating that ·O2− and ·OH were the main active substances, and h+ played a secondary role. Moreover, these results were further confirmed by electron paramagnetic resonance (EPR) tests with DMPO as the spintrapping reagent. As displayed in Fig. 12c and d, signals of ·OH and ·O2− were observed in the SnO2–AgBr (1
:
1) system and the intensities of the signals gradually became intense with prolonged light illumination time.
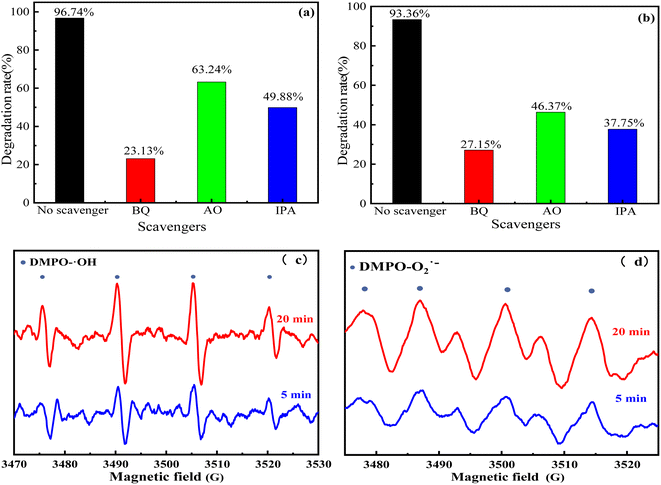 |
| Fig. 12 SnO2–AgBr (1 : 1) composite trapping experiments for (a) MO and (b) MG; EPR spectra of the SnO2–AgBr (1 : 1) composite: (c) DMPO–·OH, (d) DMPO–·O2−. | |
3.5 Prediction of degradation mechanism
According to the results of the characterization, the SnO2–AgBr composite reaction mechanism was deduced from the Mulliken empirical formula (eqn (1) and (2)):where EVB and ECB are the potentials at the top of the valence band and the bottom of the conduction band relative to the standard (H2) electrode potential, respectively; X is the electronegativity of the semiconductor; Ee is the energy of the free electron on the scale of the hydrogen atom (about 4.5 eV); Eg is the band-gap width of the semiconductor.
According to UV-vis, the band gaps of SnO2 and AgBr are 3.51 eV and 2.6 eV, respectively, and the electronegativities are 6.10 eV and 3.90 eV, respectively. The EVB and ECB of SnO2 are 3.35 eV and −0.16 eV, and those of AgBr are 2.6 eV and 0.0 V.78,79 SnO2 has no response to visible light due to its band-gap width of 3.51 eV, and therefore it cannot produce photogenerated electron–hole pairs. Since the ECB (0.00 eV) of AgBr is higher than that of SnO2 (−0.16 eV), photogenerated electrons cannot migrate from AgBr to SnO2 (Fig. 13a). Therefore, if the photoinduced electrons and holes migrate by the traditional type-II charge transfer mechanism, the experimental phenomena cannot be explained.
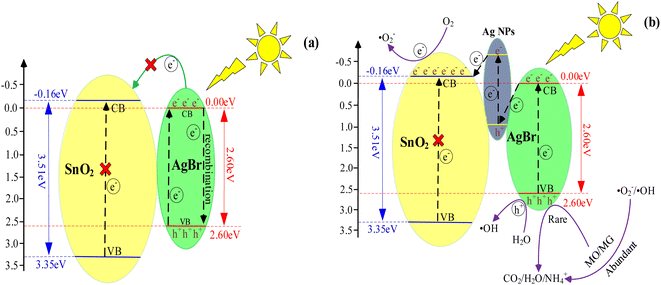 |
| Fig. 13 (a) SnO2–AgBr (1 : 1) traditional heterojunctions mechanism; (b) Z-scheme mechanism. | |
Therefore, a Z-scheme mechanism using Ag as the electronic medium is proposed (Fig. 13b). Under visible light, AgBr is partially reduced to Ag and attached to the surface of the SnO2–AgBr composite to form Ag@SnO2–AgBr. Ag has two roles in a Z-scheme mechanism: as an electronic medium and as a photosensitizer.80,81 From the UV-vis results (Fig. 7a), it can be seen that AgBr responds to visible light, while SnO2 cannot, so Ag serves as both a photosensitizer and an electronic medium.82,83 Ag assumed the responsibility for visible light absorption, not SnO2. The photogenerated electrons (eCB1−) in the AgBr conduction band combine with the photogenerated holes (hVB2+) in the highest occupied orbital of Ag, while the photogenerated electrons (eCB2−) in the lowest unoccupied orbital of Ag migrate to the conduction band of SnO2. The photogenerated electrons (eCB3−) are enriched in the conduction band of SnO2 and the holes (hVB1+) are enriched in the valence band of AgBr, and the photogenerated hole–electron separation of AgBr is realized (eqn (3)–(10)).
