DOI:
10.1039/D3RA07367J
(Paper)
RSC Adv., 2023,
13, 35562-35568
Hydrophilic amorphous Cr2O3 supported Co species toward efficient hydrogen production from ammonia borane under visible light irradiation†
Received
29th October 2023
, Accepted 29th November 2023
First published on 6th December 2023
Abstract
Herein, we synthesized a hydrophilic support formed from amorphous Cr2O3 and KIT-6 containing P123 using a simple solvent-free thermal method for Co species. The obtained catalyst (denoted as Co/Cr2O3-F-X) exhibits high activity for hydrogen production from ammonia borane (NH3BH3). The optimal Co/Cr2O3-F-0.5 exhibits the highest catalytic performance with the turnover frequency (TOF) value of 111.1 min−1. Advanced characterizations suggest the high catalytic performance is attributed to the synergistic effect of the strong interaction between amorphous Cr2O3 and KIT-6 containing P123 and improved wettability of the catalyst.
1. Introduction
Hydrogen (H2) as clean energy has attracted much attention owing to zero carbon emissions and high combustion value.1–3 Ammonia borane (NH3BH3) as a promising hydrogen storage material with high hydrogen content (19.6 wt%) can present the solution for the challenges of safe transportation and storage of H2.4,5 The hydrolysis of NH3BH3 (NH3BH3 + 2H2O → NH4BO2 + 3H2) is considered a promising strategy to generate H2 due to the mild reaction conditions.6,7 Supported heterogeneous catalysts play an important role in accelerating the reaction rate.8–10 Various metal based catalysts including precious and non-precious metals have been employed for hydrolysis of NH3BH3.11–18 However, the high cost of precious metals and low activity of non-precious metals hinder the large-scale application of NH3BH3. Many efforts have been made to decrease the dosage of precious metals and enhance the activity of non-precious metal based catalysts. Regulating the electronic structure of active metals is a common strategy to improve the activity of catalysts. Recently, introducing light irradiation into reaction systems, especially non-precious metals working as catalysts, has been proven to be an effective method to boost the charge imbalance distribution and then catalytic performance.19–22 Nevertheless, the activity of non-precious metal catalyst is still far from satisfaction. As a result, it is urgent to rationally design the structure of non-noble metal catalysts to obtain high activity level.
Earth-abundant Co catalysts have been utilized in NH3BH3 hydrolysis.23–26 For example, Co-Co3O4 has been employed as excellent catalyst for H2 generation from NH3BH3 due to electronic structures at the interface and abundant adsorption site.27 Our group have also prepared high performance Co/Cr2O3 composed of electron-deficient Co species and mesoporous Cr2O3. Cr2O3 is selected as support due to several reasons. Firstly, Cr2O3 has been widely applied in photoinduced reaction.28 Secondly, Cr2O3 with relatively high work function induces electron transfer from Co. Importantly, electron-deficient Co species can decrease H2O activation and dissociation energy barriers (rate-determining step in NH3BH3 hydrolysis) and then accelerate the rate of reaction.29 To date, the design of catalyst has mainly focused on tuning electronic structures of active metal, while neglects catalyst wettability. The appropriate wettability of a catalyst can significantly enhance the adsorption and transfer of reactants, resulting in enhancement activity.30 For example, Zhao group reported the activity of NiFe/NiFe:Pi toward oxygen evolution is significantly enhanced by tuning the wettability of the catalyst.31 Xiao group reported the conversion of syngas to methane, dimethyl ether, and olefins can be regulated by controlling the catalyst hydrophobicity.32 Accordingly, regulating catalyst wettability is an effective strategy to further boost the rate of NH3BH3 hydrolysis. Introduction of hydrophilic SiO2 into reaction system can alter H2O adsorption behaviour.33 Therefore, introduction of material containing hydrophilic SiO2 is effective strategy to enhance the catalyst activity.
Herein, we prepared hydrophilic Co-based catalyst Co/Cr2O3-F composed with amorphous Cr2O3 and functionalized with KIT-6 containing P123 and Co species based our group previous report. The optimal Co/Cr2O3-F-0.5 exhibits a high catalytic performance with TOF value of 111.1 min−1. Advanced characterizations reveal the synergistic effect of strong interaction at interface and improved wettability is responsible for the high catalytic performance.
2. Experimental
2.1 Materials
Cobalt chloride hexahydrate (CoCl2·6H2O), ammonia borane (NH3BH3), deuterium oxide (D2O), potassium iodide (KI), tetraethyl orthosilicate (C8H20O4Si) and sodium borohydride (NaBH4) were obtained from Aladdin. Chromium(III) nitrate nonahydrate (Cr(NO3)3·6H2O) was obtained from Macklin. Isopropyl alcohol (C3H8O) was purchased from Tianjin Yongda ChemicalReagent Co. Ltd, poly(ethylene glycol)-block-poly(propylene glycol)-block-poly(ethylene glycol) was obtained from Sigma-Aldrich. Potassium dichromate (K2Cr2O7) were obtained from Sinopharm Chemical Reagent Co., Ltd.
