DOI:
10.1039/D3RA05993F
(Paper)
RSC Adv., 2023,
13, 29944-29958
Ba2−xHoxSr2−yNiyFe12O22 and its composite with MXene: synthesis, characterization and enhanced visible light mediated photocatalytic activity for colored dye and pesticide
Received
3rd September 2023
, Accepted 5th October 2023
First published on 13th October 2023
Abstract
The rapid recombination of charges of photogenerated electrons and holes severely limits single semiconductor photocatalytic applications. In this study, a simple and facile sol–gel approach was used to synthesize Ba2−xHoxSr2−yNiyFe12O22 (x = 0, 0.1 and y = 0, 0.5). The composite of holmium–nickel doped barium–strontium ferrite with MXene (Ba1.9Ho0.1Sr1.5Ni0.5Fe12O22@MXene) was synthesized by ultrasonication method. These synthesized samples were subsequently used to photodegrade rhodamine B (RhB) and pendimethalin under visible light illumination. The results of the experiments demonstrated that MXene, as a cocatalyst, considerably reduces the rate of recombination of charges and broadens absorption of visible light by providing increased surface functional groups to improve the photocatalytic activity of synthesized samples. MXene is thermally stable, have high electrical conductivity, have adjustable bandgap, and hydrophilic in nature. The optimized Ba1.9Ho0.1Sr1.5Ni0.5Fe12O22@MXene composite demonstrated an excellent photocatalytic rate by degrading 78.88% RhB and 75.59% pendimethalin in 140 minutes. Moreover, the scavenging experiment revealed that photogenerated electrons and holes were the primary active species involved in RhB and pendimethalin photodegradation, respectively. Ba1.9Ho0.1Sr1.5Ni0.5Fe12O22@MXene showed increased photocatalytic behavior because it has increased surface area which decreases rate of recombination of electron and hole pair, hence photocatalytic activity increases. It is observed that Ba1.9Ho0.1Sr1.5Ni0.5Fe12O22@MXene has potential application in photocatalytic degradation of harmful pollutants.
1. Introduction
Grain yields have increased recently as a result of intensive farming methods. However, intensive agricultural techniques and overuse of agrochemicals have caused herbicide build-up in the soil, drinking water and groundwater.1 Approximately 2 million tons of pesticides are being used annually worldwide and of which 47.5% are herbicides. The family of nitroaromatic compounds known as dinitroaniline herbicides include pendimethalin, nitralin, dinitramine, trifluralin etc. Dinitroanilines often reach the soil during agricultural applications and may also do so through surface runoff, groundwater discharge, field drainage, and spray drift as well as surface waters and sediments.2 Dinitroaniline herbicide “pendimethalin” is widely used on a variety of crops, including cereals (wheat, barley, triticale, rye etc.), vegetables, potatoes, rice, legumes, maize, soybeans, nuts and fruits as well as ornamental plants and trees, to control grassy weed species and broadleaf weeds. Pendimethalin separates the chromosomes of harmful weeds and prevents their cell division and the formation of cell wall.3 So, herbicide removal is one of the major and challenging tasks to prevent pollution of the environment. Some recent techniques for the removal of herbicides from polluted water include electrochemical oxidation, biological treatments, adsorption, photocatalysis and a combination of photocatalysis and ozonation.4 Since many herbicides are stubborn pollutants, they must be eliminated.
Rhodamine B (RhB) is an organic dye, soluble in water and used in water tracing, fabric colouring, laser dyes, photosensitizers and fluorescent markers for microscopic structural testing. It is also used in biomedical research as a biological stain. Since it is carcinogenic in nature, its use in food processing is prohibited. Because of industrialization and unmonitored discharge of RhB, RhB severely contaminate fish and other species present in water that enters the food chain and have hazardous effects on humans and animals.5
Photocatalysis, which can result in the pollutant's total annihilation or mineralization, is an excellent choice for elimination of contaminants from waste water.6,7 According to reports, semiconductor metal oxide photocatalysis is a remarkable green method for combating environmental pollution.8 Ferrites, having excellent magnetic properties, have been studied and used for over 50 years.9 The term “ferrite” is often used to describe a type of magnetic oxide compound having ferromagnetic characteristics that are found in powder or ceramic bodies and include a substantial amount of iron oxide. Ferrites are made up of transition metals and lanthanides especially chromium, iron and manganese. Their general formula is M′M′′2O4, where M′ is divalent metal cation (such as Mg2+, Fe2+, Cu2+, Ni2+, Zn2+, and Co2+) and M′′ is trivalent metal ions (such as Fe3+, Al3+, Cr3+, and Mn3+ etc.).10 Ferrites are dark grey or black, hard and brittle oxide ceramics.11 Iron ore magnetite (Fe3O4) is natural ferrite, having the chemical formula Fe2+Fe23+O4. Its equivalent general formula is formulated as Me2+Fe23+O4, here Me represents divalent metal cation (such as Cu, Mn, Co, Mg, Cd, Co, Ni, Zn etc.). The three forms of ferrites that may be distinguished by their crystal structures are spinel (MFe2O4), garnet (M3Fe5O12), and hexagonal (MFe12O19), where M stands for divalent transition metal (Cu, Mn, Zn, Fe, Ni and Co).12 While garnet and spinel are magnetically soft, hexagonal are hard. In the AO–Fe2O3–MeO system, hexagonal ferrites are a group of complex oxides, where A is Ca, Ba or Sr, etc., and Me is Fe, Zn, Ni, Co, Cu or Mn, etc.13 In the research labs of Philips, hexagonal ferrites were studied about 60 years ago.14 The first magnetic mineral, magnetoplumbite, was given its name in 1925. In 1938, the PbFe7.5Mn3.5Al0.5Ti0.5O19 crystal structure was identified. It was shown that PbFe12O19 made up the magnetoplumbite configuration in the simulation.15 Magnetoplumbite are a class of magnetic oxides with hard magnetic characteristics and a hexagonal crystal structure. Recently, it was shown that several hexaferrites display single-phase multiferroic properties (materials with ferroelectric, ferroelastic, and ferromagnetic properties). Due to these characteristics, interest in hexagonal ferrites has grown significantly during the past years.16 They are used in photocatalysis because of the light frequency they absorb for photocatalytic reaction and also due to their magnetic nature. They contain more catalytic sites which enhances their catalytic activity. They are used in degrading organic contaminants, dyes, CO2, and as an antimicrobial agent.17 Raut, S. S., et al., reported the synthesis of BaFe12O19 nanoplatelets by molten salt method at various temperatures. Photocatalytic activity of synthesized nanoplatelets was studied by degrading hexahydro-1,3,5-trinitro-1,3,5 triazine (RDX) under UV and visible light. Photocatalytic degradation was less in visible region as compared to UV region. The synthesized nanoplatelets were magnetic in nature, has low bandgap (2.1 eV), easily separatable, reusable, and showed better activity than P25 TiO2.18 Bibi, I., et al., synthesized Cr and Co doped Ba1−xCoxFe12−yCryO19 nanoparticles by microemulsion method. The photocatalytic activity of synthesized nanoparticles was studied by using crystal violet dye under sunlight. The doped sample degraded 64.23% dye within 60 minutes.19 Bibi, F., et al., reported the synthesis of Nd and Cu doped Ba1−xNdxFe12−yCuyO19 nanoparticles. Methylene green was used as a model pollutant to study the photocatalytic activity of synthesized samples. Undoped and co-doped sample degraded 37.4% and 92.6% dye, respectively. The increased activity is due to the formation of oxygen vacancies which reduces the rate of recombination of electron–hole pairs.20 Doping with transition metals and rare earth metals is done to improve the catalytic activity of semiconductors. They reduce the bandgap energy, improve the light absorption ability and also increase the separation of charges.21,22 Nickel is transition metal with an abundant electron shell structure. The incorporation of Ni into the semiconductor lattice would actively modify the physical properties of semiconductors by creating impurity energy level.23 Rare earth metal ions have incompletely filled 4f and vacant 5d orbitals which often serve as a catalyst or promote catalysis.24 Among all rare earth elements, holmium has one of the highest magnetic moments. Also, holmium oxide crystallizes in cubic structure which expose highly reactive base sites (O2− and/or OH−) and Lewis-acid sites. This OH− may react with holes and generate hydroxyl radicals which improve the photocatalytic performance of semiconductors.25
MXene is a layered two-dimensional structure of transition metal, carbide or nitride. MXene have various properties such as high electrical conductivity, large surface area, wide interlayer spacing, more active sites and hydrophilicity.26,27 MXene can help in removing heavy metals, radioactive nuclides and dyes.28 In order to achieve better characteristics, MXene-based composites were also being investigated by scientists.29–31 MXene serve as a 2D platform for the preparation of composite with photoactive semiconductors. MXene has negatively charged surface due to presence of surface functional groups which enables it to adsorb metal cations by electrostatic attractions and formation of semiconductors on MXene surface. MXene enhance the properties of semiconductors by facilitating separation of charge carriers in the composites and improve their photocatalytic activity. Favourable energy structure and high electrical conductivity of MXene enable it to trap and shuttle the photoelectrons generated from semiconductors thus promoting the charge-carriers separation and the photoactivity enhancement of MXene-based composites.32,33 In current study, Ba2−xHoxSr2−yNiyFe12O22 (x = 0, 0.1 and y = 0, 0.5) have been synthesized by sol–gel method. Sol–gel method is a versatile method for synthesis of ferrites having many advantages such as high purity product is obtained at low temperature.34 It is a low-cost method and no special instruments are needed. In this method, polycrystalline particles are prepared as homogeneous mixing of metal ions took place at molecular level. By adjusting various parameters i.e., spin speed, nature and concentration of precursors, reaction time, calcination temperature etc., the properties of synthesized samples can be controlled easily.35 Another advantage of this method is that an active dopant is easily incorporated in sol during gelation step which allows the dopants to directly interact with metal ions and enhance their catalytic properties.36 The composite of Ba2−xHoxSr2−yNiyFe12O22 with MXene was synthesized by ultra-sonication method. The photocatalytic activity of these synthesized materials was studied using rhodamine B dye and pendimethalin pesticide.