In addition, since the ECB of SnO2 (−0.16 eV vs. NHE) is lower than that of O2 (−0.046 eV vs. NHE), ·O2− can be produced. Similarly, the EVB of AgBr (2.60 eV vs. NHE) is higher than that of OH-/H2O (2.30 eV vs. NHE), and.
·OH can be formed normally. The Z-scheme mechanism of SnO2–AgBr composite well reveals its superior photocatalytic performance.
|
AgBr + hν → eCB1− + hVB1+
| (3) |
|
AgNPs + hν → eCB2− + hVB2+
| (5) |
|
eCB1− + hVB2+ → recombination
| (6) |
|
eCB2− + AgNPs → SnO2 + eCB3−
| (7) |
|
hVB1+ + H2O →·OH + H+
| (8) |
|
·OH/·O2−/h+ + MO/MG → intermediate product + CO2 + H2O
| (10) |
3.6 Prediction of photocatalytic degradation pathway
In order to further study the degradation process of MO/MG, samples of 0 min, 15 min, 60 min and 120 min were taken for HPLC-MS analysis, and the results are shown in Fig. 14. At t = 0, m/z 304.1 is the diffraction peak of MO (Fig. 14a), which is an ionic state formed when MO molecules dissolve in water and remove Na+, while m/z 329.2 corresponds to the characteristic peak of MG (Fig. 14e).84,85 With the progress of the reaction, it was found that the diffraction peaks of MO and MG were significantly reduced and gradually transformed into small molecules. The main m/z of MO were 338.0, 175.3, 122.6, 157.0, 108.3 and 94.4, while the m/z of MG were 347.2, 329.2, 315.1, 301.0, 273.0, 226.1 and 150.1.
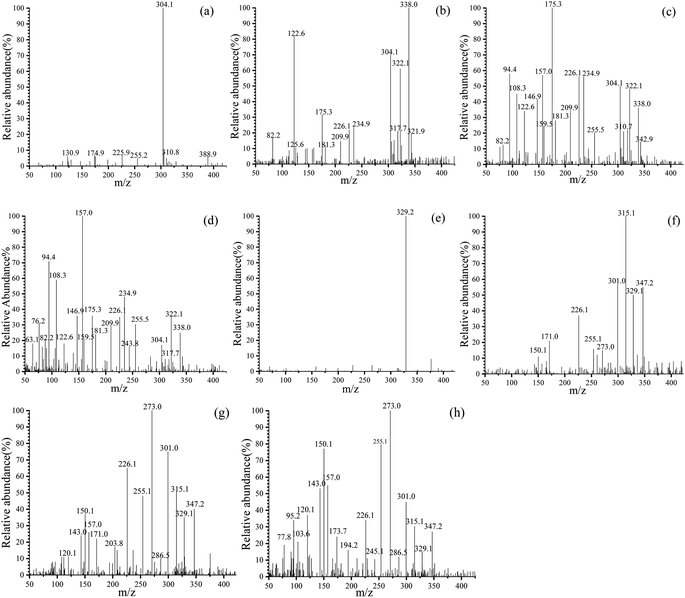 |
| Fig. 14 Mass spectrum of the intermediates of MO at: (a) 0 min, (b) 15 min, (c) 60 min, (d) 120 min; and of MG at: (e) 0 min, (f) 15 min, (g) 60 min, (h) 120 min. | |
Based on the m/z generated in different times, the degradation pathways for MO were proposed (Fig. 15 MO). The benzene ring in A (m/z 304.1) is substituted by ·OH to form product B;86 B is attacked by active substances; the C–N bond breaks due to low bond energy, forming products C, D and N2;87 C removes ·OH to form product E; and D generates products F and G by demethylation.
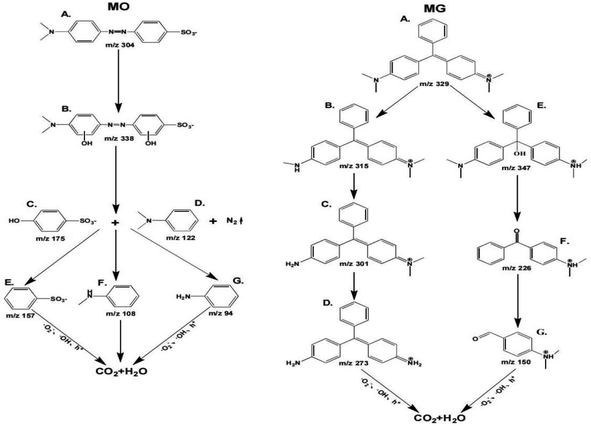 |
| Fig. 15 Prediction of degradation pathways of MO and MG. | |
The degradation pathways of MG (Fig. 15 MG) are: first, A (m/z 329) generates B through demethylation, which is the main pathway of photocatalytic degradation and mineralization.88 Subsequently, B is further demethylated to produce C and D; second, A (m/z 329) undergoes decarboxylation to form E; the covalent bond between the benzene ring and the central carbon atom is broken, forming F; G is formed by debenzenization of F. Subsequently, the intermediate of MO/MG will be degraded into CO2 and H2O.