2.2 Synthesis and photocatalytic H2 production
Preparation of Cr2O3-F-0.5: KIT-6-F was firstly synthesized according to reported literature with minor modification.34 We didn't calcine the sample to maintain the p123 in pore and on the surface of KIT-6. Next, a 0.5 g KIT-6-F and 0.2 g Cr(NO3)3 were ground and transferred into a Teflon-lined stainless steel and maintained at 240 °C for 3.5 h. Meanwhile, we prepared five other samples denoted as Cr2O3-F-X (X represents the quality of KIT-6-F). The KIT-6-E was prepared by calcination KIT-6-F at 550 °C for 3 h. The Cr2O3-E-0.5 was synthesized by KIT-6-E with same procedure as Cr2O3-F-0.5 for comparison.
Preparation of Co/Cr2O3-F-0.5 and catalytic H2 production: 16.0 mg Cr2O3-F-0.5 was dispersed in containing 0.034 mmol CoCl2·6H2O aqueous solution (0.9 mL) for in reactor 1 h. Next, 0.068 mmol NaBH4 acted as reduction and 1.71 mmol NH3BH3 were dissolved in 1.5 mL H2O and was injected into the reactor. Meanwhile, the H2 generated under visible light irradiation and in the dark. Five other samples including Co/Cr2O3-F-0.1, Co/Cr2O3-F-0.3, Co/Cr2O3-E-0.5, Co/KIT-6-F and Co/KIT-6-E were prepared by same method.
2.3 Catalyst characterization
Powder X-ray diffraction (PXRD) on D8 Advance with Cu-Kα radiation was employed to obtain crystalline structures and component of samples. Transmission electron microscopy (TEM, JEM-2100F) were used to observe the morphologies of samples. X-ray photoelectron spectroscopy (XPS) was conducted on a Thermo Scientific K-Alpha with pass energies = 50 eV and the calibrated using the C 1s peak at 284.8 eV was reference. PerkinElmer Lambda 750 was employed to collect UV-vis spectra of sample. Contact angle measurements were carried out on Dataphysics OCA40. TriStar II Plus 3.03 was used to measure the surface area of samples. The transient photocurrent and electrochemical impedance spectroscopy (EIS) were conducted on an electrochemical station (CHI600E) in 0.5 M Na2SO4 electrolyte. Fourier transform infrared (FTIR) was performed on FTIR-7600 spectrometer.
3. Results and discussion
3.1 Chemical structure analysis
As described in Fig. 1a, the catalyst Co/Cr2O3-F-0.5 was prepared by two-step method. Firstly, Cr2O3-F-0.5 was obtained by solvent-free thermal method. TEM image in Fig. 1b, S1a and S1b† shows the compound inherits the morphology of KIT-6. However, no long range ordered structure can be observed from HRTEM image, further confirming the amorphous nature of Cr2O3-F-0.5 (Fig. 1c). Moreover, the SAED of Cr2O3-F-0.5 (inset in Fig. 1c) demonstrates rings composed of diffraction spots, further verifying the amorphous feature of Cr2O3-F-0.5. XRD pattern displays abroad peak indexed to KIT-6,35 and no characteristic peak assigned to Cr2O3 was clearly observed suggesting the sample is composed with KIT-6-F and amorphous Cr2O3 (Fig. 2). Additionally, samples including KIT-6-F, Cr2O3-F-0.1, Cr2O3-F-0.3, and Cr2O3-F-0.6 were also tested to further confirm the amorphous nature of support (Fig. 2). The result shows the compound sample remains amorphous after adjusting the quality of KIT-6-F. Moreover, a closer look at the abroad peaks in the compound shifts to big larger 2θ angle with increasing KIT-6-F, suggesting the strong electronic interaction between KIT-6-F and Cr2O3. Similar phenomenon has been observed in metal alloys due to changed electronic structure.36 Additionally, the image and structure of Cr2O3-E-0.5 also be tested for comparison. As shown in Fig. S2,† Cr2O3-E-0.5 displays the same morphology as KIT-6 and Cr2O3-F-0.5. XRD pattern shows an amorphous nature of Cr2O3-E-0.5 which is similar to the Cr2O3-F-0.5 (Fig. S3†). Therefore, the introduction of P123 cannot significantly affect the crystallinity of support. After loading Co species, TEM images from Fig. 1d and S4† show Co species are formed on the surface of Cr2O3-F-0.5. The long-range order also can't be observed in HRTEM image of Co/Cr2O3-F-0.5 in Fig. 1e, indicating the catalyst is composed of amorphous component. The SAED of Co/Cr2O3-F-0.5 (inset in Fig. 1e) further confirms the amorphous feature of catalyst. Additionally, the element mapping (Fig. 1f–k) and EDX further the Co species has supported on the surface of Cr2O3-F-0.5 (Fig. S5†). No obvious sharp peak assigned to Co is observed in XRD pattern of Co/Cr2O3-F-0.5 (Fig. S6†), suggesting the formation of amorphous Co species which is consistent with previous reports.37 Additionally, the broad peak around 45° assigned to Co can further confirm the existence of amorphous Co.38 BET surface area of Cr2O3-F-0.5 was calculated to be 246.2512 m2 g−1 which is smaller than Co/Cr2O3-F-0.5 (296.8048 m2 g−1) (Fig. S7 and S8†). The result suggests Co species mainly disperse on the surface of Cr2O3-F-0.5 rather than channel of KIT-6 maybe due to the interaction between Co2+ and amorphous Cr2O3.
