DOI:
10.1039/D3RA06767J
(Paper)
RSC Adv., 2023,
13, 33644-33653
Experimental study of CO2 capture by nanoparticle-enhanced 2-amino-2-methyl-1-propanol aqueous solution
Received
5th October 2023
, Accepted 10th November 2023
First published on 16th November 2023
Abstract
2-Amino-2-methyl-1-propanol (AMP) is often used as a moderator to enhance the CO2 capture capacity of absorbents due to its unique spatial site resistance structure, and relatively few studies have been conducted on the enhancement of AMP aqueous solutions by nanoparticles for CO2 capture. In order to investigate the effect of nanoparticles on the CO2 capture performance of AMP aqueous solution, different nanofluids were formulated in this paper using a two-step method, and a bubbling reactor and an oil bath were used as the experimental setup for absorption/desorption, and through comparative experiments, it was found that the type of nanoparticles, the solid content, and the different parameters have great influences on the CO2 absorption load and desorption rate. The experimental results show that the addition of TiO2 nanoparticles to the AMP base solution can accelerate the absorption–desorption mass transfer rate of CO2, and there exists an optimal solid content of 1 g L−1 (±1.0%, ±2.5%); after multiple absorption–desorption experiments, good cycling performance can still be achieved. The experimental results of the nanofluid-promoted mass transfer mechanism are also illustrated and analyzed in this paper.
1 Introduction
CO2 has become the most major greenhouse gas with the amount of CO2 in the atmosphere increasing by about 25% since the industrial revolution.1 In particular, the burning of fossil fuels such as oil and coal in industrial production accumulates large quantities of CO2 and other gases, and these greenhouse gases not only cause a series of ecological and environmental problems, but also result in huge economic losses. Nowadays, scientific and technological developments have made it possible to capture, utilize and store CO2 from industrial exhaust gases or directly from the air.2,3 Monoethanolamine (MEA) is usually the most widely used chemical absorbent for industrial applications, but its regeneration energy consumption is as high as 3.2–4.0 GJ ton−1CO2, and such a high renewable energy consumption increases the cost of CO2 capture, so there are obvious limitations for large-scale commercial applications.3,4 Space-site-resistant amines are often used as modulators and are characterized by bulky groups adjacent to the amino group. Due to the influence of space-site resistance, they are prone to form unstable carbamates when reacting with CO2 and decompose easily in carbonates,5 and currently widely studied is 2-amino-2-methyl-1-propanol (AMP). Wai et al.6 showed that AMP aqueous solutions have a high capacity for CO2 absorption, good thermal stability, and the existence of the site resistance effect makes AMP highly degradable and have low regeneration energy. Han et al.7 compared the CO2 absorption capacity of AMP with MEA and found that the absorption rate of AMP was slightly lower than MEA. In addition, many studies have been reported on the physicochemical and thermodynamic properties of AMP such as corrosion rate, density, viscosity and regeneration performance in mixed amine solvents.8,9
Recently, research on the use of nanofluids to enhance mass transfer and reduce energy consumption has been actively underway. According to previous studies, it has been found that nanoparticles have a high specific surface area, and when small amounts are introduced into the base solution of organic amine absorbents by physical or chemical methods,10,11 absorbents are acquired with enhanced CO2 absorption performance and other advantages (e.g., improved solvent stability and reduced solvent vapor pressure, etc.).12 Meanwhile, nanoparticles can reduce the mass transfer resistance of gas–liquid two-phase and accelerate the mass transfer rate, thereby reducing energy consumption.13,14 TiO2 is a nanoparticle with high CO2 adsorption capacity, which was found to be due to the presence of oxygen vacancies in TiO2 nanoparticles, which introduce additional adsorption sites.22,37 Fang et al.49 researched the effect of nanoparticles on CO2 absorption in ammonia and found that TiO2 nanoparticles can enhance the gas–liquid mass transfer process of chemical reactions. Kim et al.15 conducted CO2 absorption experiments through utilizing SiO2 nanoparticles in a bubbling absorber of a water-based nanofluid. It was demonstrated that the addition of nanoparticles increased the total absorption rate by 24%. Lee et al.16 and Ma et al.17 employed Al2O3, carbon nanotubes (CNTs) and other nanoparticles to study the CO2 absorption process in NH3/H2O solution. Moreover, Kim et al.18 measured the vapor absorption rate of a falling film type H2O/LiBr for CO2 absorption process employing SiO2 nanoparticles and reported a maximum enhancement of mass transfer rate up to 18%. While there are many studies on enhanced CO2 absorption,15–21 considering the advantages of AMP in solvent utilization, further studies on the unique and superior properties of nanofluids when AMP is used as a base fluid, which have not yet been reported, are needed for further development of AMP absorption systems. In this work, AMP-based nanofluids were utilized to control the CO2 capturing performance in order to enhance the absorption and desorption rate, as well as to improve its recycling performance.
Herein, we have systematically investigated the effects of type, concentration and different parameters of nanofluid on the absorption–desorption of CO2 based on 2-amino-2-methyl-1-propanol (AMP), and proposed the mechanism of the enhanced CO2 absorption and regeneration performance of AMP-based nanofluid. The experimental results provide a theoretical basis for the development of new hybrid absorbents with high absorption capacity, fast absorption rate and good desorption performance.
2 Experiment
2.1 Experimental materials and instruments
Herein, the following reagents were used: 2-amino-2-methyl-1-propanol (AMP), Shanghai McLean Biochemical Science and Technology Co. Ltd; CO2 gas with a purity (v/v) of 99.99%, Shaanxi Chang’ an Gas Co. Ltd; TiO2 (anatase, 99.7%, 15 nm), Al2O3 (α-phase, 99.6%,10–15 nm), SiO2 (99.6%, 15 nm), Shanghai McLean Biochemical Science and Technology Co. Ltd; deionized water. The instruments used in this study are gas mass flow meter, electronic balance, ultrasonic crusher, water bath, oil bath, CO2 gas analyzer, soap film flow meter.