4. Conclusions
An SnO2–AgBr composite showing a Z-scheme mechanism was successfully prepared by a simple hydrothermal procedure and in situ precipitation method. Characterization and photocatalytic experiments showed that the composite performance was best when the molar ratio of SnO2 to AgBr was 1
:
1. The Z-scheme mechanism reduces the photogenic hole–electron recombination rate of AgBr, and produces a large number of ·OH, ·O2− and h+, and the degradation rates of simulated MO and MG wastewater reach 96.74% and 93.36%, respectively. Different factors have different effects on the degradation efficiency of simulated dye wastewater. The effect of pH on the degradation rate was small, indicating that the composite had good acid–alkali resistance. The degradation rate increased with composite dosage, and the humic acid concentration was negatively correlated with the degradation rate, which played an inhibitory role. According to mass spectrometry analysis, the degradation pathway of MO and MG proceeded through bond-breaking oxidation, ring-opening and deep oxidation decomposition into intermediate products, and finally degradation into CO2 and H2O. The repeatability results of SnO2–AgBr are not very good, because the silver-based photocatalyst easily undergoes photocorrosion. It is hoped that this study can provide a reference scheme for the design and preparation of a new Z-scheme mechanism, and realize the more efficient, economical and green application of photocatalysis in the field of water treatment.
Conflicts of interest
There are no conflicts to declare.
Acknowledgements
The authors acknowledge the support of Xiaoliang Yang, Yukai Wang and Fei Tang, and their friends in College of Civil Engineering, Guizhou University, Guiyang, China.
References
- A. Shahi, B. N. Rai and R. S. J. A. B. Singh, Biotechnology, Biodegradation of Reactive Orange 16 Dye in Microbial Fuel Cell: An Innovative Way to Minimize Waste Along with Electricity Production, Appl. Biochem. Biotechnol., 2020, 192(2), 196–210 CrossRef CAS PubMed.
- T. Angelika, M. Kamila and P. Andrzej, Synthetic organic dyes as contaminants of the aquatic environment and their implications for ecosystems: A review, Sci. Total Environ., 2020, 717(15), 137222 Search PubMed.
- S. Li, C. Wang, Y. Liu, M. Cai, Y. Wang, H. Zhang, Y. Guo, W. Zhao, Z. Wang and X. Chen, Photocatalytic degradation of tetracycline antibiotic by a novel Bi2Sn2O7/Bi2MoO6 S-scheme heterojunction: Performance, mechanism insight and toxicity assessment, Chem. Eng. J., 2022, 429, 132519 CrossRef CAS.
- T. Liu, C. Wang, C. Ding, W. Wang, B. Wang, M. Wang and J. Zhang, The improved photocatalytic antibiotic removal performance achieved on Ir/WO2.72 photocatalysts, Colloids Surf., A, 2022, 645, 128891 CrossRef CAS.
- T. H. A. Nguyen, V. T. Le, V. D. Doan, A. V. Tran, V. C. Nguyen and Y. Vasseghian, Green synthesis of Nb-doped ZnO nanocomposite for photocatalytic degradation of tetracycline antibiotic under visible light, Mater. Lett., 2022, 308, 131129 CrossRef CAS.
- Z. H. Diao, S. T. Huang, X. Chen, M. Y. Zou, H. Liu, P. R. Guo, L. J. Kong and W. Chu, Peroxymonosulfate-assisted photocatalytic degradation of antibiotic norfloxacin by a calcium-based Ag3PO4 composite in water: Reactivity, products and mechanism, J. Cleaner Prod., 2022, 330, 129806 CrossRef CAS.
- X. Hu, Y. Yu, D. Chen, W. Xu, J. Fang, Z. Liu, R. Li, L. Yao, J. Qin and Z. Fang, Anatase/Rutile homojunction quantum dots anchored on g-C3N4 nanosheets for antibiotics degradation in seawater matrice via coupled adsorption-photocatalysis: Mechanism insight and toxicity evaluation, Chem. Eng. J., 2022, 432, 134375 CrossRef CAS.
- Y. Xie, Y. Yu, H. Xie, F. Huang and T. C. J. J. o. h. m. Hughes, 3D-printed heterogeneous Cu2O monoliths: Reusable supports for Antibiotic Treatmentantibiotic treatment of wastewater, J. Hazard. Mater., 2022, 2(435), 1–14 Search PubMed.
- X. Zhang, B. Xu, S. Wang, X. Li, B. Liu, Y. Xu, P. Yu and Y. J. J. o. H. M. Sun, High-density dispersion of CuNx sites for H2O2 activation toward enhanced Photo-Fenton performance in antibiotic contaminant degradation, J. Hazard. Mater., 2022, 423, 127039 CrossRef CAS PubMed.
- L. Jing, Y. Xu, J. Liu, M. Zhou and J. Xie, Direct Z-scheme red carbon nitride/rod-like lanthanum vanadate composites with enhanced photodegradation of antibiotic contaminants, Appl. Catal., B, 2020, 277, 119245 CrossRef CAS.