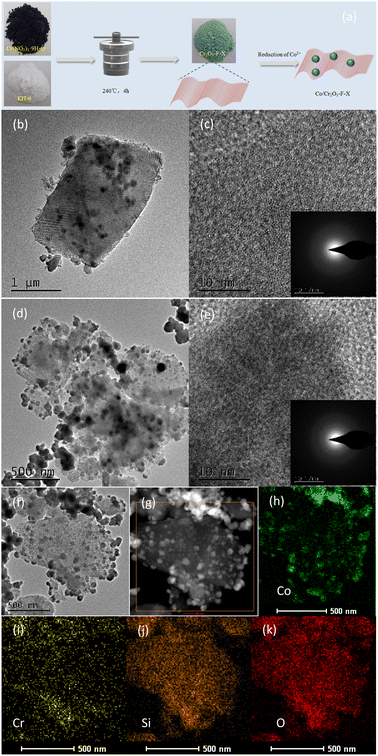 |
| Fig. 1 (a) The illustration preparation process of Co/Cr2O3-F-0.5, (b and c) TEM images of Cr2O3-F-0.5 with different magnifications, (d–k) TEM images of Co/Cr2O3-F-0.5 with different magnifications, and the corresponding dark field elemental mappings. | |
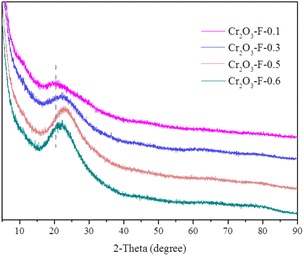 |
| Fig. 2 XRD patterns of samples. | |
XPS was employed to confirm the component Cr2O3-F-0.5 and complete XPS spectra for KIT-6-F for comparison. The full spectra display Si and O signal suggesting the coexisting of two elements in KIT-6-F. In addition to the aforementioned elemental signals, a faint Cr signal is also present in Cr2O3-F-0.5 (Fig. S9†), suggesting the compound is composed of Si, O and Cr elements. As shown in Fig. 3, in the high resolution XPS spectrum of Si 2p, a peak located at 103.5 eV assigns to the Si–O–Si band for KIT-6-F and Cr2O3-F-0.5.39 Notably, high resolution XPS spectrum of Si 2p in Cr2O3-F-0.5 shifts to high binding energy compared with KIT-6-F. Similar phenomenon is observed in O 1s, suggesting the strong interaction between Cr2O3 and KIT-6-F (Fig. S10†). Additionally, high resolution XPS spectrum of Si in KIT-6-E and Cr2O3-E-0.5 also is collected to the effect of P123 on interaction between Cr2O3 and KIT-6. Compared to KIT-6-E, the Si 2p spectra in Cr2O3-E-0.5 undergo a shift to lower binding energy (Fig. S11†). This phenomenon is different from Cr2O3-F-0.5 due to the absence of P123.
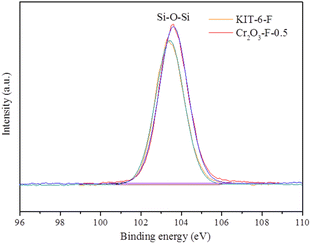 |
| Fig. 3 XPS spectra of Si 2p in Cr2O3-F-0.5 and KIT-6-F. | |
FTIR spectra of KIT-6-F and Cr2O3-F-0.5 were collected to investigate the structure of samples and interaction. As shown in Fig. 4, the bands in two samples at around 1635, 1081, 960, 799 and 464 cm−1correspond to absorbed water, symmetric stretching vibrations of Si–O–Si,–Si–OH vibration, asymmetric stretching vibrations of Si–O–Si, and bending vibrations of Si–O–Si, respectively.40,41 Notably, compared with pristine KIT-6-F, the band at 1
635
960 and 464 cm−1 in compound are red-shifted, indicating the strong interaction between pristine KIT-6-F and Cr2O3, which is facilitated to regulate electron density of catalyst and obtain high performance.42 Combined with the above characterization result, Cr2O3-F-0.5 strong interaction between amorphous Cr2O3 and KIT-6-F had been successfully synthesized. Additionally, The IR spectra of KIT-6-F were collected after five months (Fig. S12†). The result shows no obvious change in characteristic peak is observed, suggesting reserved P123 on the surface of KIT-6 does not leach out.
