Soybean peroxidase immobilised on cellulose-alginate hydrogels for removal of recalcitrant organic pollutants in water†
Received
5th January 2023
, Accepted 31st March 2023
First published on 1st April 2023
Abstract
In recent years, bioremediation has become very attractive for environmental applications, especially enzyme-based treatments, since they offer high catalytic capacity with milder reaction conditions, production of nontoxic compounds, and environmental friendliness. These aspects, together with the increasing attention toward the circular economy approach, encourage many researchers to investigate how to obtain materials with high added value from biomass waste. In this work, we focused our attention on Soybean hulls, which are agro-industrial waste rich in cellulose and soybean peroxidase (SBP), two interesting and versatile components exploitable for environmental treatments. Cellulose containing SBP and alginate were used to prepare fully bio-based hybrid hydrogels tested for removal of bisphenol A, 2,4,6-trichlorophenol and triclosan from both ultrapure and real water spiked solutions. Through both adsorption and enzymatic catalysis, in 5 hours, all contaminants are completely removed from the solution, with a corresponding significant reduction in toxicity. The efficiency of these materials is maintained for eight cycles of reuse and it is also confirmed in a real water matrix. Finally, the burial test in compost soil shows the biodegradability of the hydrogels proving the high eco-compatibility of the whole process.
1. Introduction
The implementation and improvement of urban and industrial water and wastewater treatments are great challenges for researchers, and several strategies have been developed in the past decades to remove pollutants (ozone treatments,1 UV,2 photocatalysis, biological treatments,3etc.4). Unfortunately, some recalcitrant contaminants, known as Contaminants of Emerging Concerns (CECs), are not abated by common treatments and are released in surface water used for irrigation or in aquaculture farms.5 Thus, the development of new treatments aims to preserve not only the right to clean water but also to safe food and wellness of every human being (UN 2030 Agenda for Sustainable Development).6 The raised complexity of the problem also presupposes a greater complexity of the effective strategies to adopt, which must be increasingly focused on their impact on the environment and society. Many integrated strategies, aimed at improving the performance of individual treatments and broadening the target of contaminants, have been developed in recent years. Among these, hybrid materials and nanomaterials are becoming more and more popular,7 and the choice of feedstock for their synthesis often falls on biopolymers-derivatives or other organic components that can be obtained from waste valorisation, for a circular economy approach. The introduction of enzymatic components in these materials' structures is very common. Indeed, immobilisation can stabilise enzymes and enables the exploitation of their catalytic capabilities for multiple processing cycles, although it often results in a reduction in activity.8,9 In this work, we recovered Soybean hulls, which are usually considered a putrescible waste, exploiting soybean peroxidase and the residual cellulose, to prepare a fully bio-based hybrid material. Soybean peroxidase (SBP) is a glycoprotein whose catalytic cycle is activated by the presence of hydrogen peroxide and which is able to oxidise many substances, including chlorinated phenolic compounds and different dyes.10–13 Moreover, this protein is very interesting because it maintains its catalytic activity over a wide range of pH and temperature.14,15 Cellulose, which makes up about 40–50% of Soybean hulls,16 is a biopolymer suitable for various chemical modifications, for different environmental applications and also for enzyme immobilization.17–19 Herein, hybrid hydrogels were obtained by introducing SBP immobilised on chemically modified-cellulose inside sodium alginate beads. These materials were characterised and tested towards three different organic contaminants, triclosan (antibacterial and antifungal), bisphenol A (xenoestrogen and plasticiser), and 2,4,6-trichlorophenol (present in antiseptics, general pesticides, bactericides, fungicides, herbicides, insecticides, germicides, and wood and glue preservatives), already studied as SBP substrates in solution.20–22 Hydrogels' capability in contaminant removal was also evaluated in spiked pond water for goldfish and carp aquaculture to explore their possible real applications.