- A. Wieckowska, E. Jablonowska, M. Dzwonek, M. Jaskolowski and R. J. C. Bilewicz, Tailored Lipid Monolayers Doped with Gold Nanoclusters: Surface Studies and Electrochemistry of Hybrid-film-covered Electrodes, ChemElectroChem, 2022, 9(9), e202101367 CrossRef CAS.
- C. Li, Y. Guo, D. Tang, Y. Guo, G. Wang, H. Jiang and J. Li, Optimizing electron structure of Zn-doped AgFeO2 with abundant oxygen vacancies to boost photocatalytic activity for Cr(VI) reduction and organic pollutants decomposition: DFT insights and experimental - ScienceDirect, Chem. Eng. J., 2021, 411, 128515 CrossRef CAS.
- T. Liu, C. Wang, M. Wang, J. Bai and Q. Zhou, The improved spatial charge separation and antibiotic removal performance on Z-scheme Zn-Fe2O3/ZnIn2S4 architectures, Colloids Surf., A, 2021, 628, 127226 CrossRef CAS.
- S. Li, Y. Zhang and H. Huang, Black phosphorus-based heterostructures for photocatalysis and photoelectrochemical water splitting, J. Energy Chem., 2022, 67, 745–779 CrossRef CAS.
- Y. Jia, S. Li, H. Ma, J. Gao, G. Zhu, F. Zhang, J. Y. Park, S. Cha, J. S. Bae and C. Liu, Oxygen vacancy rich Bi2O4-Bi4O7-BiO2-x composites for UV-vis-NIR activated high efficient photocatalytic degradation of bisphenol A, J. Hazard. Mater., 2020, 382(15), 121121 CrossRef CAS PubMed.
- M. Ghrib, T. Ghrib, W. Dimassi, M. Gaidi, R. Ouertani and H. Ezzaouia, Correlation between microstructural and optoelectronic properties of porous Si/SnO2 composites coated Al2O3, Optik, 2022, 258, 168900 CrossRef CAS.
- F. G. El Desouky, M. M. Saadeldin, M. A. Mahdy and I. K. El Zawawi, Tuning the structure, morphological variations, optical and magnetic properties of SnO2/NiFe2O4 nanocomposites for promising applications, Vacuum, 2021, 185, 110003 CrossRef CAS.
- N. P. Reddy, R. Muniramaiah, R. Santhosh, J. M. Fernandes, D. B. Padmanaban, G. Maharana, M. Kovendhan, D. P. Joseph and B. Murali, Cost-effective Sb-doped SnO2 films as stable and efficient alternative transparent conducting electrodes for dye-sensitized solar cells, J. Mater. Chem. C, 2022, 20, 7997–8008 RSC.
- D. Liu, W. Zhang, Z. Ren and X. Li, Yb-doped SnO2 electron transfer layer assisting the fabrication of high-efficiency and stable perovskite solar cells in air, RSC Adv., 2022, 12, 14631–14638 RSC.
- P. Chen and Y. Wang, Improved arc erosion resistance of Ag/SnO2 composites fabricated by Ag melt-infiltration in the SnO2 skeleton, Mater. Lett., 2022, 310, 131520 CrossRef CAS.
- D. Maestre, E. Hernández, A. Cremades, M. Amati and J. Piqueras, Design, Synthesis and Characterization of Small Dimensional Structures of Er− Doped SnO2 and ErbiumTinOxide, Cryst. Growth, 2020, 12(5), 2478–2484 CrossRef.
- S. B. O. Dos Santos, M. H. Boratto, R. A. Ramos Jr and L. V. A. Scalvi, Influence of Pb2+ doping in the optical and electro-optical properties of SnO2 thin films, Mater. Chem. Phys., 2022, 278, 125571 CrossRef CAS.
- L. Gnanasekaran, S. Rajendran, P. S. Kumar, A. K. Priya, F. Gracia, M. A. Habila and K. Saravanakumar, Visible light stimulated binary nanostructure and defect enriched TiO2-SnO2 for photocatalysis and antibacterial activity, Mater. Lett., 2022, 316, 131998 CrossRef CAS.
- L. Huang, J. Yao, L. Yang, J. Liu, J. Liu, S. Shu, M. Hua, Y. Song and H. Zhu, Compounds, Construction of Z-scheme Bi12O15Cl6/SnO2-x heterojunction for enhanced photocatalytic degradation of dyes and antibiosis, J. Alloys Compd., 2022, 908, 164395 CrossRef CAS.
- W. Ren, J. Yang, J. Zhang, W. Li, C. Sun, H. Zhao, Y. Wen, O. Sha and B. Liang, Recent progress in SnO2/g-C3N4 heterojunction photocatalysts: Synthesis, modification, and application, J. Alloys Compd., 2022, 906, 164372 CrossRef CAS.