2. Experimental part
2.1. Chemicals
The chemicals used in the synthesis of materials were metal nitrates such as barium nitrate [Ba(NO3)2 (≥99%)], holmium nitrate [Ho(NO3)3·5H2O (99.9%)], nickel nitrate [Ni(NO3)2·6H2O (≥98.5%)], strontium nitrate [Sr(NO3)2 (≥99%)], iron nitrate [Fe(NO3)3·9H2O (≥99.95%)], citric acid [C6H8O7·H2O (99%)], MAX powder [Ti3AlC2 (≥90%)], hydrofluoric acid [HF], aqueous ammonia [NH4OH] and deionized water. These chemicals were of analytical grade and used without further purification. All of these were brought from Sigma-Aldrich. The photocatalytic activity of samples was studied using rhodamine B dye and pendimethalin pesticide.
2.2. Synthesis of Ba2Sr2Fe12O22 and Ba1.9Ho0.1Sr1.5Ni0.5Fe12O22
Nickel nitrate, iron nitrate, barium nitrate, strontium nitrate, holmium nitrate, and citric acid were used in proper ratios to synthesize nickel and holmium substituted Ba–Sr hexagonal Y-type ferrites as given in Table 1. Samples with composition x = 0, 0.1 and y = 0, 0.5 were prepared by the sol–gel auto-combustion method by doping with different concentrations. Aqueous solutions of chemicals were prepared and stirred separately with the help of a magnetic stirrer. Then, all these separate solutions were mixed together and the resulting solution was stirred until a homogeneous solution was obtained. Ammonia solution was added dropwise to maintain pH at 7. After maintaining pH at 7, the solution was heated along with continuous stirring. After 4 hours of heating, xerogel was formed, which on further heating converted into ash. Then, ash was ground to obtain a fine powder using pestle mortar. Sintering of obtained powder was done for 6 hours at 950 °C. After sintering, again samples were ground to obtain fine powder. Fig. 1 shows the schematic diagram of synthesis of Ba2−xHoxSr2−yNiyFe12O22.
Table 1 Concentration and weight of chemicals of Ba2−xHoxSr2−yNiyFe12O22
Composition |
Barium nitrate g/5 ml |
Holmium nitrate g/5 ml |
Strontium nitrate g/5 ml |
Nickel nitrate g/5 ml |
Iron nitrate g/5 ml |
Citric acid |
x = 0 |
2.614 |
0 |
2.117 |
0 |
24.24 |
3.1521 g/15 ml |
y = 0 |
x = 0.1 |
2.483 |
0.221 |
1.587 |
0.727 |
24.24 |
5.2537 g/25 ml |
y = 0.5 |
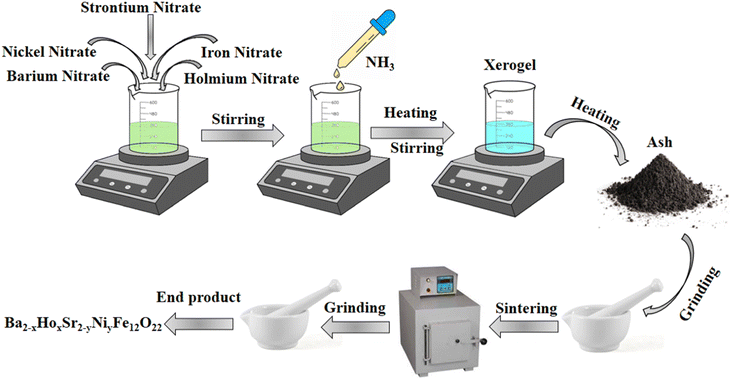 |
| Fig. 1 Schematic diagram of synthesis of Ba2−xHoxSr2−yNiyFe12O22. | |
2.3. Synthesis of MXene (Ti3C2)
MAX powder (Ti3AlC2) was used as a precursor in the synthesis of MXene (Ti3C2). 1 g of MAX powder was dissolved in 20 ml hydrofluoric acid (40%, HF). This solution was magnetically stirred for 48 hours in a Teflon vessel for the etching of aluminium. De-ionized water was added to the resulting black-coloured sample and centrifugation was done at 5500 rpm for 10 minutes. Washing of the sample was done until pH became neutral. At last, the sample was dried in the oven at 60 °C.
2.4. Synthesis of composite with MXene
Ultrasonication method was used to prepare a composite of Ba1.9Ho0.1Sr1.5Ni0.5Fe12O22 with MXene. At first, separate solutions of Ba1.9Ho0.1Sr1.5Ni0.5Fe12O22 and MXene were prepared in distilled water in ratio 9
:
1. These solutions were sonicated for 2 hours and stirred for 1 hour to prepare a homogeneous mixture. Then, both solutions were mixed and sonicated for 3 hours to obtain a homogeneous sample solution. The sample solution obtained was then dried in an oven at 80 °C and the obtained sample was ground to make a fine powder of Ba1.9Ho0.1Sr1.5Ni0.5Fe12O22@MXene composite.
2.5. Photocatalytic activity
The photocatalytic behaviour of synthesized materials was investigated by photodegrading aqueous solutions of rhodamine B dye and pendimethalin pesticide which were taken as model pollutants. This experiment was performed in two light bulbs of 200 W each. A 7 ppm solution of rhodamine B dye and 0.6 ppm solution of pendimethalin was prepared in distilled water and 500 ml of each was taken in separate beakers. 30 mg of each synthesized photocatalyst was added to these beakers and stirring was done in dark for 1 hour to achieve adsorption–desorption equilibrium. About 5 ml of sample solutions were taken from the reaction mixture. Then, the apparatus was kept in a bulb and sample solutions were obtained at regular time intervals (20 min), centrifuged and absorbance was noted using UV-vis spectrophotometer. The absorbance of both dye and pesticide was noted by the same procedure.