2. Experimental
2.1. Materials and instruments
All chemical reagents are in analytical grade and used without purification. Fresh yellow soybean (Glycine max) seeds were purchased by Del Prete s.r.l. (Fondi, LT, Italy) and stored at room temperature before use. Compost Florawiva® was provided by ACEA Pinerolese (Pinerolo, TO, Italy). The pond water was taken from goldfish and carp aquaculture basins in the Tetto Frati Agrozootechnology Centre, Department of Agricultural and Forestry Sciences, University of Torino (Italy). Contaminants removal was evaluated using a Merck-Hitachi HPLC equipped with a LiChrospher RP-C18 reverse phase column (5 μm, 4 mm i.d. × 125 mm long, Merck) and a Hitachi L-4200 UV-visible detector. The morphology of hydrogels was examined using an EVO10 Scanning Electron Microscope (SEM, Carl Zeiss Microscopy GmbH) with an acceleration voltage of 20 kV. The samples were sputter-coated with a 20 nm-thick gold layer in rarefied argon, using a Quorum SC7620 Sputter Coater. Enzyme kinetics were carried out in a Thermospectronic UNICAM UV-300 dual-beam spectrophotometer equipped with magnetic stirring and temperature control module. Non-purgeable organic carbon of real aqueous samples was measured by TOC-VCSH analyser. The toxicity of reaction products was evaluated following the evolution of bioluminescence of Vibrio fischeri bacteria in a Microtox Model 500 Toxicity Analyzer (SDI Europa) and analysed with the APAT-IRSA 8030 method.23
2.2. Extraction of soybean peroxidase and cellulose
Soybean peroxidase (SBP) was extracted from fresh soybean hulls and successively purified following the method previously reported by Calza et al.,11 which can be summarised as follows: i) extraction with phosphate buffer at pH 7; ii) concentration by tangential flow filtration and precipitation by ammonium sulphate; iii) recovery of the precipitate, dissolution in phosphate buffer at pH 7 and dialysis against the same buffer; iv) final protein separation by ionic exchange chromatography in KCl gradient. After SBP extraction, soybean hulls were recovered and dried at 60 °C for 2 hours and then left at room temperature for almost 24 h. Cellulose was isolated from dried soybean peels according to a general method previously proposed in the literature.24,25 After the initial size reduction, the material was treated with a 2% w/v sodium hydroxide solution (solid–liquid ratio 1
:
10) for two hours at 80 °C, then washed with distilled water up to a neutral pH value and dried at 60 °C. Successively, the pre-treated pulp was subjected to acid hydrolysis with 1 M HCl (solid–liquid ratio 1
:
10) at 80 °C for two hours, then washed with distilled water up to a neutral pH and dried at 60 °C. Finally, the pulp was treated once more with the 2% w/v of NaOH solution (solid–liquid ratio 1
:
10), washed and dried again to obtain the final product. The weight loss after each treatment was about 30% for both the alkaline steps and 15% for the acid one.
2.3. Immobilisation of SBP on extracted cellulose
Immobilisation of SBP on cellulose fibres was carried out by adapting a method previously developed for immobilisation of the same protein on silica monoliths.9,26 One gram of extracted cellulose was suspended in 0.08 L of a 10% v/v aqueous solution of 3-aminopropyltriethoxysilane (APTES). The pH was adjusted to 4.0 with HCl and the mixture was heated under stirring at 80 °C for 3 h in a water bath. The resulting suspension was filtered on a Buchner funnel and the product was washed with bidistilled water and dried at 60 °C. This procedure was repeated twice in order to maximise the reaction yield. Successively, 0.5 g of silanised product were suspended in 0.05 L of glutaraldehyde 2.5% (v/v) in 0.1 M phosphate buffer solution at pH 7.0 and left under stirring in the dark for 1 h at room temperature. The resulting suspension was then filtered, and the solid powder was washed and added to 0.015 L of SBP 0.13 mg mL−1 solution in 0.1 M phosphate buffer at pH 7.5 and left to react at 4 °C overnight under gentle stirring. The amount of the immobilised enzyme was evaluated by difference from the starting solution using UV-visible analysis. The enzyme concentrations before and after immobilisation were calculated following an established method on the basis of the absorbance at 403 nm, typical of SBP Fe(III)-heme group, by using 94
600 M−1 cm−1 as molar extinction coefficient.14
2.4. Hydrogels preparation