During the experiments, the amount of CO2 and the pressure inside the reactor were recorded continuously. Pressure measurements were performed using an Omega pressure sensor with an accuracy of ±1.0% and a temperature sensor with an accuracy of ±2.7 K (at 100 °C). And the uncertainty of CO2 absorption was evaluated and determined to be ±2.1% for the pure solvent and ±1.0% for the nanofluidic absorbents. The uncertainty was ±2.0% for pure solvent desorption and ±2.5% for nanofluid determination.
2.2 Preparation of nanofluids
The different nanofluids were prepared by a two-step method before the experiment. Firstly, 15 wt% AMP aqueous solution was configured in advance as the absorbent base solution, then a certain amount (0.2/0.5/1.0/1.5/2.0 g L−1) of nanoparticles was added to the base solution, followed by magnetic stirring for 30 min. Afterwards, the nanoparticles were dispersed by continuous cycling in an ultrasonic cleaner. In this study, different types of nanoparticles were used, including: TiO2, Al2O3 and SiO2. In order to avoid a rapid increase in the temperature of the solution, the experiment was carried out by intermittent vibration, with two seconds of vibration followed by two seconds of interval, and the effective vibration time was 60 min.
2.3 Experimental setup and procedure
The experimental system for nanoparticle-enhanced AMP solution absorption and desorption of CO2 is shown in Fig. 1. The absorption process takes place in a bubbling reactor, where the gas output from the CO2 cylinder is controlled and maintained at a certain pressure and mass flow rate via a gas mass flow meter. Pour 100 g of the pre-prepared nanofluid into the reactor, the water bath was heated to 40 °C ahead of time, and pure CO2 gas with purity >> 99% was supplied to the bottom of the bubble absorber (3) at a given flow rate, and the counter-current flow was directed to the liquid absorbent supplied to the top of the absorber to produce absorption, and the CO2 absorption was determined by the measurements of the mass of the solution at intervals of 10 min (g CO2 per 100 g of the solution).
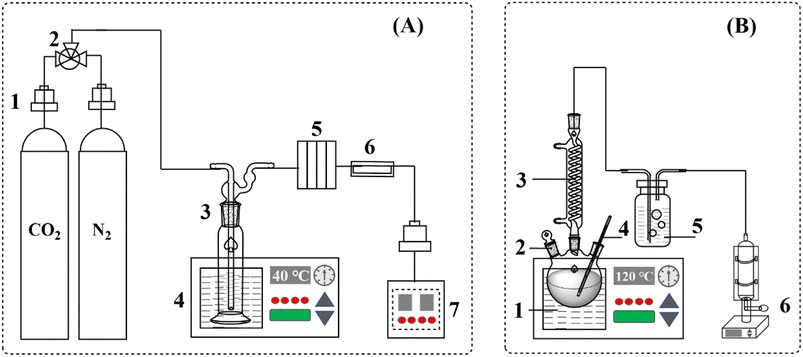 |
| Fig. 1 (A) Bubble absorption reaction device: 1-mass flow meter; 2-tee valve; 3-bubble absorption tube; 4-water bath; 5-condensation tube; 6-drying tube (glass tube with color-changing silica gel); 7-CO2 gas analyzer. (B) Desorption reaction device: 1-oil bath; 2-three-neck flask; 3-snake condenser tube; 4-laboratory thermometer; 5-drying flask (glass bottle with concentrated sulfuric acid); 6-soap film flow meter. | |
The desorption experiments were conducted in a three-necked flask with the rotor inserted and the flask corked tightly. The temperature of the oil bath was set to 120 °C in advance, and the three-necked flask was placed in it with magnetic stirring turned on, noting that the start of stirring was recorded as the reaction 0 moment. The temperature of the absorbent is increased by the heat exchange, which reduces the solubility of CO2 and separates CO2 from the absorbent. Impurities are similarly removed through the condenser tube so that only pure CO2 is measured. The desorbed CO2 was dried in a drying flask and entered into a soap film flowmeter, where the rate of desorption CO2 (mL min−1) at that moment was recorded. Measured flow rates were always corrected for the vapor pressure of water as a function of temperature. The initial solution volume is 100 mL, assuming that the solution volume does not change during the entire reaction.
2.4 Data analysis
In the absorption experiments, the CO2 loading in the liquid phase (α, mol CO2 per mol amine) was used to express the CO2 absorption performance as shown in eqn (1). In these tests, in order to investigate the CO2 desorption performance of the nanofluids, the desorption efficiency x is the ratio of the amount of substance of CO2 desorbed to the amount of substance of CO2 absorbed in total by the enriched fluid as shown in eqn (2).
It should be noted that the enhancement factor is usually defined as the ratio of the mass transfer coefficient of the nanofluid to the pure solution, and is used to evaluate the mass transfer enhancement of nanoparticles. In this work, the total CO2 uptake (mol CO2 per mol amine) was used to evaluate the absorption performance of the absorbent. The enhancement factor is defined here as the ratio of the total amount of CO2 absorbed/desorbed by the nanofluid to the pure AMP base fluid, denoted by Eabs and Edes, respectively, as shown in eqn (3) and (4), so that the effect of the nanoparticles on CO2 absorption/desorption can be investigated. All experiments were repeated three times to avoid uncertainty in the experimental results.
|
 | (1) |
|
 | (2) |
|
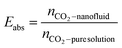 | (3) |
|
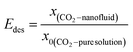 | (4) |
where
m,
m0,
MCO2,
M0,
ndes(CO2), and
nabs(CO2) are defined as the mass of CO
2 absorbed (g), the mass of amine in solution when no CO
2 is absorbed (g), the molar mass of CO
2 (g mol
−1), the molar mass of amine (g mol
−1), the amount of CO
2 desorbed in mol, and the amount of CO
2 absorbed in total by the enriched solution in mol, respectively.