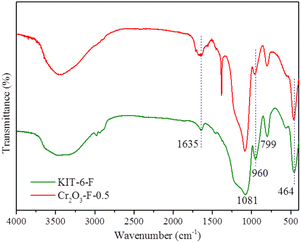 |
| Fig. 4 IR spectra of Cr2O3-F-0.5 and KIT-6-F. | |
UV-vis spectra were conducted to investigate the optical characterization of samples. As shown in Fig. 5, KIT-6-F is not capable of absorbing visible light while after coupling with Cr2O3, visible light absorption capacity is significantly improved and exhibits two absorbing peaks which is similar that of Cr2O3. Absorption strength is increasing with decreasing content of KIT-6-F because large quantities of KIT-6-F can form the light-shield effect and Cr2O3-F-0.3 has the strongest visible light reaction absorption. Notably, Cr2O3-F-0.1 doesn't show the best visible light response. This result may be due to too few KIT-6-F not beneficial to the formation of Cr2O3. Additionally, compared with Cr2O3-F-0.5, the Cr2O3-E-0.5 prepared by calcined KIT-6-F displays weak absorption of visible light, indicating the reserved p123 can strengthen the interaction between KIT-6-F and Cr2O3.
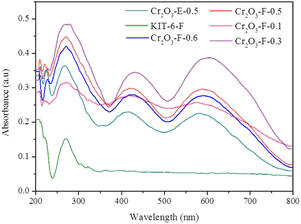 |
| Fig. 5 UV-vis spectra of samples. | |
Separation efficiency of photogenerated electrons and holes has important influence in catalytic performance of catalyst. Transient photocurrent density was conducted to test the effect of reserved P123 on photogenerated carrier separation efficiency of compound. Cr2O3-E-0.5 also was test for comparison. As shown in Fig. 6a, the photogenerative carrier of the Cr2O3-E-0.5 can be effectively separated under visible light irradiation. In contrast, Cr2O3-F-0.5 exhibits stronger signal, revealing that Cr2O3-F-0.5 is benefit to carrier separation and transfer due to the strong interaction between KIT-6 and Cr2O3 with assistance of P123. EIS was performed to confirm the effect of different structure of samples on electron transition. As shown in Fig. 6b, Cr2O3-F-0.5 shows a slightly smaller arcradius, suggesting relatively small resistance to electron transport.
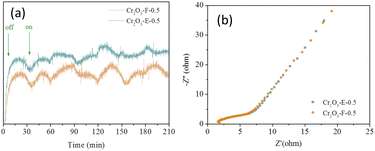 |
| Fig. 6 (a) Profiles of time versus transient photocurrent density of two supports, (b) EIS Nyquist plots of samples. | |
To investigate the effect of reserved P123 on wettability of support, contact angle measurements were conducted for Cr2O3-F-0.5, Cr2O3-E-0.5, Co/Cr2O3-F-0.5 and Co/Cr2O3-E-0.5. Generally speaking, the lower the contact angle is liable to better H2O adsorption. As illustrated in Fig. 7, the Cr2O3-E-0.5 shows the water-droplet contact angle of 17.9° while contact angles of water droplet on the surface of the Cr2O3-F-0.5 decrease values with 11.2°, confirming reserved P123 can enhance the wettability of support and favour the charge transfer and accelerate the rate of NH3BH3 hydrolysis. The contact angles of water droplet on surface of Co/Cr2O3-E-0.5 (28.1°) is larger than Cr2O3-E-0.5. Conversely, the contact angles of water droplet on surface of Co/Cr2O3-F-0.5 (6.1°) is smaller than Cr2O3-F-0.5. The result suggests water can easily spread on the surface of Co/Cr2O3-F-0.5, leading to an increased H2 evolution rate.