- Z. Fan, J. Luan, C. Zhu and F. J. M. R. B. Liu, Depositing Ag2S quantum dots as electron mediators in SnS2/g-C3N4 nanosheet composites for constructing Z-scheme heterojunction with enhanced photocatalytic performance, Mater. Res. Bull., 2021, 133, 111045 CrossRef CAS.
- G. N. Silva, T. A. Martins, I. C. Nogueira, R. K. Santos, M. S. Li, E. Longo and G. Botelho, Synthesis of Ag3PO4/SnO2 composite photocatalyst for improvements in photocatalytic activity under visible light, Mater. Sci. Semicond. Process., 2021, 135, 106064 CrossRef CAS.
- Y. Lian, Y. Wang, D. Zhang and L. Xu, Visible light-driven photocatalytic and enzyme-like properties of novel AgBr/Ag2MoO4 for degradation of pollutants and improved antibacterial application, Colloids Surf., A, 2022, 639, 128348 CrossRef CAS.
- X. Hu, Q. Ma, X. Wang, Y. Yang, N. Liu, C. Zhang, N. Kawazoe, G. Chen and Y. Yang, Layered Ag/Ag2O/BiPO4/Bi2WO6 heterostructures by two-step method for enhanced photocatalysis, J. Catal., 2020, 387, 387 CrossRef.
- B. Babu, R. Koutavarapu, V. V. N. Harish, J. Shim and K. Yoo, Novel in situ synthesis of Au/SnO2 quantum dots for enhanced visible-light-driven photocatalytic applications, Ceram. Int., 2019, 45(5), 5743–5750 CrossRef CAS.
- M. A. Ahmed, A. Fahmy, M. G. Abuzaid and E. M. Hashem, Fabrication of novel AgIO4/SnO2 heterojunction for photocatalytic hydrogen production through direct Z-scheme mechanism, J. Photochem. Photobiol., A, 2020, 400, 112660 CrossRef CAS.
- P. Wang, Y. Liu, N. Jiang, R. Jing and Y. Liu, Double S-scheme AgBr heterojunction co-modified with g-C3N4 and black phosphorus nanosheets greatly improves the photocatalytic activity and stability, J. Mol. Liq., 2021, 329, 115540 CrossRef CAS.
- S. Man, X. Zeng, Z. Yin, H. Yang, H. Bao, K. Xu, L. Wang, X. Ge, Z. Mo and W. J. E. A. Yang, Preparation of a novel Ce and Sb co-doped SnO2 nanoflowers electrode by a two-step (hydrothermal and thermal decomposition) method for organic pollutants electrochemical degradation, Electrochim. Acta, 2022, 411, 140066 CrossRef CAS.
- M. Herrera, D. Maestre, A. Cremades and J. Piqueras, Growth and Characterization of Mn Doped SnO2 Nanowires, Nanobelts, and Microplates, J. Phys. Chem. C, 2020, 117(17), 8997–9003 CrossRef.
- Y. Zhang, Y. Zhao, Z. Xiong, T. Gao and J. Zhang, Elemental mercury removal by I-doped Bi2WO6 with remarkable visible-light-driven photocatalytic oxidation, Appl. Catal., B, 2021, 282, 119534 CrossRef CAS.
- X. Feng, S. Zhang, F. Wang, J. Ma and X. Wang, Metallic Ag Confined on SnO2 Surface for Soot Combustion: the Influence of Ag Distribution and Dispersion on the Reactivity, ChemCatChem, 2021, 13(9), 2222–2233 CrossRef CAS.
- A. Mohammad, M. E. Khan, I. M. Alarifi, M. H. Cho and T. Yoon, A sensitive electrochemical detection of hydrazine based on SnO2/CeO2 nanostructured oxide, Microchem. J., 2021, 171, 106784 CrossRef CAS.
- J. Shin, J. N. Heo, J. Y. Do, Y. I. Kim and M. Kang, Effective charge separation in rGO/NiWO4@Au photocatalyst for efficient CO2 reduction under visible light, J. Ind. Eng. Chem., 2019, 81, 427–439 CrossRef.
- T.-Y. Liang, S.-J. Chan, A. S. Patra, P.-L. Hsieh, Y.-A. Chen, H.-H. Ma and M. H. Huang, Inactive Cu2O Cubes Become Highly Photocatalytically Active with Ag2S Deposition, ACS Appl. Mater. Interfaces, 2021, 13(9), 11515–11523 CrossRef CAS PubMed.
- X. Shen, J. Yang, T. Zheng, Q. Wang, H. Zhuang, R. Zheng, S. Shan and S. Li, Plasmonic p–n heterojunction of Ag/Ag2S/Ag2MoO4 with enhanced Vis-NIR photocatalytic activity for purifying wastewater, Sep. Purif. Technol., 2020, 251, 117347 CrossRef CAS.
- B. Xue, H.-Y. Jiang, T. Sun, F. Mao, C.-C. Ma and J.-K. Wu, Microwave-assisted one-step rapid synthesis of ternary Ag/Ag2S/g-C3N4 heterojunction photocatalysts for improved visible-light induced photodegradation of organic pollutant, J. Photochem. Photobiol., A, 2018, 353, 557–563 CrossRef CAS.