3 Results and discussion
3.1 Absorption performance
3.1.1 Effect of nanofluid type. As can be seen from Fig. 2A, when the molar concentration of the AMP base solution is certain, the CO2 absorption rate of several nanofluids is greater than that of the pure solution during the first 40 min of absorption. There is a gradual decrease in the rate of CO2 uptake for each absorber as the reaction proceeds, due to the fact that the solution is saturated at this point.
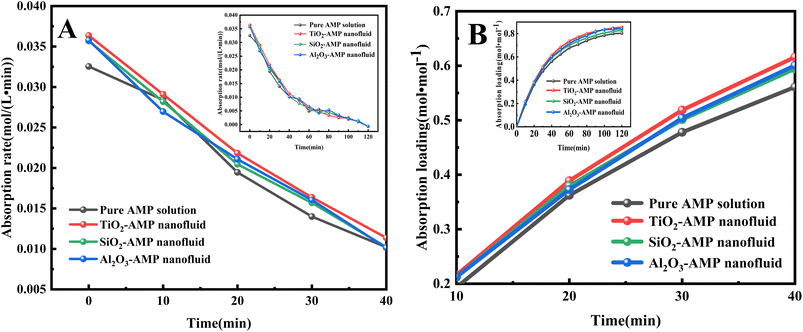 |
| Fig. 2 (A) CO2 absorption rate of different absorbents; (B) CO2 absorption loading of different absorbents. | |
The real-time absorption loading of all three nanofluids was also greater than that of the pure AMP solution at the initial stage (i.e., within 10–40 min) (Fig. 2B), suggesting that the addition of nanoparticles can enhance the absorption loading of CO2. Furthermore, the total CO2 absorption of TiO2-AMP nanofluid increased by 4.32% and Al2O3-AMP nanofluid increased by 3.71% than the pure AMP solution during 0–120 min, whereas the total CO2 absorption of SiO2-AMP nanofluid increased by only 1.39% than the pure AMP solution. This may be due to the fact that TiO2 nanoparticles contain more oxygen vacancies and can adsorb more CO2 molecules. Since the absorption of CO2 is nearly saturated in the solution after 120 min, the absorption process only lasted for 120 min in the following tests.
3.1.2 Effect of nanoparticle solid content. In order to investigate the effects of different types of nanoparticles with different solid contents on CO2 absorption mass transfer enhancement, three types of nanoparticles of the same size (15 nm) were used in this study. It was found that the CO2 absorption enhancement factor of the nanofluids increased when the solid content of the nanoparticles was increased from 0.2 g L−1 to 1.0 g L−1. However, after the solid content of nanoparticles reached 1 g L−1, the absorption enhancement factor gradually decreased (Fig. 3). As can also be seen from the figure, the absorption enhancement factor of TiO2-AMP nanofluid is better than other two nanofluids. The similar phenomenon was also reported by Turanov.22 In other words, the optimum solid content of nanoparticles enhances the CO2 absorption loading. Kesshishian et al.23,24 also reported the presence of optimum solid content. As solid content increases, the number of nanoparticles in the mass transfer film increases, resulting in enhanced Brownian motion and increased mass transfer rate. However, when the solid content exceeds a certain value, the area of the gas–liquid interface is limited,24 which may also increase particle agglomeration and precipitation, thereby hindering the mass transfer and leading to lower absorption loading.
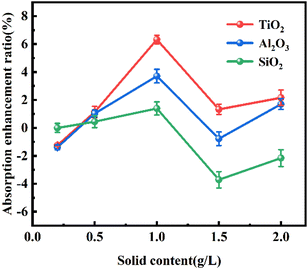 |
| Fig. 3 Enhancement ratio of CO2 absorption for different types of absorbents with different solid contents. | |
3.1.3 Effect of gas flow rate. Gas volume flow rate was also found to significantly affect CO2 absorption.25 Herein, we investigate the effect of gas–liquid flow rate on CO2 absorption loading by varying the CO2 volumetric flow rate in TiO2-AMP nanofluid (0.06/0.12/0.24/0.36 L min−1). The results showed that the CO2 absorption rate increased with increasing CO2 volume flow rate (Fig. 4A), which was attributed to the fact that the number of bubbles entering the liquid phase and the bubble rise rate increased per unit time with increasing gas flow rate, and thus the turbulence around the bubbles was enhanced, leading to enhanced mass transfer based on the boundary mixing effect, and ultimately leading to a larger change in the CO2 absorption rate. In addition, it was also found that when the volume flow rate of CO2 was 0.36 L min−1, the gas flow rate was too fast, the gas–liquid contact time was shorter, and the reaction could not be carried out sufficiently, leading to an insignificant change in the absorption rate.
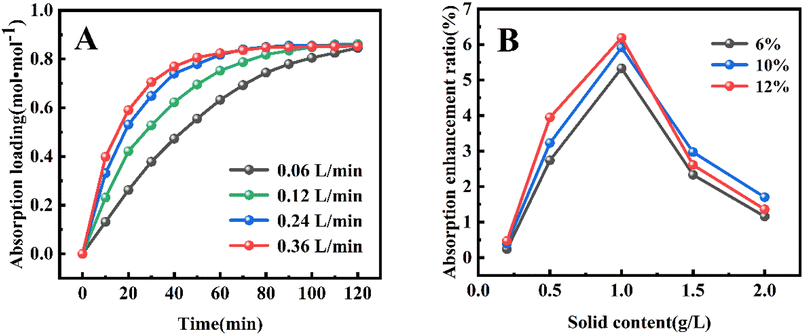 |
| Fig. 4 (A) Effect of different CO2 volume flow rates on CO2 absorption loading; (B) effect of different CO2 volume fractions on CO2 absorption. | |
Moreover, different CO2 volume fraction of 6%, 10% and 12% (Fig. 4B) was chosen to investigate the effect of initial CO2 volume fractions on the absorption performance (Fig. 4B), and the variation of CO2 absorption enhancement factor of TiO2-AMP nanofluid with nanoparticle solid content was investigated as well. The enhancement factor was found to increase with the increase in initial CO2 volume fraction when the optimum solid content was reached. According to Henry's law, it is known that as the initial CO2 volume fraction (CO2 partial pressure) increases, it will cause the CO2 equilibrium concentration to increase, leading to an increase in the depth of CO2 diffusion into the liquid phase, i.e., the mass transfer depth.22,26 In this case, more nanoparticles will enter the mass transfer boundary layer, which increases turbulence and promotes the mixing of nanofluids with different concentrations of CO2.