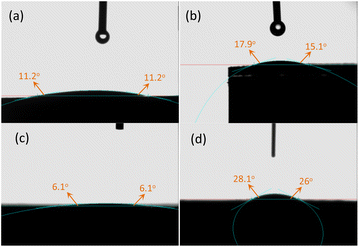 |
| Fig. 7 Water contact angle measurements for (a) Cr2O3-F-0.5, (b) Cr2O3-E-0.5, (c) Co/Cr2O3-F-0.5 and (d) Co/Cr2O3-E-0.5. | |
3.2 Photocatalytic performance
The photocatalytic activity of Co/Cr2O3-F-0.5 was tested in a reactor under visible light irradiation. Additionally, control experiments including Co/KIT-6-F, Co/Cr2O3-F-0.1, Co/Cr2O3-F-0.3, Co/Cr2O3-F-0.5, Co/Cr2O3-F-0.6, Co/Cr2O3-E-0.5 and Co/KIT-6-E were also conducted to reveal the origin of activity of catalyst. As shown in Fig. 8, both Co/KIT-6-F and Co/KIT-6-E exhibits similar activity in dark at same condition (Fig. 8a and b). Importantly, the activity of two catalysts is further boosted under visible irradiation (Fig. 8c and d). The enhanced catalytic performance is mainly attributed to the electronic structure changed induced by interband electron transfer of Co. In sharp contrast, the activity of Co/Cr2O3-F-0.5 exhibits greatly enhanced under visible irradiation than that of Co/KIT-6-F, while slightly boosted in dark. The TOF value of Co/Cr2O3-F-0.5 is 111.1 min−1 which is much higher than that of some tested non-precious metal based catalysts (Table 1). The enhanced performance is primarily attributed to the following aspects: firstly, introduction of Cr2O3 with high visible light utilization ratio induces the generation of photogenerative carriers to provide a greater drive for reaction progression. Secondly, the electron-deficiency degree of Co nanosheets is improved under visible irradiation, resulting in greatly decreased H2O activation and dissociation energy barrier.29 Thirdly, the increased NH3BH3 hydrolysis rate over Co/Cr2O3-E-0.5 under visible irradiation further conform the effect of Cr2O3 on activity of catalyst. Notably, the activity of Co/Cr2O3-E-0.5 with TOF 95.7 min−1 is lower than Co/Cr2O3-F-0.5. Combined with the UV-vis, EIS, transient photocurrent density and water contact angle measurements experimental result, the reserved P123 on surface of KIT-6 not only construct strong interaction among multiple interfaces but also improve catalyst wettability, which is benefit to adsorption of reactant molecules and carrier transfer and accelerate the NH3BH3 hydrolysis rate. Additionally, we added a control experiment over Co supported on Cr2O3-F-0.5-M (physically mixed KIT-6-F and Cr2O3) to provide evidence for the effect of strong interaction between KIT-6-F and Cr2O3 on Co-based catalytic activity. As expected, the performance of Co/Cr2O3-F-0.5-M with TOF of 76.9 min−1 is greatly reduced in comparison to Co/Cr2O3-F-0.5 (Fig. S13†). Therefore, the synergistic effect of high visible light utilization, strong interaction between KIT-6-F and Cr2O3 and improved wettability leads to a significant enhanced catalyst performance under visible irradiation.
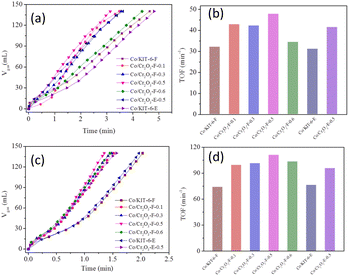 |
| Fig. 8 Plots of time versus volume of H2 evolution from NH3BH3 over Co-based catalysts (a) in dark, (b) corresponding TOF value (c) under visible light irradiation, (d) corresponding TOF value. | |
Table 1 Activities of catalysts in the H2 evolution from NH3BH3
Catalyst |
TOF (min−1) |
References |
Temperature (K) |
Irradiation |
Co/Cr2O3-F-0.3 |
101.4 |
This work |
298 |
With |
Co/Cr2O3-F-0.5 |
111.1 |
This work |
298 |
With |
Co/Cr2O3-F-0.6 |
103.4 |
This work |
298 |
With |
Co/Cr2O3 |
106.8 |
29 |
298 |
With |
NiCo-NC |
35.2 |
43 |
298 |
Without |
Co3O4–SnO2 |
17.6 |
44 |
298 |
Without |
Ni/Ni2P |
68.3 |
4 |
298 |
Without |
Co@N-C-700 |
5.6 |
23 |
298 |
With |
NiCu/CNS |
30.6 |
22 |
298 |
With |
NiCu/SiO2 |
25.3 |
45 |
298 |
Without |
Cu0.5Co0.5O-rGO |
81.7 |
24 |
room |
Without |
Cu/MIL-101 |
28.22 |
46 |
298 |
With |
In order to reveal the influence of interface effect on catalyst performance, the control experiments were performed based on Co/Cr2O3-F-0.1, Co/Cr2O3-F-0.3, Co/Cr2O3-F-0.5, Co/Cr2O3-F-0.6. It can be clearly observed that the introduction of amorphous Cr2O3 in support can slightly boost the activity of Co-based catalyst in dark (Fig. 8c). However, the activity of catalyst containing KIT-6-F can significantly improve under visible irradiation. Moreover, the activity enhances with increased KIT-6-F mass and the catalyst Co/Cr2O3-F-0.5 exhibits highest performance. The activity decreases when the mass of KIT-6-F in support is continued to increase due to light-shield effect.