Rhodamine B shows absorbance peaks at approximately 553 nm. It was observed that the intensity of characteristic absorbance peaks of rhodamine B decreased and eventually faded with the time. It was noted that bare material took greater time in photodegradation as compared to the time taken by composite with MXene.5
By utilizing the following formula percentage degradation is calculated:
|
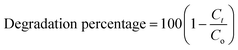 | (1) |
The starting concentration of organic dyes at time 0 min is denoted by Co, while the concentration at time t min is denoted by Ct.37
3. Characterization
Different techniques were used to determine the structural characteristics and properties of prepared materials. Shimadzu 6100AS XRD diffractometer, using Cu Kα radiation source (λ = 1.5418 Å), was used to identify the phases of synthesized samples. Shimadzu IR Affinity-IS spectrometer was used to examine Fourier transform infrared spectra in the range of 4000 cm−1 to 400 cm−1. The morphology of synthesized samples was determined by thermal emission scanning electron microscope. Using an Agilent Cary 60 spectrophotometer, UV-visible spectra of the samples was obtained.
4. Result and discussions
4.1. XRD analysis
X-ray diffraction (XRD) was used to characterize the structural characteristics of Ba2−xHoxSr2−yNiyFe12O22 (where x = 0, 0.1 and y = 0, 0.5) and the associated XRD patterns are shown in Fig. 2. Bragg's reflection peaks were observed at different 2θ values i.e., 23.105° (006), 30.43° (110), 32.99° (107), 34.28° (114), 35.56° (108), 37.19° (203), 40.45° (205), 43.24° (206), 44.63° (1011), 47.55° (1110), 49.87° (209), 55.34° (217), 56.86° (304), 58.96° (1112), 63.26° (220), 67.66° (2014). These reflection peaks are well-matched with the standard JCPDS card number (80-1198). XRD data confirmed the successful synthesis of hexagonal shaped ferrites.38 Distinct and sharp peaks indicate high crystallinity and purity of phases. Bragg's reflection peaks observed at 2θ values i.e., 25.19°, 26.25°, 27.18° in Fig. 2a and Bragg's reflection peaks at 25.46°, 26.518° and 27.55° in Fig. 2b correspond to the secondary phases of Fe2O3. Peaks at 50.29° and 61.48° in Fig. 2b correspond to HoFeO3.
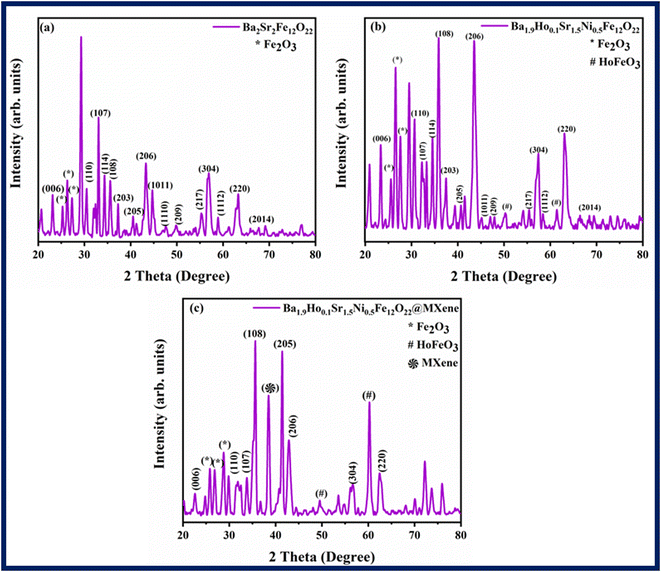 |
| Fig. 2 XRD patterns of (a) Ba2Sr2Fe12O22 (b) Ba1.9Ho0.1Sr1.5Ni0.5Fe12O22, and (c) Ba1.9Ho0.1Sr1.5Ni0.5Fe12O22@MXene. | |
Ionic radii and electronic configuration are significant variables that can have an impact on structural characteristics. The formation of the secondary phase is subsequently caused by the greater concentration of ions of rare earth elements at tetrahedral and octahedral sites. A secondary phase (impurity) formed on the surface of grain boundary as shown in the XRD patterns by “*, #” caused deformation in the lattice inside the grain regions. This deformation in lattice may be caused by internal stress brought on by the different thermal expansion coefficients of the bulk material and the inter-granular material, or it might be caused by a mismatch in the lattice at the boundary/grain interface.39 These secondary phase peaks (Fe2O3) are well-matched to the JCDPS card number (89-0599). The Ba1.9Ho0.1Sr1.5Ni0.5Fe12O22@MXene spectra in Fig. 2c showed a prominent characteristic peak of MXene at 2θ = 38° (104) which confirmed the presence of MXene in the Ba1.9Ho0.1Sr1.5Ni0.5Fe12O22@MXene composite.40
Ba2Sr2Fe12O22 and Ba1.9Ho0.1Sr1.5Ni0.5Fe12O22 crystal size was determined Debye and Scherrer formula which is as follows.41–43
|
 | (2) |
where
D is the crystallite size,
λ is the X-ray wavelength,
β stands for full width at half maximum (FWHM) and
θ is Bragg's diffraction angle. Ba
2Sr
2Fe
12O
22, and Ba
1.9Ho
0.1Sr
1.5Ni
0.5Fe
12O
22 have average crystallite sizes of 19.2645 nm, and 14.46 nm, respectively (
Table 2).
Table 2 XRD parameters of Ba2Sr2Fe12O22 and Ba1.9Ho0.1Sr1.5Ni0.5Fe12O22
|
Ba2Sr2Fe12O22 |
Ba1.9Ho0.1Sr1.5Ni0.5Fe12O22 |
Crystallite size |
19.2645 nm |
14.46 nm |
Dislocation density |
0.00269 nm−2 |
0.00478 nm−2 |
Space group |
P63/mmc |
— |
Crystal system |
Hexagonal |
Hexagonal |
Cell constant |
a = b = 5.849 (Å) |
a = b = 5.843 (Å) |
c = 23.38 (Å) |
c = 23.21 (Å) |
Volume (Å3) |
692.66 Å3 |
686.24 Å3 |
The dislocation density of prepared samples was found by following formula:
|
 | (3) |
Here,
δ is the dislocation density and
D is the crystallite size of synthesized materials.
The lattice constants of hexagonal cell was calculated using cell software. The volume of hexagonal crystal system was calculated by the following equation:
|
Volume = abc(1 − cos2 α − cos2 β − cos2 γ + 2 cos αβγ)1/2
| (4) |
(where
α =
β = 90° and
γ = 120° for hexagonal system).
4.2. FT-IR
FT-IR spectra of all synthesized samples i.e., Ba2Sr2Fe12O22, Ba1.9Ho0.1Sr1.5Ni0.5Fe12O22, and Ba1.9Ho0.1Sr1.5Ni0.5Fe12O22@MXene are shown in Fig. 3 in the range of 400 cm−1 to 4000 cm−1. FT-IR analysis was done to analyse structural information and functional group of synthesized materials. The frequency band that appeared at 610 cm−1 is due to intrinsic stretching vibrations of tetrahedral and octahedral site of barium hexaferrite structure.44 Ferrite formation is confirmed by the absorption of infra-red radiation in this region.45,46 The variation in low and high frequency bands indicated that holmium and nickel were incorporated into the matrix of barium–strontium ferrites.47,48 The relative peaks appeared at 1634 cm−1 and 3450 cm−1 are due to stretching vibrations of water molecules.49 The decrease in intensity of peaks in the FTIR spectrum of doped and composite samples confirmed the synthesis of Ba1.9Ho0.1Sr1.5Ni0.5Fe12O22, and Ba1.9Ho0.1Sr1.5Ni0.5Fe12O22@MXene samples.