3.1.4 Variation of solubility with temperature. There are also differences in CO2 solubility at different temperatures, as shown in Fig. 5. It was possible to achieve high CO2 loading in TiO2-AMP nanofluids at 0.3–0.45 mol CO2 per mol even at relatively low partial pressure of CO2 (10–20 kPa). At a high temperature of 70–80 °C, the solubility of CO2 decreased significantly. When the partial pressure of CO2 was 10–100 kPa, nevertheless, the solubility of CO2 in the nanofluid was very low at 80 °C, which was 0.01–0.05 mol CO2 per mol.
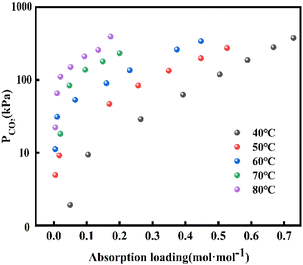 |
| Fig. 5 Variation of CO2 solubility with nanofluid temperature. | |
3.1.5 Absorption cycle stability of TiO2-AMP nanofluids. The cycling performance of an absorbent is an important judgement criterion to determine whether an absorbent can be used for industrial production. Fig. 6A shows the absorption loading of the absorbent in each cycle with pure AMP solution and 1 g L−1 TiO2-AMP nanofluid as experimental objects. From the experimental results, it can be seen that the CO2 absorption loading of the TiO2-AMP nanofluid absorbent can still reach 0.6 mol CO2 per mol amine after five cycles, which indicates that the TiO2-AMP nanofluid has an excellent cycling performance. The CO2 absorption loading of the nanofluid decreased by 33.33% after the 5th cycle, which is comparable to the 3rd cycle of the pure solution (Fig. 6B). This demonstrated that the incorporation of nanoparticles could improve the cycling performance of the absorbent.
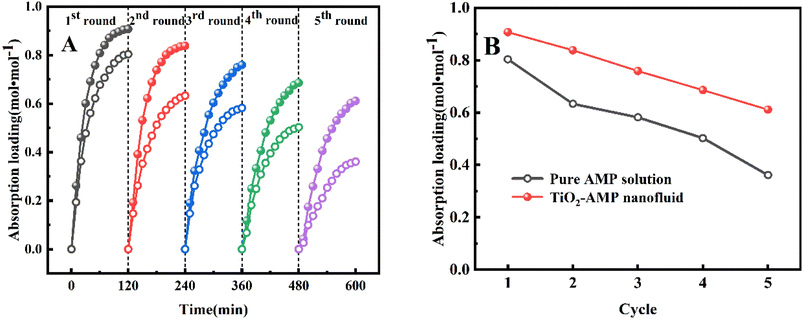 |
| Fig. 6 (A) and (B) Comparison of the performance of nanofluid and pure solution for CO2 multiple absorption; solid indicates TiO2-AMP nanofluids, hollow indicates pure AMP solution. | |
3.2 Desorption performance
3.2.1 Effect of nanofluid type. Fig. 7A represents the effect of different nanofluids on CO2 desorption rate in the same time range. Compared with the pure AMP solution, the CO2 desorption rate of nanofluids was much higher than the pure solution from 0 to 15 min. The TiO2-AMP nanofluid has the largest rate of CO2 desorption than Al2O3-AMP and SiO2-AMP nanofluid, owing to the fact that there are more oxygen vacancies in TiO2, which can adsorb more CO2, and therefore more CO2 is desorbed. And at the end of the desorption process, the desorption rate can be seen to change little over time. This is because as time goes by, the CO2 concentration inside the absorbent becomes very low and the effect of nanoparticles on the desorption performance decreases. The solid content of nanoparticles has a great influence on the rate of CO2 desorption from the absorbent-rich liquid, as seen in Fig. 7B, the desorption rate reaches the highest at the optimal solid content of 1 g L−1. Fewer nanoparticles result in less CO2 absorption and desorption, and more nanoparticles may hinder the mass transfer. As the experimental results showed that TiO2 nanoparticles were more effective in enhancing CO2 desorption and the optimum solid content was 1 g L−1 (±1.0%, ±2.5%).
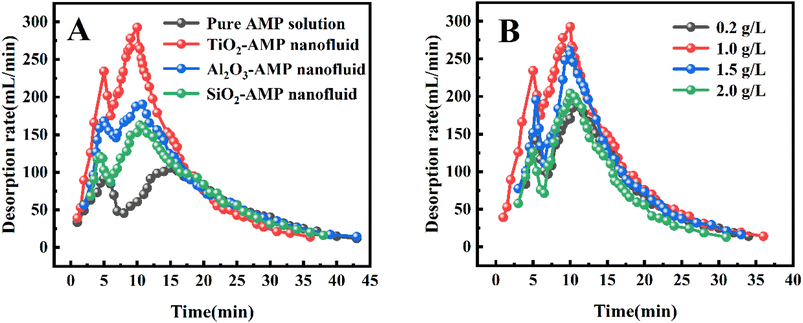 |
| Fig. 7 (A) CO2 desorption rates of different absorbents; (B) CO2 desorption rates of nanofluids with different solid contents. | |
3.2.2 Effect of desorption temperature. As well as temperature is an important factor to influence the desorption efficiency. In this paper, the CO2 desorption efficiency and generation cycle were investigated at different temperatures. The results in Fig. 8 clearly display that the desorption efficiency is extremely sensitive to the change of desorption temperature, whether pure AMP solution or nanofluid, and the desorption efficiency increases with the increase of desorption temperature. When the desorption temperature was increased from 80 °C to 120 °C, the desorption efficiency of the pure AMP solution increased from 86.2% to 98.3%, while the desorption efficiency of the nanofluid increased from 86% to 99%. Along with the gradual increase of temperature (especially 100 °C–120 °C), the increase of desorption efficiency becomes smaller, and the CO2 generation cycle is shortened. The energy consumption of solvent desorption is also an important parameter,41 and it was demonstrated by experimental results that TiO2-AMP nanofluid can have a well desorption efficiency at a low energy consumption, where the desorption temperature is 120 °C, the desorption efficiency is high and the desorption time is shortened by 12.5%.