Three different kinds of hydrogels containing alginate were prepared, namely HY-A (alginate hydrogels), HY-AC (alginate hydrogels with dispersed cellulose), HY-AC-SBP (alginate hydrogels with dispersed cellulose functionalised with SBP). To synthesise HY-A, 75 mg of alginate were dissolved in 3 ml of distilled water under stirring and then dripped in a CaCl2 solution (0.2 M).27–29 The obtained spherical hydrogels were left in the gelling solution for around 30 min and then filtered and washed. To synthesise the other types of hydrogels, 75 mg of alginate were dissolved into 3 ml of distilled water, and then 200 mg of cellulose (with or without loaded enzyme) were dispersed in the viscous solution by sonicating. These viscous suspensions were dripped in a CaCl2 solution (0.2 M). The obtained hydrogels were left in the gelling solution for around 30 min and then filtered and washed. All the hydrogels were then stored at 4 °C until use. The swelling behaviour of the hydrogels, due to water uptake, was investigated using a gravimetric method. Oven-dried hydrogels were placed in deionised water at room temperature and the weight increase was monitored by removing gels from the water and weighing them after a quick removal of water from the surface with filter paper. The swelling ratio percentage (Sw%) was calculated with the eqn (I)30–32 where Ws and Wd are the weight of the swollen and dry samples, respectively. | 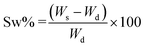 | (I) |
2.5. Enzyme activity
The activity of both free and immobilised SBP was evaluated by the H2O2/DMAB-MBTH system: in a solution containing 3-(dimethylamino)benzoic acid (DMAB, 5 × 10−4 M), 3-methyl-2-benzothiazolinonehydrazone (MBTH, 2 × 10−5 M), and H2O2 (1 × 10−4 M) in acetate buffer 0.1 M pH 5.5, one hydrogel or 5 mg of functionalised cellulose or 5 μL of SBP solution were added in order to introduce similar amount of SBP (0.018 ± 0.002 mg).33 The enzyme activity was calculated with the initial rate method following the increase in absorbance at 590 nm of the reaction product.34
2.6. Real water sampling and pre-treatment
A real pond water sample was collected in March 2022. Sampled water was collected in amber glass bottles, filtered with 70 mm glass microfibers filters (VWR) and stored at 4 °C. Hardness, pH and non-purgeable organic carbon (NPOC) of real aqueous samples were measured. The water had a pH of 8 and a hardness of 100, expressed in mg L−1 of CaCO3. The organic matter content, assessed by a NPOC analysis, was 150 mg L−1. Before spiking with the three organic contaminants, the water was analysed by HPLC-UV under the same chromatographic conditions described in section 2.7 and no signal were detected at the retention time of the pollutants.
2.7. Test on pollutants removal
Hydrogels efficiency in the removal of organic pollutants in water was evaluated by using 2,4,6-trichlorophenol (TCP), bisphenol-A (BPA) and triclosan (TCS) in single solutions at a concentration of 5 ppm and in mixtures at a concentration of 1.67 ppm (total concentration 5 ppm) as substrates. All the tests were performed both in ultrapure and real pond water. The removal of contaminants was evaluated by HPLC-UV analysis. Elution was carried out with acetonitrile and phosphate buffer (1 × 10−2 M) at pH 2.8 (70
:
30% v/v) at a flow rate of 1 mL min−1. The analytical detector wavelength was set at 275 nm for BPA and 242 nm for TCP, and TCS. In the case of the analysis of the pollutants mixture, a two-step gradient starting from 45% v/v to 70% v/v of acetonitrile in 3 min followed by 5 min of isocratic elution was carried out. In each experiment, 4 HY-AC-SBP hydrogels were added to a solution (5 mL) containing a single contaminant or the whole mixture. The removal of each pollutant was followed for 5 hours in the presence of an initial H2O2 concentration of 1 × 10−4 M. For comparison, similar measurements were carried out with SBP in solution at almost the same concentration introduced into the hydrogels. In addition, to evaluate the extent of compounds' adsorption, other experiments were performed only in the presence of hydrogels. Moreover, to determine the possible non-enzymatic oxidation of the substrates, preliminary tests were performed in the presence of H2O2 but without hydrogels. The possible reuse of hydrogels was also investigated: after the first contaminants removal experiment, hydrogels were washed with distilled water, the excess surface water was removed with filter paper, and they were used again for another reaction cycle. A total of 8 subsequent cycles of treatment were performed.