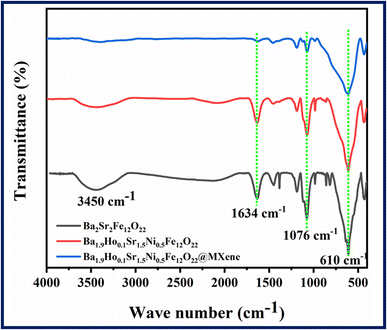 |
| Fig. 3 FTIR spectra of Ba2Sr2Fe12O22, Ba1.9Ho0.1Sr1.5Ni0.5Fe12O22, and Ba1.9Ho0.1Sr1.5Ni0.5Fe12O22@MXene. | |
4.3. Scanning electron microscopy
Scanning electron microscopy was performed to evaluate the morphology of synthesized samples. Fig. 4a–e showed SEM images of prepared Ba2Sr2Fe12O22, Ba1.9Ho0.1Sr1.5Ni0.5Fe12O22, Ba1.9Ho0.1Sr1.5Ni0.5Fe12O22/MXene, and bare MXene, respectively. The SEM images were taken at different magnifications. The SEM images (Fig. 4a) revealed that the synthesized Ba2Sr2Fe12O22 structures have flakes like morphology. In the case of Ba1.9Ho0.1Sr1.5Ni0.5Fe12O22, no significant alteration in the morphology was observed. The SEM image of Ba1.9Ho0.1Sr1.5Ni0.5Fe12O22/MXene [Fig. 4c and d] showed that synthesized particles are intercalated in MXene sheets as both flakes and MXene sheets were observed. Accordion like structure comprised of 2D sheets of MXene was observed in SEM image of pure MXene in Fig. 4e. This confirmed the successful synthesis of MXene. The average grain size of Ba2Sr2Fe12O22 and Ba1.9Ho0.1Sr1.5Ni0.5Fe12O22, was calculated using ImageJ software and found to be approximately 26.8 μm and 24.7 μm, respectively.
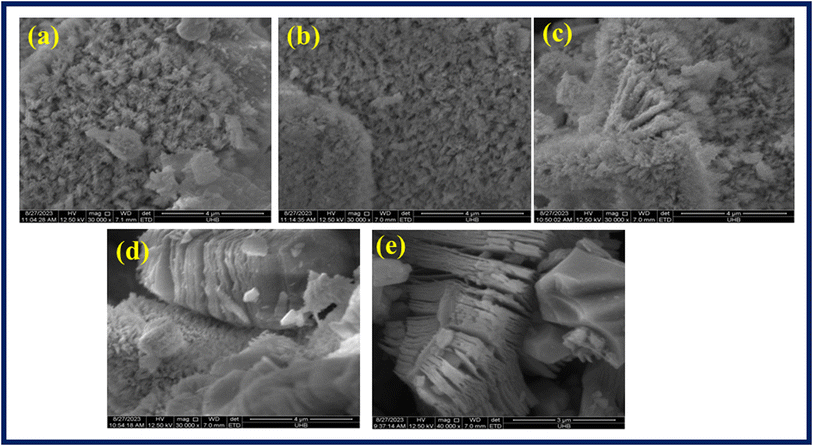 |
| Fig. 4 SEM images of (a) Ba2Sr2Fe12O22 (b) Ba1.9Ho0.1Sr1.5Ni0.5Fe12O22, (c and d) Ba1.9Ho0.1Sr1.5Ni0.5Fe12O22@MXene and (e) MXene. | |
4.4. UV-vis analysis
UV-vis spectra of Ba2Sr2Fe12O22 and Ba1.9Ho0.1Sr1.5Ni0.5Fe12O22 was measured in the range of 200 nm to 800 nm, and the results are displayed in Fig. 5.
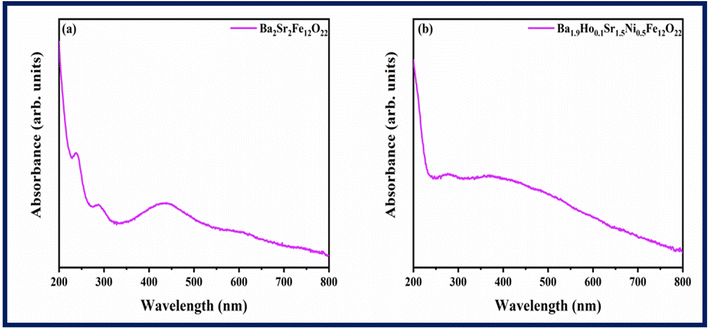 |
| Fig. 5 Absorption spectra of (a) Ba2Sr2Fe12O22 (b) Ba1.9Ho0.1Sr1.5Ni0.5Fe12O22. | |
The efficiency of synthesized samples was analysed by finding their bandgap energy. Bandgap significantly influences the generation of electron–hole pairs in conduction and valence band. Tauc plots (Fig. 6) are used to calculate the band gap energy using eqn (5) below.
in this formula,
α stands for the absorption coefficient,
ν for the frequency of the light being employed, ℏ for Planck's constant,
n stands for either direct (2) or indirect bandgap (1/2), and
β for the proportionality constant. According to
Fig. 6, the estimated optical band gaps (
Eg) for Ba
2Sr
2Fe
12O
22 and Ba
1.9Ho
0.1Sr
1.5Ni
0.5Fe
12O
22 are 1.7 eV and 1.129 eV, respectively. It shows that the band-gap of Ba
2Sr
2Fe
12O
22 is reduced by doping of holmium and nickel. Additionally, the Ba
1.9Ho
0.1Sr
1.5Ni
0.5Fe
12O
22 band-gap decreases to a lower value, confirming the electronic interaction of holmium and nickel in Ba
2Sr
2Fe
12O
22.
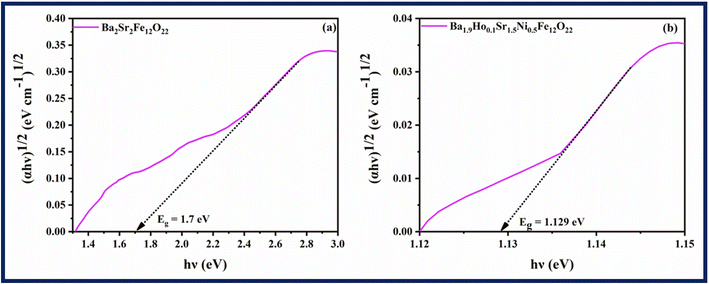 |
| Fig. 6 Tauc plots of (a) Ba2Sr2Fe12O22 (b) Ba1.9Ho0.1Sr1.5Ni0.5Fe12O22. | |
5. Photocatalysis
The ability of photocatalysts to degrade rhodamine B and pendimethalin was assessed under the influence of visible light. Fig. 7 and 8 showed the spectra of photodegradation of rhodamine B and pendimethalin, respectively. Photocatalysis is based on three phases. Photons with energies higher than the synthesized samples' bandgap energy are absorbed in the first step. In the following step, electrons and holes are produced and transferred. On the photocatalyst surface, reduction and oxidation take place in the third stage. After various reaction times, the maximum absorption of dye and pesticide gradually diminished, as seen in Fig. 7 and 8. Ba2Sr2Fe12O22 has a very low degradation efficiency for RhB under the influence of visible light. However, as shown in Fig. 7c and 8c, the proportion of RhB and pendimethalin that was photo-catalytically degraded by the composite with MXene was around 78.88% and 75.59% in 140 minutes, respectively. The surface area of the photocatalyst increases with the composite formation with MXene, that's why maximum absorbance occurred and the rate of electron–hole pair recombination decreased.