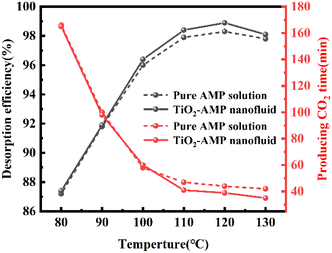 |
| Fig. 8 Effect of desorption temperature on desorption efficiency and CO2 generation period. | |
3.2.3 Absorption–desorption cyclic stability of different nanofluids. To evaluate the performance of the absorbents, the absorption–desorption cycling experiments were repeated three times for each absorbent. From the above, a higher absorption cycling loading was found for TiO2-AMP nanofluid than the others, and its cycling desorption performance was also revealed to be superior to the other nanofluids through further desorption experiments. Upon three cycles, the TiO2-AMP nanofluid desorption enhancement ratio reached 9.3% (Fig. 9). As a result, it was proved that the TiO2-AMP nanofluid behaved well in CO2 desorption likewise. Table 1 reveals a comparison of the enhancement of CO2 desorption rate by nanofluids42–46 with the present experiments.
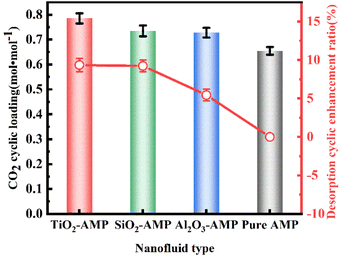 |
| Fig. 9 Average CO2 cyclic loading and desorption cyclic enhancement ratio for different nanofluids. | |
Table 1 Comparison of CO2 desorption performance of nanofluids
Authors |
Nanoparticle |
Solvent |
Conclusion |
Wang et al.44 |
Al2O3 (0.1 wt%) |
MEA/MDEA/PZ |
Enhancement performance is: PZ > MEA > MDEA |
SiO2 (0.1 wt%) |
Lee et al.46 |
Al2O3 (0.01 vol%) |
DI water |
Maximum CO2 absorption/regeneration performance enhancements are 23.5% and 11.8% |
SiO2 (0.01 vol%) |
Kim et al.27 |
Al2O3 (0.1 wt%) |
Methanol |
Enhanced up to 26% at 0.01 vol% compared with pure methanol |
Present experiments |
TiO2 (1 g L−1) |
AMP |
Enhancement performance is: TiO2 > SiO2 > Al2O3 |
Al2O3 (1 g L−1) |
SiO2 (1 g L−1) |
3.3 Absorption/desorption enhancement mechanisms of nanofluid absorbent
Currently, a large number of studies have discussed the mechanisms of nanoparticle-enhanced CO2 absorption/desorption in alcoholic amine solutions, but these mechanisms often only explain part of the experimental phenomena,19–21 few desorption experiments have been carried out.42,43 In this paper, the effect of nanoparticles on the absorption/desorption of CO2 from AMP aqueous solutions is investigated, and the enhancement mechanism is discussed in depth using TiO2-AMP nanofluids as an example (Fig. 10).
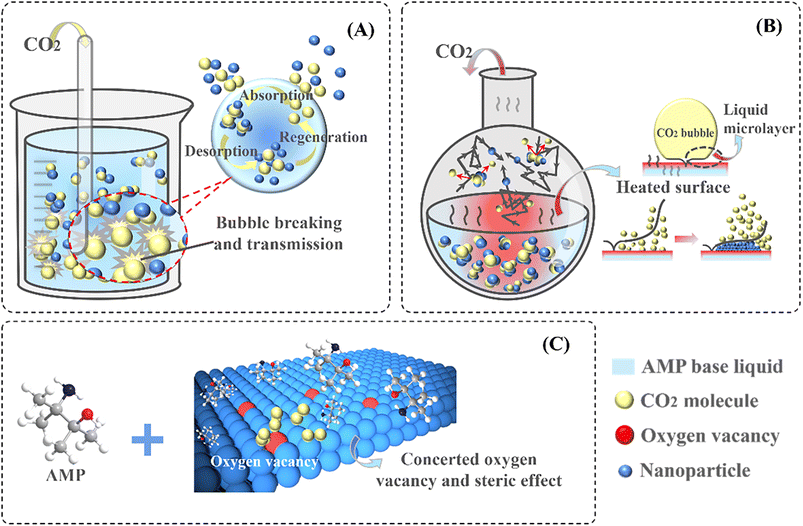 |
| Fig. 10 (A) Absorption/(B) desorption mechanisms of TiO2-AMP nanofluids and (C) present experiments. | |
Firstly, the agitation of the nanofluid and the movement of the nanoparticles make the bubbles constantly collide, leading to the rupture and deformation of the CO2 bubbles dispersed in the nanofluid, so that a larger contact area is caused, thus enhancing the absorption performance.15,27 On the other hand, TiO2 is a nanoparticle with high CO2 adsorption capacity, Liu37 et al. also modulated the preferential CO2 adsorption sites on the material surface by interfacial effects and found that CO2 adsorption on TiO2 surfaces with point defects (oxygen vacancies) was significantly changed. TiO2 nanoparticles move randomly with adsorbed CO2 in the base solution, i.e., the particles transport additional CO2 across the gas–liquid interface via adsorption and desorption.28–35 The presence of oxygen vacancies introduces additional adsorption sites due to the impregnation of TiO2 nanoparticles in the AMP base solution, and together with the spatial site-blocking effect, the stability of the system is effectively controlled,36,40 which improves the performance of the absorbent. In terms of Al2O3/SiO2-AMP nanofluids, SiO2 nanoparticles have fewer defect sites.38,39 Even though Al2O3/SiO2 nanoparticles may hinder the gas–liquid mass transfer, their enhancement factor is less than 1. As the solid content increases, the number of nanoparticles and mutual interference increase, the nanoparticles around the bubbles prevented the bubbles from agglomerating, leading to an increase in the gas–liquid mass transfer area. Hence all these mechanisms do not act individually, but together contribute to the improvement of the absorption performance.