2.8. Biodegradation tests
A plastic box (15 × 20 × 10 cm) was filled with Florawiva® compost and hydrogels were buried at a depth of 4 cm from the soil surface to ensure aerobic degradation conditions. The box was stored at room temperature (23 ± 2 °C) and the soil humidity was maintained at 30% by spraying water regularly on the surface. Fluctuations in soil moisture were followed gravimetrically using the standard method of oven drying (ASTM D2216). Comparative tests were also carried out in sterilised soil by burying the hydrogels in compost previously heat-treated at 105 °C for almost 24 hours.35–38
3. Results and discussions
Alginate-based hydrogels containing dispersed cellulose functionalised with SBP (HY-AC-SBP) were prepared, characterised, and tested in the abatement of three organic pollutants. As reference materials, simple alginate hydrogels (HY-A) and alginate hydrogels with dispersed cellulose (HY-AC) were also synthesised.
3.1. Hydrogels preparation and characterisation
For HY-AC-SBP preparation (Fig. 1), cellulose extracted from soybean hulls was chemically modified, introducing amino groups by reaction with APTES.39 As described before, a double silanisation reaction was carried out in order to obtain the highest amount of amino groups available for further reaction. The subsequent functionalisation with SBP was obtained by the formation of Schiff bases between amino groups, introduced on the cellulose and present in enzyme structure, and aldehydic groups of glutaraldehyde, using a method previously reported for the immobilisation of the same protein on silica particles.9,26 The total amount of soybean peroxidase loaded is 3.3 mg g−1. HY-AC-SBP hydrogels were formed by dropping a suspension containing alginate and functionalised cellulose in a calcium chloride solution. The so obtained beads are characterised by a diameter of 4–5 mm and an average weight of 35–45 mg. Each bead contains an average amount of 6.5 ± 0.5 mg of functionalised cellulose (corresponding to 0.023 ± 0.002 mg of SBP).
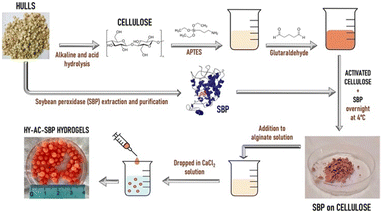 |
| Fig. 1 Summary of HY-AC-SBP hydrogels preparation. | |
For comparison, hydrogels containing only alginate (HY-A) and alginate and unmodified cellulose (HY-AC) were prepared with the same method.
The bead bulk of HY-AC and HY-AC-SBP hydrogels was investigated by SEM analysis. In Fig. 2, both hydrogel morphologies evidence the prevalence of cellulose fibres, which are well dispersed in the whole gel matrix and whose shape is in good agreement with the literature.24,40 No significant differences were detected due to the chemical modification related to SBP loading on the biopolymer. From the detail in Fig. 2B, it is also possible to note bundles of fibres that are not completely separated, probably due to the residual lignin and hemicellulose not totally removed during the extraction process (irrelevant in the functionalisation reaction).
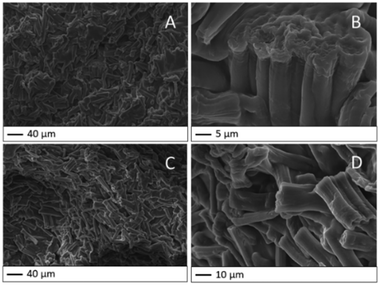 |
| Fig. 2 SEM images of hydrogels. (A) HY-AC (hydrogel containing cellulose fibres); (B) HY-AC detail of fibres not completely separated; (C) HY-AC-SBP (hydrogel containing cellulose fibres with enzymes); (D) detail of HY-AC-SBP. | |
A typical hydrogels' property is water uptake,32,41 depending on their structure. Therefore, different behaviour of synthesized hydrogels was investigated using the gravimetric method described in section 2.4. The corresponding swelling ratio percentages at different contact times with water were calculated with eqn (I) and shown in Fig. 3.