Photogenerated carriers can not only tune the electronic structure of catalyst but also induce the generation of radical intermediates such as hydroxyl radicals (˙OH), which have significantly effect on the performance of catalyst in light induced reaction. Therefore, we investigated the influence of ˙OH to the performance of Co/Cr2O3-F-0.5 using trapping experiment and EPR characterization. In capture experiment, electrons, holes and ˙OH was captured by K2Cr2O7 (100 μM), KI(100 μM) and 2-propanol (IPA) (100 μL), respectively.47 The result was shown in Fig. 9a and b, the adding of sacrificial agents in reaction system can decrease the hydrolysis rate of NH3BH3, suggesting the photogenerated carriers and radical intermediates can boost the activity of Co/Cr2O3-F-0.5. It is worth noting that the effect of photogenerated carriers and radical intermediates on enhanced activity is different based on different catalyst. ESR measurements was conducted to confirm the existing of ˙OH and superoxide anions (˙O2−) originated from the reaction of dissolved O2 and photogenerated electron was also measured which can improve the rate of NH3BH3 hydrolysis.46 As shown in Fig. 9c, no characteristic signal of ˙OH is observed in the dark based on Cr2O3-F-0.5, but the signal with 1
:
2
:
2
:
1 strength appears under irradiation. The same phenomenon is observed in ˙O2− detection (Fig. 9d). These results further conform the existing of ˙OH and ˙O2− under light irradiation.
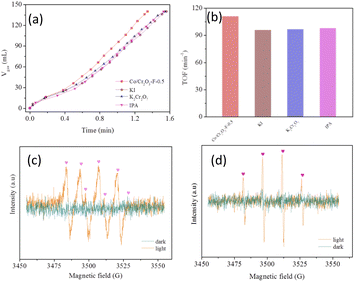 |
| Fig. 9 (a) and (b) Role of hydroxyl radicals and superoxide anions in NH3BH3 hydrolysis underlight irradiation. (c) and (d) DMPO ESR spin-labeling for ˙O2− and ˙OH. | |
The cleavage of O–H bond in H2O molecules is the rate-determining step in NH3BH3 hydrolysis.48,49 To further confirm the conclusion, the kinetic isotope effect (KIE: KH/KD) is conducted. As shown in Fig. 10 the H2 evolution rates greatly decreases using D2O to replace H2O both light irradiation and in dark. The value of KIE is 2.89 in darkness and is 2.13 under light irradiation. This result indicates the cleavage of O–H bond is the rate-determining step over Co/Cr2O3-F-0.5 toward the hydrolysis of NH3BH3.
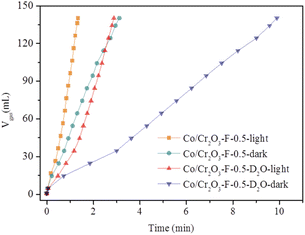 |
| Fig. 10 Plots of time versus volume of hydrogen evolution from NH3BH3 in H2O or D2O over Co/Cr2O3-F-0.5 under visible light irradiation and in dark. | |
According to characterization, experimental results and previous report, we propose a possible mechanism for NH3BH3 hydrolysis induced by visible light. Firstly, Co species and Cr2O3-F-0.5 absorbs visible light. Secondly, photoinduced electrons and holes are generated and transfer to the surface of Co species and Cr2O3-F-0.5. Meanwhile, photoinduced electrons transfer from active species to support then ˙OH and ˙O2− are formed in this process. Thirdly, the B–H bond in NH3BH3 and O–H bond in H2O are broken and H2 is generated. ˙OH and ˙O2− can accelerate the breaking of two kinds of bonds. Notably, the strong interaction among multicomponent and enhanced wettability can favor the transition of photoinduced carriers, ˙OH and ˙O2− and then improve the production rate of H2.
4. Conclusions
We synthesized a hydrophilic support by simple solvent-free thermal method. The compound works as amazing support for Co species. The optimal Co/Cr2O3-F-0.5 exhibits a highest catalytic performance for hydrogen production from NH3BH3 with TOF value of 111.1 min−1. XRD, IR and XPS results indicate strong interaction between KIT-6-F and Cr2O3-F. Contact angle measurement verifies Cr2O3-F-0.5 has improved wettability. The synergistic effect of strong interaction and improved wettability induces high catalytic performance of Co/Cr2O3-F-0.5.
Conflicts of interest
There are no conflicts to declare.
Acknowledgements
The authors gratefully acknowledge the financial support from the National Natural Science Foundation of China (52161038), Young Talents of Science and Technology in Universities of Inner Mongolia Autonomous Region (NJYT23033), Natural Science Foundation of Inner Mongolia Autonomous Region (2022MS05012), and the Talent Development Fund Project of Inner Mongolia Autonomous Region ([2022]-110).
References
- N. Dubouis, A. Serva, R. Berthin, G. Jeanmairet, B. Porcheron, E. Salager, M. Salanne and A. Grimaud, Nat. Catal., 2020, 3, 656–663 CrossRef CAS.
- J. He, Z. Huang, W. Che, X. Xiao, Z. Yao, Z. Liang, L. Zhan, L. Lv, J. Qi, X. Fan and L. Chen, Chem. Eng. J., 2022, 431, 133697 CrossRef CAS.