Further experimental results were also analyzed to determine which effect had the largest impact on enhancing CO2 desorption. As mentioned earlier, the superior absorption properties of TiO2-AMP nanofluids have benefited from the presence of oxygen vacancies, and the CO2 adsorption/desorption by oxygen vacancies on the TiO2 surface is currently more studied. It has been reported that the presence of oxygen vacancies increases the peaks of thermal desorption spectra (TDS) of TiO2 surface at 166 K and 200 K.47 The Brownian motion becomes more active with the increase of temperature, accelerating the desorption process. Besides, AMP's unique spatial site resistance effect makes the particles stably and uniformly distributed in the base liquid, which effectively prevents the flocculation and agglomeration of TiO2 nanoparticles. Meanwhile, TiO2 nanoparticles have high thermal stability, and the presence of the nanoparticles also leads to a rapid increase in the temperature of the absorbent, which allows CO2 to be desorbed quickly. Numerous studies have confirmed the enhanced heat transfer in nanofluids.45 As for the existence of Al2O3 nanoparticles, the desorption performance is reduced compared to TiO2-AMP nanofluid, because of the high surface potential of the Al2O3 nanoparticles as pH varies, which also means that the desorption temperature must be applied at a much higher temperature than the traditional conditions,48 so that CO2 gas can be desorbed from the Al2O3 nanoparticles. Nevertheless, this effect does not exist in the case of semiconductor oxides (SiO2).43
In general, AMP are often used as absorption enhancers and viscosity modifiers, and nanofluids are employed to capture CO2 for lower energy consumption. In this study, it was found that the performance of nanofluids in both absorption and desorption was improved compared the pure solution. General improvement in the efficiency of the absorption–desorption system was achieved through the use of nanofluids, and it is expected that the mixed amine CO2 capture system will be incorporated with the nanofluids mentioned in this paper to achieve a favorable absorption–desorption performance of the CO2 cycle. Considering the comprehensive application of the actual system, future research should be based on the actual operation and the economic benefits should also be taken into account in detail in the life cycle cost analysis, which will be carried out in the next step of this study.
4 Conclusion
In this paper, the effects of different nanoparticles on the CO2 absorption–desorption performance of AMP-based fluids were investigated. TiO2-AMP nanofluid, Al2O3-AMP nanofluid, and SiO2-AMP nanofluid were used as absorbents and their CO2 absorption–desorption performances were evaluated, and the following conclusions were obtained:
(1) At a certain molar concentration of base solution AMP, the CO2 absorption rate of nanofluid were greater than pure AMP solution for the first 40 min of absorption, which indicated that nanoparticles could enhance the CO2 absorption rate in the diffusion control phase.
(2) The CO2 absorption capacity of TiO2 nanoparticles was found to be better than Al2O3 and SiO2 nanoparticles, and the enhancement of absorption was TiO2 > Al2O3 > SiO2 in descending order. The desorption enhancement factor of TiO2 nanoparticles was also found to be higher than the others, and the enhancement of desorption was found to be TiO2 > SiO2 > Al2O3, and all of them exist with the optimal solid content of 1 g L−1 (±1.0%, ±2.5%).
(3) The TiO2-AMP nanofluid desorption enhancement ratio reached 9.2%, approximating the SiO2-AMP nanofluid enhancement ratio.
(4) Based on the mechanism of nanofluids to promote mass transfer, combined with the unique spatial site resistance effect of AMP, the mechanisms of various nanofluids to enhance the CO2 absorption–desorption performance were discussed.
Author contributions
Qiuli Zhang: revision, supervision, funding acquisition. Zhongyi Ning: research, experimental conducting, discussion, writing – original draft. Xuelian Li: discussion, supervision. Xiaogang Ning, Fan Wu: experimental conducting. Jun Zhou: revision, supervision.
Conflicts of interest
All co-authors have seen and agree with the contents of the manuscript and there is no financial interest to report. We certify that the submission is original work and is not under review at any other publication.
Acknowledgements
The authors would like to acknowledge the support of the Innovation Capability Support Program of Shaanxi (Program no. 2020TD-028), the Natural Science Basic Research Program of Shaanxi (Program no. 2019JLP-17).
References
- IEA, Global energy & CO2 status report 2021, International Energy Agency, 2021. https://www.iea.org/reports/global-energy-review-2021 Search PubMed.
- K. Zickfeld, D. Azevedo, S. Mathesius and H. D. Matthews, Asymmetry in the climate carbon cycle response to positive and negative CO2 emissions, Nat. Clim. Change, 2021, 11, 613–617, DOI:10.1038/s41558-021-01061-2.
- X. Zhu, H. Lu, K. Wu, Y. Zhu, Y. Liu, C. Liu and B. Liang, DBU-glycerol solution: a CO2 absorbent with high desorption ratio and low regeneration energy, Environ. Sci. Technol., 2020, 54, 7570–7578, DOI:10.1021/acs.est.0c01332.
- Y. Shen, H. Chen, J. Wang, S. Zhang, C. Jiang, J. Ye, L. Wang and J. Chen, Two-stage interaction performance of CO2 absorption into biphasic solvents: mechanism analysis, quantum calculation and energy consumption, Appl. Energy, 2020, 260, 114343 CrossRef CAS.