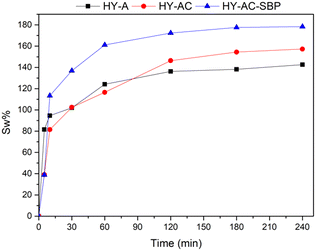 |
| Fig. 3 Swelling ratio of different kind of hydrogels. | |
Experimental data evidence that all the samples reach an equilibrium condition after 4 hours, with swelling ratio values higher than 100%. The presence of cellulose appears to increase the swelling capacity of the material; this behaviour has already been observed in other studies and ascribed to the hydrophilic characteristics of cellulose.42,43 On the other hand, soybean peroxidase is a water-soluble globular protein able to form electrostatic interactions with the surrounding environment.44 Indeed, the highest swelling percentage occurs with the introduction of chemically modified cellulose and SBP in HY-AC-SBP (about 178%). Moreover, electrostatic interactions between cellulose and other molecules modify the gelly structure increasing the mesh size of the network.45,46 Therefore, a high swelling ratio can also promote better contact between aqueous contaminants and hydrogel active phase.
In order to evaluate the residual activity of SBP encapsulated in HY-AC-SBP, a comparison between the activity of SBP in solution loaded on cellulose and incorporated in one hydrogel was made by using the DMAB/MBTH method. According to the literature,9 immobilisation leads to a significant reduction of enzyme activity and the loss of activity is also confirmed in the case of HY-AC-SBP. Still, the system is stable and the enzyme activity is maintained for almost 10 reaction cycles (Fig. S1†).
3.2. Pollutants removal
HY-AC-SBP hydrogels were used to remove three organic pollutants, 2,4,6-trichlorophenol (TCP), Bisphenol-A (BPA) and Triclosan (TCS), both in ultra-pure and pond water (Fig. 4).
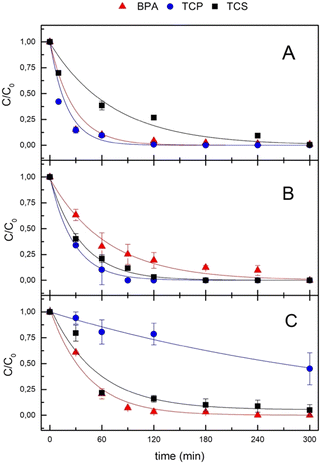 |
| Fig. 4 Removal of BPA, TCP and TCS with HY-AC-SBP. (A) Enzymatic removal of single contaminant solutions in ultrapure water; (B) enzymatic removal of contaminants mixture in ultrapure water; (C) enzymatic removal of contaminants mixture in real water (pond water). | |
The experiments were carried out both in a single contaminant solution and in a mixture of the three compounds in order to mimic possible interactions between them in a more complex system.
Preliminary tests were carried out to evaluate the contribution of the adsorption of pollutants on HY-AC-SBP hydrogels. Moreover, their possible oxidation by H2O2 only was also tested before studying the removal by the final HY-AC-SBP/H2O2 system. The results obtained after 5 hours of treatment in ultra-pure water are summarised in Table 1.
Table 1 Removal percentage due to different processes after 5 hours of treatment in ultra-pure water
|
|
Abatement% (H2O2 only) |
Adsorption (%) (HY-AC-SBP) |
Removal% (HY-AC-SBP + H2O2) |
Time of complete removal (min) |
k
obs (min−1) |
Half-life time (min) |
Single contaminants |
BPA |
0.7% |
20% |
100% |
200 |
0.037 ± 0.003 |
18.0 ± 1.6 |
TCP |
2.4% |
42% |
100% |
120 |
0.049 ± 0.010 |
14.0 ± 2.8 |
TCS |
10% |
62% |
100% |
300 |
0.014 ± 0.001 |
50.0 ± 5.0 |
Mix of contaminants in ultra-pure water |
BPA |
10% |
28% |
100% |
300 |
0.015 ± 0.001 |
46.0 ± 4.0 |
TCP |
13% |
38% |
100% |
120 |
0.037 ± 0.001 |
19.0 ± 0.3 |
TCS |
16% |
43% |
100% |
180 |
0.027 ± 0.001 |
26.0 ± 1.2 |
Experimental data show as the oxidation by hydrogen peroxide in the absence of SBP is negligible for BPA and limited for TCS and TCP when they are alone. Still, a slightly higher effect is observed when they are in the mixture, with a removal of 10–16% after 5 hours of reaction (Fig. S2†). The contribution of adsorption to pollutants removal by HY-AC-SBP is more consistent and shows a similar trend (BPA < TCP < TCS) both in single solutions and in the mixture (Fig. S3†). This behaviour agrees with the increasing lipophilicity of the three compounds, in terms of their log
P values (3.32, 3.69, 4.76 respectively47).