- X. Dong, S. Wang, Q. Wu, K. Liu, F. Kong and J. Liu, Co-catalyst boosted photocatalytic hydrogen production driven by visible-light over g-C3N4 : The synergistic effect between Ag and Ag2S, J. Alloys Compd., 2021, 875, 160032 CrossRef CAS.
- H. Zhang, D. Yu, W. Wang, P. Gao and B. Liu, Construction of a novel BON-Br-AgBr heterojunction photocatalysts as a direct Z-scheme system for efficient visible photocatalytic activity, Appl. Surf. Sci., 2019, 497, 143820 CrossRef CAS.
- D. Liu, L. Feng, L. Cao, T. Xiao and J. Huang, W/O site replace by Ce/N of Bi2WO6 as cations/anions to regulate the reduction potential of conduction band for enhanced photocatalytic degradation and hydrogen evolution capacity, J. Alloys Compd., 2022, 890, 161920 CrossRef CAS.
- H. Katsumata, M. A. I. Molla and J. B. Islam, Dual Z-scheme heterojunction g-C3N4/Ag3PO4/AgBr photocatalyst with enhanced visible-light photocatalytic activity, Ceram. Int., 2022, 48(15), 21898–21905 CrossRef.
- Y. Li, M. Liu, M. Zhang, Y. Liu, Q. Zhao, X. Li, Q. Zhou, Y. Chen and S. Wang, Preparation of g-C3N4/TiO2 Heterojunction Composite Photocatalyst by NaCl Template Method and Its Photocatalytic Performance Enhancement, Nano, 2023, 18(02), 2350009 CrossRef CAS.
- J. Wang, G. Wang, B. Cheng, J. Yu and J. Fan, Sulfur-doped g-C3N4/TiO2 S-scheme heterojunction photocatalyst for Congo Red photodegradation, Chin. J. Catal., 2021, 42(1), 56–68 CrossRef CAS.
- X. Guo, H. Wang, L. Wang, D. Zeng and Q. Xiang, Triple-Mode Bi2WO6/Pg-C3N4@rGO Core-Shell Synergistic Effect with Enhanced Light-induced Photocatalytic Activity, Bull. Korean Chem. Soc., 2019, 40(3), 254–261 CrossRef CAS.
- P. Bai, N. Wu, Y. Wang, T. Yang and X. Wang, PH-controllable Regeneration and Visible-light Photocatalytic Redox of Carbon and Nitrogen Codoped Zn3Nb2O8 towards Multiple Contaminants, Catal. Sci. Technol., 2020, 10(9), 2810–2820 RSC.
- A. Q. Alorabi, Effective Removal of Malachite Green from Aqueous Solutions Using Magnetic Nanocomposite: Synthesis, Characterization, and Equilibrium Study, Adsorpt. Sci. Technol., 2021, 2021, 2359110 Search PubMed.
- P. Wang, Y. Cao, X. Zhou, C. Xu and Q. Yan, Facile construction of 3D hierarchical flake ball-shaped γ-AgI/Bi2WO6 Z-scheme heterojunction towards enhanced visible-light photocatalytic performance - ScienceDirect, Appl. Surf. Sci., 2020, 531, 147345 CrossRef CAS.
- M. K. A. Mohammed, Lithium-doped SnO2@CeO2nanoparticles for highly photodegradation of Rhodamine B dye upon visible light irradiation, Int. J. Mod. Phys. B, 2023, 37(04), 2350036 CrossRef CAS.
- N. K. Razdan and A. Bhan, Catalytic site ensembles: A context to reexamine the Langmuir-Hinshelwood kinetic description, J. Catal., 2021, 404, 726–744 CrossRef CAS.
- C. Han, L. Ge, C. Chen, Y. Li, X. Xiao, Y. Zhang and L. Guo, Novel visible light induced Co3O4-g-C3N4 heterojunction photocatalysts for efficient degradation of methyl orange, Appl. Catal., B, 2014, 147, 546–553 CrossRef CAS.
- M. May-Lozano, R. Lopez-Medina, V. Mendoza-Escamilla, G. Rivadeneyra-Romero and S. Martínez-Delgadillo, Intensification of the Orange II and Black 5 degradation by sonophotocatalysis using Ag-graphene oxide/TiO2 systems, Chem. Eng. Process., 2020, 158, 108175 CrossRef CAS.
- Y. Song, J. Qi, J. Tian, S. Gao and F. Cui, Construction of Ag/g-C3N4 photocatalysts with visible-light photocatalytic activity for sulfamethoxazole degradation, Chem. Eng. J., 2018, 341, 547–555 CrossRef CAS.
- X. Lei, M. You, F. Pan, M. Liu, P. Yang, D. Xia, Q. Li, Y. Wang and J. Fu, CuFe2O4@GO nanocomposite as an effective and recoverable catalyst of peroxymonosulfate activation for degradation of aqueous dye pollutants, Chin. Chem. Lett., 2019, 30(12), 245–249 CrossRef.