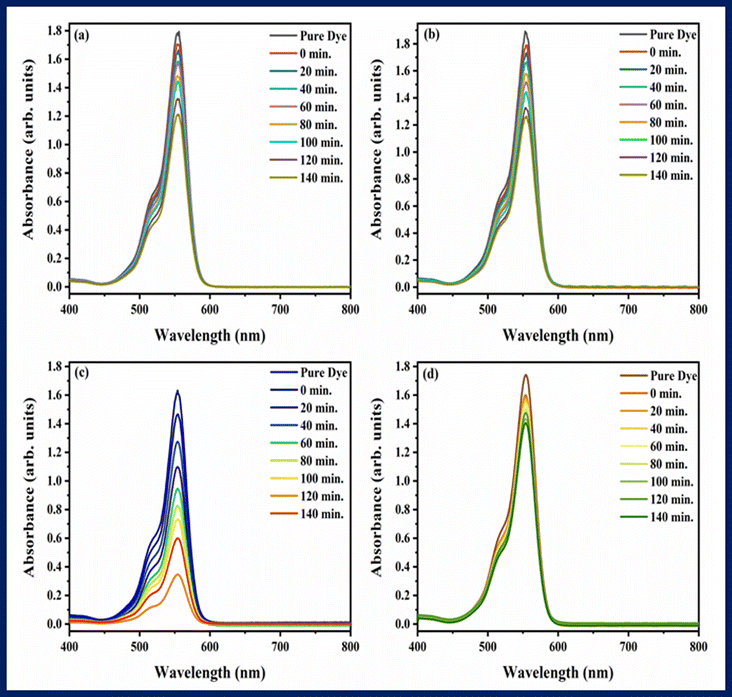 |
| Fig. 7 Photodegradation spectra of rhodamine B using (a) Ba2Sr2Fe12O22 (b) Ba1.9Ho0.1Sr1.5Ni0.5Fe12O22, (c) Ba1.9Ho0.1Sr1.5Ni0.5Fe12O22@MXene, and (d) MXene. | |
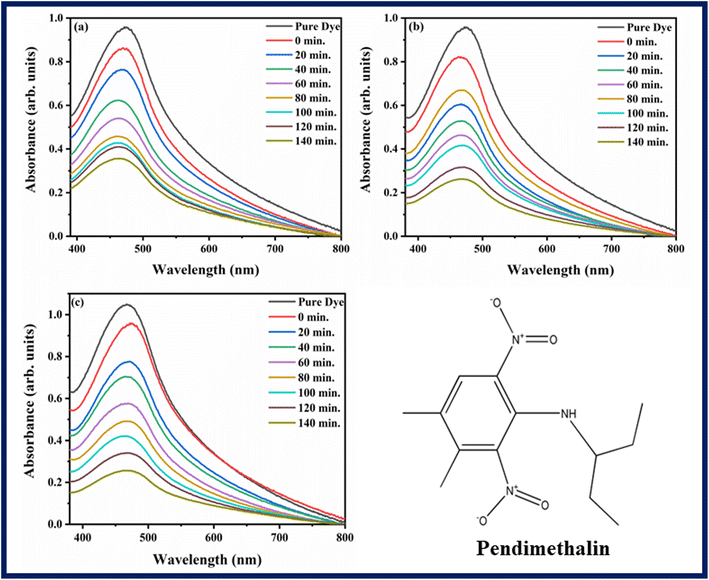 |
| Fig. 8 Photodegradation spectra of pendimethalin using (a) Ba2Sr2Fe12O22, (b) Ba1.9Ho0.1Sr1.5Ni0.5Fe12O22, and (c) Ba1.9Ho0.1Sr1.5Ni0.5Fe12O22@MXene. | |
Using pseudo-first-order kinetic eqn (6), the rate of photodegradation of RhB dye and pendimethalin was investigated.
|
 | (6) |
in this equation,
K and
t stand for pseudo-first-order reaction rate constant and irradiation time, respectively.
Fig. 9 and
10 showed the kinetics graphs for degradation of rhodamine B and pendimethalin, respectively. Slope of the line of

represents the rate constant. It was discovered that the kinetic rate constant of the Ba
1.9Ho
0.1Sr
1.5Ni
0.5Fe
12O
22 composite is greater than that of Ba
2Sr
2Fe
12O
22. The value of rate constant was also found using
eqn (4) as shown in
Fig. 9d and
10d. Using MXene, Ba
2Sr
2Fe
12O
22, Ba
1.9Ho
0.1Sr
1.5Ni
0.5Fe
12O
22 and Ba
1.9Ho
0.1Sr
1.5Ni
0.5Fe
12O
22@MXene the rate constant for degradation of rhodamine B was 0.00108 min
−1, 0.00223 min
−1, 0.00455 min
−1, and 0.00737 min
−1 respectively and the rate constant for the degradation of pendimethalin was 0.00643 min
−1, 0.00779 min
−1 and 0.0086 min
−1, respectively. It showed that Ba
1.9Ho
0.1Sr
1.5Ni
0.5Fe
12O
22@MXene has highest rate of degradation of rhodamine B and pendimethalin. Percentage degradation of rhodamine B (
Fig. 9c) in bulb using pure MXene, Ba
2Sr
2Fe
12O
22, Ba
1.9Ho
0.1Sr
1.5Ni
0.5Fe
12O
22 and Ba
1.9Ho
0.1Sr
1.5Ni
0.5Fe
12O
22@MXene was 16.84%, 41.56%, 55.86%, and 78.88%, respectively. Percentage degradation of pendimethalin (
Fig. 10c) in bulb using Ba
2Sr
2Fe
12O
22, Ba
1.9Ho
0.1Sr
1.5Ni
0.5Fe
12O
22, and Ba
1.9Ho
0.1Sr
1.5Ni
0.5Fe
12O
22@MXene was 53.389%, 62.83% and 75.59%, respectively.
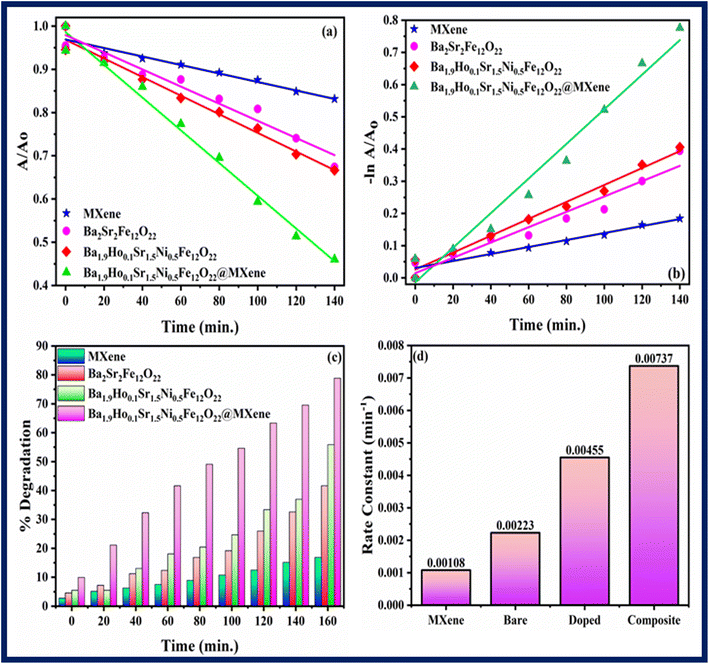 |
| Fig. 9 Kinetic study of all synthesized samples using rhodamine B (a) kinetic rate (b) linear kinetics (c) percentage degradation (d) rate constant. | |
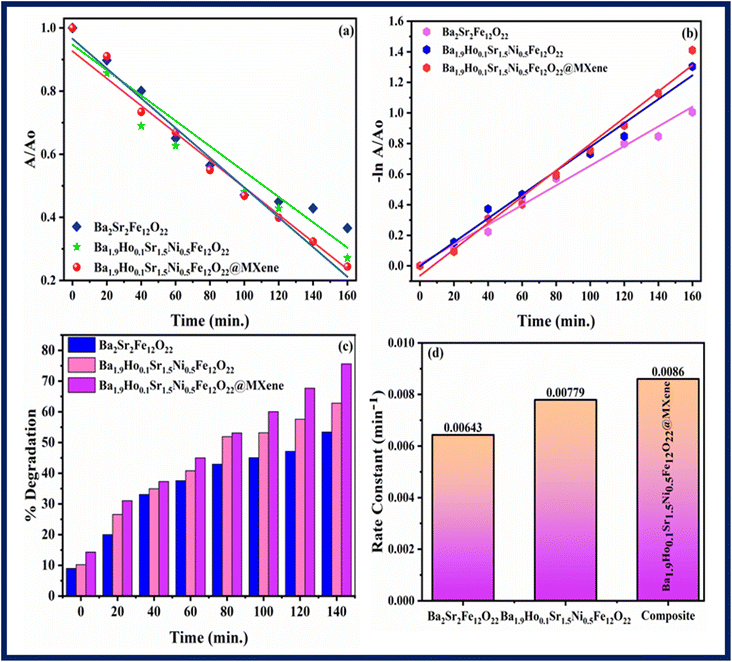 |
| Fig. 10 Kinetic study of all synthesized samples using pendimethalin (a) kinetic rate (b) linear kinetics (c) percentage degradation (d) rate constant. | |
For investigation of degradation mechanism for photocatalytic activity, following equations are used for calculation of valence band potential (EVB) and conduction band potential (ECB):50
Here
X is the absolute electronegativity value,
Ee is the free electron energy (4.5 eV relative to NHE) and
Eg is the bandgap energy of synthesized semiconductor. The calculated values of
EVB and
ECB are −2.5765 eV and −3.7055 eV, respectively.