- X. Zhou, G. Jing, B. Lv, F. Liu and Z. Zhou, Low-viscosity and efficient regeneration of carbon dioxide capture using a biphasic solvent regulated by 2-amino-2-methyl-1-propanol, Appl. Energy, 2019, 235, 379–390 CrossRef CAS.
- S. K. Wai, C. Nwaoha, C. Sai wan, R. Idem and T. Supap, Absorption heat, solubility, absorption and desorption rates, cyclic capacity, heat duty, and absorption kinetic modeling of AMP−DETA blend for post-combustion CO2 capture, Sep. Purif. Technol., 2018, 194, 89–95 CrossRef CAS.
- S.-J. Han and J.-H. Wee, CO2 Absorption Performance and Electrical Properties of 2-Amino-2-methyl-1-propanol Compared to Monoethanolamine Solutions as Primary Amine-Based Absorbents, Energy Fuels, 2021, 35(4), 3197–3207 CrossRef CAS.
- C. Nwaoha, C. Saiwan, T. Supap, R. Idem, P. Tontiwachwuthikul, W. Rongwong, M. J. Al-Marri and A. Benamor, Carbon dioxide (CO2) capture performance of aqueous tri-solvent blends containing 2-amino-2-methyl-1-propanol (AMP) and methyl diethanolamine (MDEA) promoted by diethylenetriamine (DETA), Int. J. Greenhouse Gas Control, 2016, 53, 292–304 CrossRef CAS.
- W. Conway, S. Bruggink, Y. Beyad, W. Luo, I. Melian-Cabrera, G. Puxty and P. Feron, CO2 absorption into aqueous amine blended solutions containing mono ethanol amine (MEA), N, N-dimethylethanolamine (DMEA), N, N-diethylethanolamine (DEEA) and 2-amino-2-methyl-1-propanol (AMP) for post-combustion capture processes, Chem. Eng. Sci., 2015, 126, 446–454 CrossRef CAS.
- W. M. Budzianowski, Explorative analysis of advanced solvent processes for energy efficient carbon dioxide capture by gas-liquid absorption, Int. J. Greenhouse Gas Control, 2016, 49, 108–120 CrossRef CAS.
- M. Arshadi, H. Taghvaei and M. Abdolmaleki, et al., Carbon dioxide absorption in water/nanofluid by a symmetric amine-based nano-dendritic adsorbent, Appl. Energy, 2019, 242, 1562–1572 CrossRef CAS.
- D. S. Mannel, G. Qi and L. R. Widger, et al., Enhancements in mass transfer for carbon capture solvent part II: Micron-sized solid particles, Int. J. Greenhouse Gas Control, 2017, 61, 138–145 CrossRef CAS.
- P. Agarwal, H. Qi and L. A. Archer, The ages in a self-suspended nanoparticle liquid, Nano Lett., 2010, 10(1), 111–115 CrossRef CAS PubMed.
- Z. Zhang, J. Cai and F. Chen, et al., Progress in enhancement of CO2 absorption by nanofluids: A mini review of mechanisms and current status, Renewable Energy, 2018, 118, 527–535 CrossRef CAS.
- W. G. Kim, H. U. Kang, K. M. Jung and S. H. Kim, Synthesis of silica nanofluid and application to CO2 absorption, Sep. Sci. Technol., 2008, 43(11e12), 3036e55 Search PubMed.
- J. K. Lee, J. Koo, H. Hong and Y. T. Kang, The effects of nanoparticles on absorption heat and mass transfer performance in NH3/H2O binary nanofluids, Int. J. Refrig., 2010, 33(2), 269e75 Search PubMed.
- X. Ma, F. Su, J. Chen, T. Bai and Z. Han, Enhancement of bubble
absorption process using a CNTs-ammonia binary nanofluid, Int. Commun. Heat Mass Transfer, 2009, 36, 657e60 Search PubMed.
- H. Kim, J. Jeong and Y. T. Kang, Heat and mass transfer enhancement for falling film absorption process by SiO2 binary nanofluids, Int. J. Refrig., 2012, 35(3), 645e51 CrossRef.
- W. Yu, T. Wang and A.-H. A. Park, et al., Review of liquid nano-absorbents for enhanced CO2 capture, Nanoscale, 2019, 11(37), 17137–17156 RSC.
- J. Zhang, S. Li and L. Peng, et al., Progress in research on gas-liquid mass transfer enhancement of nanofluids, Chem. Ind. Eng. Prog., 2013, 32(4), 732–739 CAS.
- S. Y. Cheng, Y. Z. Liu and G. S. Qi, Progress in the enhancement of gas-liquid mass transfer by porous nanoparticle nanofluids, J. Mater. Sci., 2019, 54(20), 13029–13044 CrossRef CAS.
- A. N. Turanov and Y. V. Tolmachev, Heat- and mass-transport in aqueous silica nanofluids, Heat Mass Transfer, 2009, 45, 1583–1588 CrossRef CAS.
- N. Keshishian, M. Nasr Esfahany and N. Etesami, Experimental investigation of mass transfer of active ions in silica nanofluids, Int. Commun. Heat Mass Transfer, 2013, 46, 148–153 CrossRef CAS.
- S. Karve and V. A. Juvekar, Gas absorption into slurries containing fine catalyst particles, Chem. Eng. Sci., 1990, 45, 587–594 CrossRef CAS.
- J. Z. Jiang, B. Zhao and Y. Q. Zhuo, et al., Experimental study of CO2 absorption in aqueous MEA and MDEA solutions enhanced by nanoparticles, Int. J. Greenhouse Gas Control, 2014, 29135–29141 Search PubMed.
- Y. M. Xuan and Q. Li, Nanofluid Energy Transfer Theory and Application, Science Press, Beijing, 2010, pp. 154–162 Search PubMed.