- Y. Shaveisi and S. Sharifnia, Deriving Ag_3PO_4–CaO composite as a stable and solar light photocatalyst for efficient ammonia degradation from wastewater, J. Energy Chem., 2018, 27(01), 304–313 Search PubMed.
- Y. Yang, X. Hu, Y. Zhao, L. Cui, Z. Huang, J. Long, J. Xu, J. Deng, C. Wu and W. Liao, Decontamination of tetracycline by thiourea-dioxide-reduced magnetic graphene oxide: Effects of pH, ionic strength, and humic acid concentration, J. Colloid Interface Sci., 2017, 495, 68–77 CrossRef CAS PubMed.
- A. Yqg, B. Nyg, B. Whc, B. Yfz, Z. A. Jia and B. Dqy, UV-activated persulfate oxidation of sulfamethoxypyridazine: Kinetics, degradation pathways and impact on DBP formation during subsequent chlorination, Chem. Eng. J., 2019, 370, 706–715 CrossRef.
- J. Niu, Y. Li and W. Wang, Light-source-dependent role of nitrate and humic acid in tetracycline photolysis: Kinetics and mechanism, Chemosphere, 2013, 92(11), 1423–1429 CrossRef CAS PubMed.
- R. Cheng, M. Kang, Z. Shen, L. Shi and X. Zheng, Visible-light-driven photocatalytic inactivation of bacteriophage f2 by Cu-TiO2 nanofibers in the presence of humic acid, J. Environ. Sci., 2019, 77, 383–391 CrossRef CAS PubMed.
- T. Tang, G. Lu, W. Wang, R. Wang, K. Huang, Z. Qiu, X. Tao and Z. Dang, Photocatalytic removal of organic phosphate esters by TiO2: Effect of inorganic ions and humic acid, Chemosphere, 2018, 206, 26–32 CrossRef CAS PubMed.
- A. Cai, J. Deng, M. Xu, T. Zhu, S. Zhou, J. Li, G. Wang and X. Li, Degradation of tetracycline by UV activated monochloramine process: Kinetics, degradation pathway, DBPs formation and toxicity assessment, Chem. Eng. J., 2020, 395, 125090 CrossRef CAS.
- Z. Hassan, Effects of pH on Antibiotic Denitrification and Biodegradation of Sulfamethoxazole Removal from Simulated Municipal Wastewater by a Novel 3D-BER System, J. Environ. Eng., 2020, 146(12), 04020134 CrossRef.
- Q. Chen, B. He, Y. Ma, X. Wang, Q. Xiong, J. Li and Q. H. J. P. P. Liu, Polymers, Influence of the pH value on the degradation of an azo dye of methyl orange by air discharge plasma, Plasma Processes Polym., 2019, 16(5), 1800152 CrossRef.
- M. F. Hanafi and N. Sapawe, Influence of pH on the photocatalytic degradation of methyl orange using nickel catalyst, Mater. Today: Proc., 2020, 31, 339–341 CAS.
- Y. Chen, Y. Zhang, C. Liu, A. Lu and W. Zhang, Photodegradation of Malachite Green by Nanostructured Bi WO Visible Light-Induced Photocatalyst, Int. J. Photoenergy, 2011, 2012(1), 1302–1312 Search PubMed.
- W. Li, M. Xu, Q. Cao, J. Luo, S. Yang and G. Zhao, Magnetic GO/Fe3O4 for rapid malachite green (MG) removal from aqueous solutions: a reversible adsorption, RSC Adv., 2021, 11, 19387–19394 RSC.
- E. Sharifpour, E. A. Dil, A. Asfaram, M. Ghaedi and A. Goudarzi, Optimizing adsorptive removal of malachite green and methyl orange dyes from simulated wastewater by Mn-doped CuO-Nanoparticles loaded on activated carbon using CCD-RSM: Mechanism, regeneration, isotherm, kinetic, and thermodynamic studies, Appl. Organomet. Chem., 2019, 33(3), e4678 CrossRef.
- A.-T. Vu, V. H. Mac, T. H. Nguyen and T. H. Nguyen, Preparation of carnation-like Ag-ZnO composites for enhanced photocatalysis under visible light, Nanotechnology, 2023, 34(27), 275602 CrossRef PubMed.
- B. Prakash, V. Katoch, A. Shah, M. Sharma and A. K. Ganguli, Continuous Flow Reactor for The Controlled Synthesis and Inline Photocatalysis of Antibacterial Ag2S Nanoparticles, Photochem. Photobiol., 2020, 96(6), 1273–1282 CrossRef CAS PubMed.
- P. P. Tun, J. Wang, T. T. Khaing, X. Wu and G. Zhang, Fabrication of functionalized plasmonic Ag loaded Bi2O3/montmorillonite nanocomposites for efficient photocatalytic removal of antibiotics and organic dyes, J. Alloys Compd., 2019, 818, 152836 CrossRef.