51
To facilitate the reduction and oxidation processes, photocatalysts use light energy. When light is illuminated on a photocatalyst, the valence electrons of the photocatalyst are excited into conduction band. As a result, electron–hole pairs are created, which are then in charge of causing reduction and oxidation processes. When holes oxidize H2O molecules and OH− ions during photocatalytic activity in an aqueous phase and adsorbed oxygen is reduced by electrons, OH˙ and O2˙− radicals are produced. Compared to the commonly utilized oxidants H2O2 and O3, they are good oxidants. Complete oxidation occurs as a result of OH oxidants' repeated attacks on organic pollutants. There are two ways that OH˙ is produced. First is the reduction of oxygen into O2− oxygen, which is then changed into ˙OOH by attack of hole. Second, by oxidizing OH, OH˙ is produced.52,53 Rhodamine B dye is degraded by these radicals into harmless products like CO2 and H2O (Fig. 11).
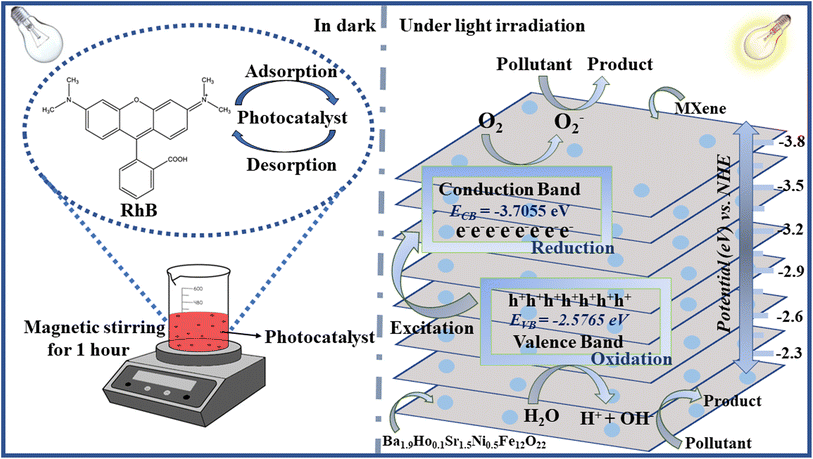 |
| Fig. 11 Schematic diagram of photodegradation using synthesized samples. | |
The following is a presentation of the suggested mechanism of the synthesized samples:
|
Composite + hν → composite (e−/h+)@MXene
| (9) |
|
Composite (e−/h+)@MXene → composite (h+)@MXene (e−)
| (10) |
|
MXene (e−) + O2 → O22−* + MXene
| (11) |
|
O22−* + RhB → degradation products
| (12) |
|
Composite (h+) + OH− → composite + OH*
| (13) |
|
OH* + RhB → degradation products
| (14) |
|
MXene (h+) + RhB → degradation products
| (15) |
There are two potential causes for the enhanced photoactivity of synthesized composite materials. Doping of holmium and nickel in Ba2Sr2Fe12O22 and composite with MXene decreases agglomeration and increases specific surface area. A greater specific surface area often yields a better harvesting efficiency of light. MXene sheets trap electrons and increase the charge carrier separation. Second, distinct redox energy levels of doped semiconductor made it easier for influentially separated photogenerated e−/h+ pairs to form, lengthening the life of charge carriers. According to the aforementioned findings, bare Ba2Sr2Fe12O22 is less active than Ba1.9Ho0.1Sr1.5Ni0.5Fe12O22@MXene composite.54
6. Scavenging experiment
Scavenging experiment was performed to investigate the main active species which are responsible for photodegradation of rhodamine B. Scavenging experiment was also carried out in similar conditions and procedure as followed in photocatalysis. Silver nitrate, ammonium oxalate, and tertiary butanol were taken as scavengers for quenching electrons (e−), holes (h+) and hydroxyl radicals (OH*), respectively.55 Fig. 12–14 show results of scavenging experiment using Ba2Sr2Fe12O22, Ba1.9Ho0.1Sr1.5Ni0.5Fe12O22, and Ba1.9Ho0.1Sr1.5Ni0.5Fe12O22@MXene. Fig. 12–14(a) and (b) showed kinetics graphs of scavenging experiment and Fig. 12–14(c) and (d) showed the rate constant values and variation in % photocatalytic degradation of RhB by all three samples using these scavengers. The results of this experiment showed that electrons and holes were the main active species involved in photodegradation process while hydroxyl radicals showed the least contribution in photocatalytic activity of RhB by using synthesized samples. The order of active species involved in photocatalytic activity was:
Electrons > holes > hydroxyl radicals |
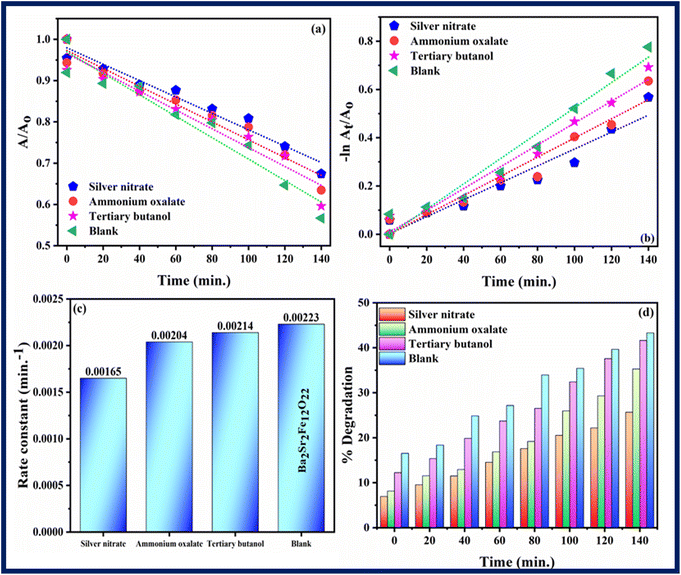 |
| Fig. 12 (a and b) Kinetics plots, (c) rate constants plot (d) % degradation related to RhB dye degradation using Ba2Sr2Fe12O22 using different scavengers. | |
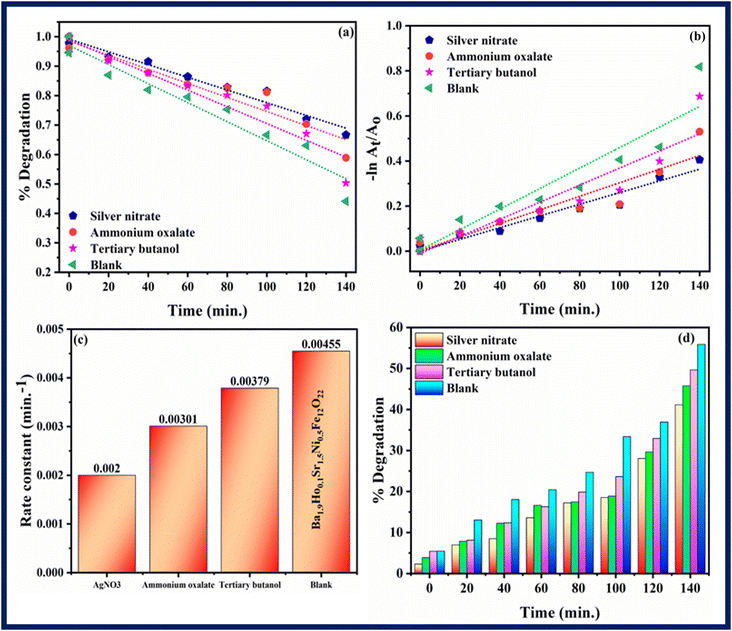 |
| Fig. 13 (a and b) Kinetics plots, (c) rate constants plot (d) % degradation related to RhB dye degradation using Ba1.9Ho0.1Sr1.5Ni0.5Fe12O22 using different scavengers. | |
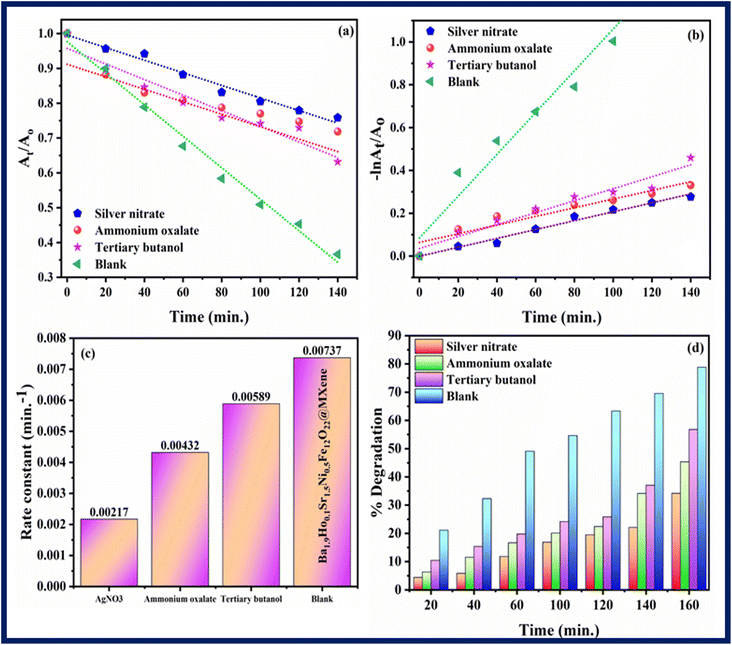 |
| Fig. 14 (a and b) Kinetics plots, (c) rate constants plot (d) % degradation related to RhB dye degradation using Ba1.9Ho0.1Sr1.5Ni0.5Fe12O22@MXene using different scavengers. | |
7. Conclusion
Ba2−xHoxSr2−yNiyFe12O22 (x = 0, 0.1 and y = 0, 0.5) and composite with MXene was successfully fabricated by sol–gel and ultra-sonication method. Various properties such as crystallite size, volume, band gap etc., were analysed using X-ray diffraction (XRD), Fourier transform infrared spectroscopy (FT-IR), Scanning Electron Microscopy (SEM), and UV-visible spectroscopy. Ba2Sr2Fe12O22 and Ba1.9Ho0.1Sr1.5Ni0.5Fe12O22 have average crystallite sizes of 19.26 nm and 14.46 nm, respectively. The average grain size of Ba2Sr2Fe12O22 and Ba1.9Ho0.1Sr1.5Ni0.5Fe12O22, was calculated using ImageJ software and found to be approximately 26.8 μm and 24.7 μm, respectively. Ba2Sr2Fe12O22 and Ba1.9Ho0.1Sr1.5Ni0.5Fe12O22 have indirect band gap 1.7 eV and 1.129 eV, respectively. Photocatalytic behaviour of synthesized samples was studied using rhodamine B dye and pendimethalin pesticide as model pollutants. Maximum photodegradation of dye and pesticide was observed i.e., 78.88% and 75.59%, respectively under similar conditions using Ba1.9Ho0.1Sr1.5Ni0.5Fe12O22@MXene. Scavenging experiment revealed that electrons and holes were the main active species taking part in photodegradation process. Ba2−xHoxSr2−yNiyFe12O22@MXene composite can be used as a potential applicant in cleaning environment and removing pollutants.