- M. K. Sarmah, T. P. Singh, P. Kalita and A. Dewan, RSC Adv., 2023, 13, 25253–25275 RSC.
- Y. Lin, L. Yang, H. Jiang, Y. Zhang, D. Cao, C. Wu, G. Zhang, J. Jiang and L. Song, J. Phys. Chem. Lett., 2019, 10, 1048–1054 CrossRef CAS PubMed.
- Y. Meng, Q. Sun, T. Zhang, J. Zhang, Z. Dong, Y. Ma, Z. Wu, H. Wang, X. Bao, Q. Sun and J. Yu, J. Am. Chem. Soc., 2023, 145, 5486–5495 CrossRef CAS PubMed.
- L. Wei, Y. Lu, R. Lua and Y. Cui, RSC Adv., 2023, 13, 7614–7620 RSC.
- C. Wang, J. Tuninetti, Z. Wang, C. Zhang, R. Ciganda, L. Salmon, S. Moya, J. Ruiz and D. Astruc, J. Am. Chem. Soc., 2017, 139, 11610–11615 CrossRef CAS PubMed.
- C. D. Mboyi, D. Poinsot, J. Roger, K. Fajerwerg, M. L. Kahn and J.-C. Hierso, Small, 2021, 17, 2102759 CrossRef CAS PubMed.
- H. Wu, Y. Chen, Y. Fan, X. Lu, L. Li, B. Liu, B. Li and S. Lu, Int. J. Hydrogen Energy, 2020, 45, 30325–30340 CrossRef CAS.
- S. Guan, Y. Liu, H. Zhang, R. Shen, H. Wen, N. Kang, J. Zhou, B. Liu, Y. Fan, J. Jiang and B. Li, Adv. Sci., 2023, 10, 2300726 CrossRef CAS PubMed.
- Y. Ren, J. Duan, X. Liu, L. Bian, Y. Fan and B. Liu, Energy Fuels, 2021, 35, 16222–16231 CrossRef CAS.
- S. Akbayrak and S. Özkar, J. Colloid Interface Sci., 2021, 596, 100–107 CrossRef CAS PubMed.
- L.-T. Guo, Y.-Y. Cai, J.-M. Ge, Y.-N. Zhang, L.-H. Gong, X.-H. Li, K.-X. Wang, Q.-Z. Ren, J. Su and J.-S. Chen, ACS Catal., 2015, 5, 388–392 CrossRef CAS.
- W. Chen, G. Lv, J. Fu, H. Ren, J. Shen, J. Cao and X. Liu, ACS Appl. Mater. Interfaces, 2021, 13, 50017–50026 CrossRef CAS PubMed.
- B. Wang, L. Xiong, H. Hao, H. Cai, P. Gao, F. Liu, X. Yu, C. Wu and S. Yang, J. Alloys Compd., 2020, 844, 156253 CrossRef CAS.
- J. Liao, Y. Wu, Y. Shao, Y. Feng, X. Zhang, W. Zhang, J. Li, M. Wu, H. Dong, Q. Liu and H. Li, Chem. Eng. J., 2022, 449, 137755 CrossRef CAS.
- F. Qiu, X. Hao, W. Huang, Y. Wu, R. Chu, J. Yang, W. Fu, G. Ren, C. Xu and W. Bao, RSC Adv., 2023, 13, 632–637 RSC.
- L. Zhang, J. Ye, Y. Tu, Q. Wang, H. Pan, L. Wu, X. Zheng and J. Zhu, Nano Res., 2022, 15, 3034–3041 CrossRef CAS.
- H. Yin, Y. Kuwahara, K. Mori and H. Yamashita, J. Mater. Chem. A, 2018, 6, 10932–10938 RSC.
- N. Kang, Q. Wang, R. Djeda, W. Wang, F. Fu, M. M. Moro, M. de los A. Ramirez, S. Moya, E. Coy, L. Salmon, J.-L. Pozzo and D. Astruc, ACS Appl. Mater. Interfaces, 2020, 12, 53816–53826 CrossRef CAS PubMed.
- N. Kang, X. Wei, R. Shen, B. Li, E. G. Cal, S. Moya, L. Salmon, C. Wang, E. Coy, M. Berlande, J.-L. Pozzo and D. Astruc, Appl. Catal., B, 2023, 320, 121957 CrossRef CAS.
- S. Zhang, M. Li, L. Li, F. Dushimimana, J. Zhao, S. Wang, J. Han, X. Zhu, X. Liu, Q. Ge and H. Wang, ACS Catal., 2020, 10, 14903–14915 CrossRef CAS.
- H. Wang, Y. Zhao, F. Cheng, Z. Tao and J. Chen, Catal. Sci. Technol., 2016, 6, 3443–3448 RSC.