- J. H. Kim, C. W. Jung and Y. T. Kang, Mass transfer enhancement during CO2 absorption process in methanol/Al2O3 nanofluids, Int. J. Heat Mass Transfer, 2014, 76, 484–491 CrossRef CAS.
- L. J. Won, P. I. Torres and L. J. Hun., et al., Combined CO2 absorption/regeneration performance enhancement by using nano-absorbents, Applied energy, 2016, 178(Sep.15), 164–176 Search PubMed.
- E. Alper, B. Wichtendahl and W. D. Deckwer, Gas-absorption mechanism in catalytic slurry reactors, Chem. Eng. Sci., 1980, 35, 217–222 CrossRef CAS.
- E. Alper and S. Ozturk, The effect of activated carbon loading on oxygen absorption into aqueous sodium sulfide solutions in a slurry reactor, Chem. Eng. J. Biochem. Eng. J., 1986, 32, 127–130 CAS.
- J. T. Tinge and A. A. H. Drinkenburg, Absorption of gases into activated carbon water slurries in a stirred cell, Chem. Eng. Sci., 1992, 47, 1337–1345 CrossRef CAS.
- J. H. J. Kluytmans, B. G. M. van Wachem, B. F. M. Kuster and J. C. Schouten, Mass transfer in sparged and stirred reactors: influence of carbon particles and electrolyte, Chem. Eng. Sci., 2003, 58, 4719–4728 CrossRef CAS.
- V. Linek, M. Kordac and M. Soni, Mechanism of gas absorption enhancement in presence of fine solid particles in mechanically agitated gas–liquid dispersion. Effect of molecular diffusivity, Chem. Eng. Sci., 2008, 63, 5120–5128 CrossRef CAS.
- S. Kim, R. Xu, W. Lee, C. K. Choi and Y. T. Kang, CO2 absorption performance enhancement by dodecane nano emulsion absorbents, J. CO2 Util., 2019, 30, 18–27 CrossRef CAS.
- J. A. Eastman, S. U. S. Choi and S. Li, et al., Anomalously increased effective thermal conductivities of ethylene glycol-based nanofluids containing copper nanoparticles, Appl. Phys. Lett., 2001, 78(6), 718–720 CrossRef CAS.
- Z. Sheng nan, T. Lina and Y. Lixin, et al., Phosphine-based ionic liquids for CO2 chemical fixation: Improving stability and activity by asymmetric flexible steric hindrance, J. Environ. Chem. Eng., 2023, 11(3), 109883 CrossRef.
- L. Liu, Z. Liu and H. Sun, et al., Morphological effects of Au13 clusters on the adsorption of CO2 over anatase TiO2 (101), Appl. Surf. Sci., 2017, 399, 469–479 CrossRef CAS.
- G. Pragati, J. G. Navea and S. C. Larsen, et al., Carbon dioxide (C∼ (16)O_22 and C∼ (18)O_2) adsorption in zeolite Y materials: effect of cation, adsorbed water and particle size, Energy Environ. Sci., 2009, 2(4), 401–409 RSC.
- A. Haghtalab, M. Mohammadi and Z. Fakhroueian, Absorption and solubility measurement of CO2 in water-based ZnO and SiO2 nanofluids, Fluid Phase Equilib., 2015, 392, 33–42 CrossRef CAS.
- T. L. Thompson, O. Diwald and J. Yates, et al., CO2 as a Probe for Monitoring the Surface Defects on TiO2 (110) Temperature-Programmed Desorption, J. Phys. Chem. B, 2003, 107(42), 11700–11704 CrossRef CAS.
- P. Zhang, Y. Shi and J. Wei, Regeneration of 2-amino-2-methyl-1-propanol used for carbon dioxide absorption, J. Environ. Sci., 2008, 20(1), 39–44 CrossRef CAS PubMed.
- J. W. Lee, I. T. Pineda, J. H. Lee and Y. T. Kang, Combined CO2 absorption/regeneration performance enhancement by using nano-absorbents, Appl. Energy, 2018, 178, 164–176 CrossRef.
- J. S. Lee, J. W. Lee and Y. T. Kang, CO2 absorption/regeneration enhancement in DI water with suspended nanoparticles for energy conversion application, Appl, Energy, 2015, 143, 119–129 CAS.
- T. Wang, W. Yu, F. Liu, M. Fang, M. Farooq and Z. Luo, Enhanced CO2 absorption and desorption by mono ethanolamine (MEA)-based nanoparticle suspensions, Ind. Eng. Chem. Res., 2016, 55(28), 7830–7838 CrossRef CAS.
- X. Jiajun, Y. Bao and H. Boualem, Thermophysical Properties and Pool Boiling Characteristics of Water-in-Polyalphaolefin Nanoemulsion Fluids, J. Heat Transfer, 2013, 135(9), 0913031–0913036 Search PubMed.
- J. H. Lee, J. W. Lee and Y. T. Kang, CO2 regeneration performance enhancement by nanoabsorbents for energy conversion application, Appl. Therm. Eng., 2016, 103, 980–988 CrossRef CAS.
- T. L. Thompson, O. Diwald and J. Yates, et al., CO2 as a Probe for Monitoring the Surface Defects on TiO2 (110) Temperature-Programmed Desorption, J. Phys. Chem. B, 2003, 107(42), 11700–11704 CrossRef CAS.
- L. J. Won, P. I. Torres and L. J. Hun., et al., Combined CO2 absorption/regeneration performance enhancement by using nanoabsorbents, Appl. Energy, 2016, 178, 164–176 CrossRef.
- L. Fang, H. Liu and Y. Bian, et al., Experimental Study on Enhancement of Bubble Absorption of Gaseous CO2 with Nanofluids in Ammonia, J. Harbin Inst. Technol., 2017, 24(02), 80–86 Search PubMed.
|
This journal is © The Royal Society of Chemistry 2023 |
Click here to see how this site uses Cookies. View our privacy policy here.