Conflicts of interest
There are no conflicts of interest to declare for our manuscript being submitted to your journal.
Acknowledgements
The authors express their sincere gratitude to the Deanship of Scientific Research at the Islamic University of Madinah for the support provided to the Post-Publishing Program. Authors are also thankful to the Division of Physical Chemistry, Institute of Chemistry, BJ Campus, The Islamia University of Bahawalpur (Pakistan) and GCU-Faisalabad (Pakistan).
References
- W. Ouyang, et al., Optimization of typical diffuse herbicide pollution control by soil amendment configurations under four levels of rainfall intensities, J. Environ. Manage., 2016, 175, 1–8 CrossRef CAS PubMed.
- W. Gong, et al., Abiotic reduction of trifluralin and pendimethalin by sulfides in black-carbon-amended coastal sediments, J. Hazard. Mater., 2016, 310, 125–134 CrossRef CAS PubMed.
- J. V. Rohit and S. K. Kailasa, Simple and selective detection of pendimethalin herbicide in water and food samples based on the aggregation of ractopamine-dithiocarbamate functionalized gold nanoparticles, Sens. Actuators, B, 2017, 245, 541–550 CrossRef CAS.
- A. Shahnazi, M. R. Nabid and R. Sedghi, Synthesis of surface molecularly imprinted poly-o-phenylenediamine/TiO2/carbon nanodots with a highly enhanced selective photocatalytic degradation of pendimethalin herbicide under visible light, React. Funct. Polym., 2020, 151, 104580 CrossRef CAS.
- A. K. Al-Buriahi, et al., Elimination of rhodamine B from textile wastewater using nanoparticle photocatalysts: a review for sustainable approaches, Chemosphere, 2022, 287, 132162 CrossRef CAS PubMed.
- S.-M. Lam, et al., Sunlight responsive WO3/ZnO nanorods for photocatalytic degradation and mineralization of chlorinated phenoxyacetic acid herbicides in water, J. Colloid Interface Sci., 2015, 450, 34–44 CrossRef CAS PubMed.
- H. M. Abo-Dief, et al., Ternary metal oxide WO3 NiO ZnO nanoparticles and their composite with CNTs for organic dye photocatalytic degradation, Ceram. Int., 2022, 48(15), 22269–22277 CrossRef CAS.
- R. Sedghi, et al., High-performance visible light-driven Ni-ZnO/rGO/nylon-6 & Ni-ZnO/rGO/nylon-6/Ag nanofiber webs for degrading dye pollutant and study their antibacterial properties, J. Alloys Compd., 2017, 729, 921–928 CrossRef CAS.
- K. Okumura, et al., Magnetism and magnetoelectricity of a U-type hexaferrite Sr 4 Co 2 Fe 36 O 60, Appl. Phys. Lett., 2011, 98(21), 212504 CrossRef.
- R. Srivastava and B. C. Yadav, Ferrite materials: introduction, synthesis techniques, and applications as sensors, Int. J. Green Nanotechnol., 2012, 4(2), 141–154 CrossRef CAS.
- R. Kausar, et al., Investigation into the structural and magnetic features of nickel doped U-type hexaferrites prepared through sol–gel method, J. Magn. Magn. Mater., 2022, 549, 169054 CrossRef CAS.
- B. I. Kharisov, H. R. Dias and O. V. Kharissova, Mini-review: ferrite nanoparticles in the catalysis, Arabian J. Chem., 2019, 12(7), 1234–1246 CrossRef CAS.
- D. Lisjak, D. Makovec and M. Drofenik, Formation of U-type hexaferrites, J. Mater. Res., 2004, 19(8), 2462–2470 CrossRef CAS.
- M. Chandra Dimri, et al., Magnetic properties and 57Fe NMR studies of U-type hexaferrites, J. Magn. Magn. Mater., 2011, 323(16), 2210–2213 CrossRef CAS.
- R. C. Pullar, Hexagonal ferrites: a review of the synthesis, properties and applications of hexaferrite ceramics, Prog. Mater. Sci., 2012, 57(7), 1191–1334 CrossRef CAS.
- R. Kausar, et al., Investigation into the structural and magnetic features of nickel doped U-type hexaferrites prepared through sol–gel method, J. Magn. Magn. Mater., 2022, 549, 169054 CrossRef CAS.
- P. Roonasi and M. Mazinani, Synthesis and application of barium ferrite/activated carbon composite as an effective solar photocatalyst for discoloration of organic dye contaminants in wastewater, J. Environ. Chem. Eng., 2017, 5(4), 3822–3827 CrossRef CAS.
- S. S. Raut, et al., Enhanced photocatalytic activity of magnetic BaFe12O19 nanoplatelets than TiO2 with emphasis on reaction kinetics, mechanism, and reusability, Ind. Eng. Chem. Res., 2018, 57(48), 16192–16200 CrossRef CAS.
- I. Bibi, et al., Effect of doping on dielectric and optical properties of barium hexaferrite: photocatalytic performance under solar light irradiation, Ceram. Int., 2021, 47(22), 31518–31526 CrossRef CAS.