- G. Yentür and M. Dükkancı, Fabrication of magnetically separable plasmonic composite photocatalyst of Ag/AgBr/ZnFe2O4 for visible light photocatalytic oxidation
of carbamazepine, Appl. Surf. Sci., 2020, 510, 145374 CrossRef.
- A. Rezaei, M. R. Rezaei and M. H. Sayadi, 3D network structure graphene hydrogel-Fe3O4@SnO2/Ag via an adsorption/photocatalysis synergy for removal of 2,4 dichlorophenol, J. Taiwan Inst. Chem. Eng., 2021, 121, 154–167 CrossRef CAS.
- J. Lan, B. He, C. Haw, M. Gao and J. Kang, Band Engineering of ZnO/Si Nanowire Arrays in Z-Scheme Heterojunction for Efficient Dye Photodegradation, Appl. Surf. Sci., 2020, 529, 147023 CrossRef CAS.
- Y. Xue, W. Tang, H. Gu, M. Wei, E. Guo, Q. Lu and Y. Pang, Flexible Bi2MoO6/N-doped carbon nanofiber membrane enables tetracycline photocatalysis for environmentally safe growth of Vigna radiata, J. Alloys Compd., 2022, 902, 163860 CrossRef CAS.
- H. Shen, X. Zhao, L. Duan, R. Liu and H. Li, Enhanced visible light photocatalytic activity in SnO2@g-C3N4 core-shell structures, Mater. Sci. Eng., B, 2017, 218, 23–30 CrossRef CAS.
- S. Zarezadeh, A. Habibi-Yangjeh and M. Mousavi, BiOBr and AgBr co-modified ZnO photocatalyst: A novel nanocomposite with p-n-n heterojunctions for highly effective photocatalytic removal of organic contaminants, J. Photochem. Photobiol., A, 2019, 379, 11–23 CrossRef CAS.
- M. Ge and Z. Li, All-Solid-State Z-Scheme Photocatalytic Systems Based on Silver-Containing Semiconductor Materials, Prog. Chem., 2017, 29(8), 846–858 CAS.
- H. Guo, C. G. Niu, L. Zhang, X. J. Wen, C. Liang, X. G. Zhang, D. L. Guan, N. Tang and G. Zeng, Engineering, Construction of direct Z-scheme AgI/Bi2Sn2O7 nanojunction system with enhanced photocatalytic activity: Accelerated interfacial charge transfer induced efficient Cr(VI) reduction, tetracycline degradation and Escherichia coli inactivation, ACS Sustainable Chem., 2018, 6(6), 8003–8018 CrossRef CAS.
- R. Xie, L. Zhang, H. Xu, Y. Zhong, X. Sui and Z. Mao, Fabrication of Z-scheme photocatalyst Ag-AgBr@Bi20TiO32 and its visible-light photocatalytic activity for the degradation of isoproturon herbicide, J. Mol. Catal. A: Chem., 2015, 406, 194–203 CrossRef CAS.
- X. Wang, S. Li, Y. Ma, H. Yu and J. Yu, H2WO4 center dot H2O/Ag/AgCl Composite Nanoplates: A Plasmonic Z-Scheme Visible-Light Photocatalyst, J. Phys. Chem. C, 2011, 115(30), 14648–14655 CrossRef CAS.
- L. V. Trandafilović, D. J. Jovanović, X. Zhang, S. Ptasińska and M. D. Dramićanin, Enhanced photocatalytic degradation of methylene blue and methyl orange by ZnO:Eu nanoparticles, Appl. Catal., B, 2017, 203, 740–752 CrossRef.
- A. Barapatre, K. R. Aadil and H. Jha, Biodegradation of Malachite Green by the Ligninolytic Fungus Aspergillus flavus, Clean: Soil, Air, Water, 2017, 45(4), 1600045 Search PubMed.
- J. Kaur and S. Singhal, Facile synthesis of ZnO and transition metal doped ZnO nanoparticles for the photocatalytic degradation of Methyl Orange, Ceram. Int., 2014, 40(5), 7417–7424 CrossRef CAS.
- A. Bahramian, M. Rezaeivala, K. He and D. D. Dionysiou, Enhanced visible-light photoelectrochemical hydrogen evolution through degradation of methyl orange in a cell based on coral-like Pt-deposited TiO2 thin film with sub-2 nm pores, Catal. Today, 2019, 335, 333–344 CrossRef CAS.
- B. P. Sanjay, S. Sandeep, A. S. Santhosh, C. S. Karthik, D. N. Varun, N. K. Swamy, P. Mallu, K. S. Nithin, R. J. Ramalingam and K. Muthusamy, Unprecedented 2D GNR-CoB nanocomposite for detection and degradation of malachite green – A computational prediction of degradation pathway and toxicity, Chemosphere, 2022, 287, 132153 CrossRef CAS PubMed.
|
This journal is © The Royal Society of Chemistry 2023 |
Click here to see how this site uses Cookies. View our privacy policy here.