- H. Zheng, K. Feng, Y. Shang, Z. Kang, X. Sun and J. Zhong, Inorg. Chem. Front., 2018, 5, 1180–1187 RSC.
- H. Zhang, X. Gu, P. Liu, J. Song, J. Cheng and H. Su, J. Mater. Chem. A, 2017, 5, 2288–2296 RSC.
- J. Song, X. Gu, Y. Cao and H. Zhang, J. Mater. Chem. A, 2019, 7, 10543–10551 RSC.
- H. Wu, M. Wu, B. Wang, X. Yong, Y. Liu, B. Li, B. Liu and S. Lu, J. Energy Chem., 2020, 48, 43–53 CrossRef.
- Y. Chen, G. Mao, Y. Tang, H. Wu, G. Wang, L. Zhang and Q. Liu, Chin. J. Catal., 2021, 42, 225–234 CrossRef CAS.
- J. Song and F. Wu, Nanoscale, 2023, 15, 16741–16751 RSC.
- L. Wang and F.-S. Xiao, ChemCatChem, 2014, 6, 3048–3052 CrossRef CAS.
- Y. Li and C. Zhao, ACS Catal., 2017, 7, 2535–2541 CrossRef CAS.
- C. Wang, L. Liu, H. Li, L. Wang and F.-S. Xiao, Matter, 2023, 6, 2697–2710 CrossRef CAS.
- X. Zhang, Y. Zhao, X. Jia, Y. Zhao, L. Shang, Q. Wang, G. I. N. Waterhouse, L.-Z. Wu, C.-H. Tung and T. Zhang, Adv. Energy Mater., 2018, 8, 1702780 CrossRef.
- K. Soni, B. S. Rana, A. K. Sinha, A. Bhaumik, M. Nandi, M. Kumar and G. M. Dhar, Appl. Catal., B, 2009, 90, 55–63 CrossRef CAS.
- H. Al-shaikh, J. Lasri, J. G. Knight and S. T. Al-Goul, Fuel, 2022, 325, 124962 CrossRef CAS.
- P. Liu, X. Gu, H. Zhang, J. Cheng, J. Song and H. Su, Appl. Catal., B, 2017, 204, 497–504 CrossRef CAS.
- J. Song, F. Wu, H. Ma, Y. Liu, N. Song, J. Yu and Y. Wang, ACS Appl. Energy Mater., 2021, 4, 8377–8385 CrossRef CAS.
- J. Pang, S. Jin, J. Hou, G. Wang, K. Sun, Y. Zheng, H. Li, Y. Shen, X. Yang and L. Chen, Sens. Actuators, B, 2023, 374, 132725 CrossRef CAS.
- Y. Ding, J. Wang, M. Liao, J. Li, L. Zhang, J. Guo and H. Wu, Chem. Eng. J., 2021, 418, 129470 CrossRef CAS.
- P. Han, T. Liu, X. Ji and S. Tang, Chin. Chem. Lett., 2018, 29, 1305–1309 CrossRef CAS.
- X. Zhou, H. Zhao, S. Liu, D. Ye, R. Qu, C. Zheng and X. Gao, Appl. Surf. Sci., 2020, 525, 146382 CrossRef CAS.
- P. Zhou, S.-X. Guo, L. Li, T. Ueda, Y. Nishiwaki, L. Huang, Z. Zhang and J. Zhang, Angew. Chem., Int. Ed., 2023, 62, e202214881 CrossRef CAS PubMed.
- L. Zhao, Q. Wei, L. Zhang, Y. Zhao and B. Zhang, Renewable Energy, 2021, 173, 273–282 CrossRef CAS.
- H.-Z. Wang, Y.-X. Shao, Y.-F. Feng, Y.-J. Tan, Q.-Y. Liao, X.-D. Chen, X.-F. Zhang, Z.-H. Guo and H. Li, Rare Met., 2023, 42, 3013–3023 CrossRef CAS.
- K. Guo, Y. Ding, J. Luo, M. Gu and Z. Yu, ACS Appl. Energy Mater., 2019, 2, 5851–5861 CrossRef CAS.
- M. Wen, Y. Cui, Y. Kuwahara, K. Mori and H. Yamashita, ACS Appl. Mater. Interfaces, 2016, 8, 21278–21284 CrossRef CAS PubMed.
- S. Jo, P. Verma, Y. Kuwahara, K. Mori, W. Choi and H. Yamashita, J. Mater. Chem. A, 2017, 5, 21883–21892 RSC.
- S. Zhou, Y. Yang, P. Yin, Z. Ren, L. Wang and M. Wei, ACS Appl. Mater. Interfaces, 2022, 14, 5275–5286 CrossRef CAS PubMed.
- Z. Li, T. He, L. Liu, W. Chen, M. Zhang, G. Wu and P. Chen, Chem. Sci., 2017, 8, 781–788 RSC.
|
This journal is © The Royal Society of Chemistry 2023 |
Click here to see how this site uses Cookies. View our privacy policy here.