- F. Bibi, et al., Evaluation of structural, dielectric, magnetic and photocatalytic properties of Nd and Cu co-doped barium hexaferrite, Ceram. Int., 2021, 47(21), 30911–30921 CrossRef CAS.
- S. Anandan, et al., Photocatalytic degradation of 2,4,6-trichlorophenol using lanthanum doped ZnO in aqueous suspension, Catal. Commun., 2007, 8(9), 1377–1382 CrossRef CAS.
- C. V. Reddy, et al., Ni-dopant concentration effect of ZrO2 photocatalyst on photoelectrochemical water splitting and efficient removal of toxic organic pollutants, Sep. Purif. Technol., 2020, 252, 117352 CrossRef CAS.
- M. Shaban, et al., Ni-doped and Ni/Cr co-doped TiO2 nanotubes for enhancement of photocatalytic degradation of methylene blue, J. Colloid Interface Sci., 2019, 555, 31–41 CrossRef CAS PubMed.
- X. Liu, et al., Effect of holmium doping on
the structure and photocatalytic behavior of TiO2-based nanosheets, J. Mater. Sci., 2014, 49(23), 8063–8073 CrossRef CAS.
- J.-w. Shi, J.-t. Zheng and P. Wu, Preparation, characterization and photocatalytic activities of holmium-doped titanium dioxide nanoparticles, J. Hazard. Mater., 2009, 161(1), 416–422 CrossRef CAS PubMed.
- M. Minakshi, et al., New insights into the electrochemistry of magnesium molybdate hierarchical architectures for high performance sodium devices, Nanoscale, 2018, 10(27), 13277–13288 RSC.
- A. Ihsan, et al., A strategy to boost the electrochemical properties of Ag–Fe2O3 with intercalation of MXene hydrogel, Ceram. Int., 2023 DOI:10.1016/j.ceramint.2023.09.091.
- S. Munir, et al., Exploring the influence of critical parameters for the effective synthesis of high-quality 2D MXene, ACS Omega, 2020, 5(41), 26845–26854 CrossRef CAS PubMed.
- A.-Z. Warsi, et al., Synthesis, Characterization, Photocatalysis, and Antibacterial Study of WO3, MXene and WO3/MXene Nanocomposite, Nanomaterials, 2022, 12(4), 713 CrossRef CAS PubMed.
- S. K. Hwang, et al., MXene: an emerging two-dimensional layered material for removal of radioactive pollutants, Chem. Eng. J., 2020, 397, 125428 CrossRef CAS.
- M. Naguib, et al., 25th anniversary article: MXenes: a new family of two-dimensional materials, Adv. Mater., 2014, 26(7), 992–1005 CrossRef CAS PubMed.
- A. Hojjati-Najafabadi, et al., Magnetic-MXene-based nanocomposites for water and wastewater treatment: a review, J. Water Process Eng., 2022, 47, 102696 CrossRef.
- I. A. Alsafari, et al., Synthesis, characterization, photocatalytic and antibacterial properties of copper Ferrite/MXene (CuFe2O4/Ti3C2) nanohybrids, Ceram. Int., 2021, 47(20), 28874–28883 CrossRef CAS.
- D. Bokov, et al., Nanomaterial by Sol-Gel Method: synthesis and Application, Adv. Mater. Sci. Eng., 2021, 2021, 5102014 Search PubMed.
- S. M. Reda, Synthesis of ZnO and Fe2O3 nanoparticles by sol–gel method and their application in dye-sensitized solar cells, Mater. Sci. Semicond. Process., 2010, 13(5), 417–425 CrossRef CAS.
- A. Zaleska, Doped-TiO2: a review, Recent Pat. Eng., 2008, 2(3), 157–164 CrossRef CAS.
- A. Rasheed, et al., ZrxCo0. 8− xNi0. 2− xFe2O4-graphene nanocomposite for enhanced structural, dielectric and visible light photocatalytic applications, Ceram. Int., 2016, 42(14), 15747–15755 CrossRef CAS.
- I. Desai, et al., Synthesis and characterization of magnetic manganese ferrites, Mater. Sci. Energy Technol., 2019, 2(2), 150–160 Search PubMed.
- D. Ravinder, Far-infrared spectral studies of mixed lithium–zinc ferrites, Mater. Lett., 1999, 40(5), 205–208 CrossRef CAS.
- M. A. Iqbal, et al., Ti3C2-MXene/Bismuth Ferrite Nanohybrids for Efficient Degradation of Organic Dyes and Colorless Pollutants, ACS Omega, 2019, 4(24), 20530–20539 CrossRef CAS PubMed.
- M. Aadil, et al., Superior electrochemical activity of α-Fe2O3/rGO nanocomposite for advance energy storage devices, J. Alloys Compd., 2016, 689, 648–654 CrossRef CAS.
- J. Song, et al., Microwave electromagnetic and absorbing properties of Dy3+ doped MnZn ferrites, J. Rare Earths, 2010, 28(3), 451–455 CrossRef CAS.
- M. Afaq, et al., Large-scale sonochemical fabrication of a Co 3 O 4–CoFe 2 O 4@ MWCNT bifunctional electrocatalyst for enhanced OER/HER performances, RSC Adv., 2023, 13(28), 19046–19057 RSC.
- K. Hussain, et al., Study of structural, optical, electrical and magnetic properties of Cu2+doped Zn0.4Co0.6-xCe0.1Fe1.9O4 spinel ferrites, Phys. B, 2020, 584, 412078 CrossRef CAS.
- M. Hashim, et al., Manganese ferrite prepared using reverse micelle process: structural and magnetic properties characterization, J. Alloys Compd., 2015, 642, 70–77 CrossRef CAS.
- K. Qian, et al., The influence of Nd substitution in Ni–Zn ferrites for the improved microwave absorption properties, Ceram. Int., 2020, 46(1), 227–235 CrossRef.
- S. Debnath, et al., X-ray diffraction analysis for the determination of elastic properties of zinc-doped manganese spinel ferrite nanocrystals (Mn0.75Zn0.25Fe2O4), along with the determination of ionic radii, bond lengths, and hopping lengths, J. Phys. Chem. Solids, 2019, 134, 105–114 CrossRef CAS.
- B. Aslibeiki, Nanostructural, magnetic and electrical properties of Ag doped Mn-ferrite nanoparticles, Curr. Appl. Phys., 2014, 14(12), 1659–1664 CrossRef.
- A. Farooq, et al., Fabrication of Ag-doped magnesium aluminate/rGO composite: a highly efficient photocatalyst for visible light-driven photodegradation of crystal violet and phenol, Phys. B, 2023, 650, 414508 CrossRef CAS.
- A. Irshad, et al., Ag-doped FeCo2O4 nanoparticles and their composite with flat 2D reduced graphene oxide sheets for photocatalytic degradation of colored and colorless compounds, FlatChem, 2022, 31, 100325 CrossRef CAS.
- K. Khan, et al., Structural, electrical, and photocatalytic properties of Y-type hexaferrite/carbon dot composite, J. Mater. Sci.: Mater. Electron., 2023, 34(25), 1793 CrossRef CAS.
- H. M. Abo-Dief, et al., Ternary metal oxide WO3.NiO.ZnO nanoparticles and their composite with CNTs for organic dye photocatalytic degradation, Ceram. Int., 2022, 48(15), 22228–22236 CrossRef.
- A. Ihsan, et al., NiFe2O4/ZnO nanoparticles and its composite with flat 2D rGO sheets for efficient degradation of colored and colorless effluents photocatalytically, Opt. Mater., 2022, 134, 113213 CrossRef CAS.
- S. H. U. Din, et al., Ag-doped nickel ferrites and their composite with rGO: synthesis, characterization, and solar light induced degradation of coloured and colourless effluents, Ceram. Int., 2022, 48(11), 15629–15639 CrossRef CAS.
- X. Chen, et al., Preparation of ZnO Photocatalyst for the Efficient and Rapid Photocatalytic Degradation of Azo Dyes, Nanoscale Res. Lett., 2017, 12(1), 143 CrossRef PubMed.
|
This journal is © The Royal Society of Chemistry 2023 |
Click here to see how this site uses Cookies. View our privacy policy here.