More interesting results were obtained with HY-AC-SBP hydrogels in the presence of H2O2, when SBP catalytic cycle occurs, since the experimental data show the complete removal of all the contaminants in 5 hours, both in a single-component solution and in the mixture (Fig. 4A and B).
Pollutants abatement can be assimilable to a pseudo-first-order decay and the different reaction rates can be due to distinct affinities of substrates for the enzyme. The corresponding kinetic constants (kobs), together with the calculated half-life times (t1/2), are reported in Table 1. TCP shows the shortest half-life time, with similar values both in single and in the mixture (15 and 19 min, respectively), while BPA and TCS reaction rates are significantly influenced by the presence of the other contaminants. Indeed, BPA half-time life increases from 18 to 46 min, whereas that of TCS decreases from 50 to 26 min (results for single solution and mixture, respectively). These effects can be related to differences in the reaction mechanism. Indeed, according to the literature, peroxidases induce the formation of phenoxy radicals which subsequently leave the active site of the enzyme and undergo different fates depending on their structure (Fig. S4†). In the case of TCP, the intermediate is stabilised by the substitution of one chlorine atom with an oxygen and the formation of the stable product 2,6-dichloro-1,4-benzoquinone.48,49 On the contrary, in the case of BPA and TCS, the first radical intermediate generates a mixture of substances at higher molecular weight deriving from the coupling with parent molecules or secondary radicals.50,51 In the latter case, interferences due to the contemporary presence of these species generated by TCS and BPA could explain the differences in reaction rates observed in the experiments with the mixture of pollutants. The removal rate trend (TCP > BPA ≫ TCS) in the single solution is also in agreement with analogous test carried out with free SBP, in which calculated half-life times are 5.7, 6.4 and 79 min, respectively (Fig. S5†).
In order to investigate the possibility of using HY-AC-SBP hydrogels to treat actual water, spiked pond water from aquaculture was also used. The complexity of the real matrix does not influence the physical removal of contaminants. In fact, adsorption values follow a similar trend to that measured in ultrapure water (Table 2): physical removal is higher for TCS, while for TCP and BPA, it is similar.
Table 2 Removal percentage due to different processes after 5 hours of treatment in real pond water
|
Adsorption (%) (HY-AC-SBP) |
Removal (%) (HY-AC-SBP + H2O2) |
Time of complete removal (min) |
k
obs (min−1) |
Half-life time (min) |
BPA |
36% |
100% |
240 |
0.0230 ± 0.002 |
30.0 ± 2.3 |
TCP |
35% |
55% |
— |
0.0026 ± 0.0002 |
— |
TCS |
64% |
96% |
∼300 |
0.018 ± 0.002 |
39.0 ± 5.0 |
In contrast, removal due to enzymatic catalysis in the real matrix is slower and undergoes significant changes, as shown in Fig. 4C and Table 2. Nevertheless, BPA and TCS are completely removed after 5 hours with similar trends (half-life times are 30 and 40 minutes, respectively), whereas TCP is only reduced to 55%. To better understand the reason for the uncompleted TCP removal, we carried out additional measurements with different concentrations of H2O2 and at different pH values in ultra-pure water (Fig. S6†). Both factors appear not to significantly affect the removal of TCP, so implying that the reduced abatement could be attributable to the inorganic and organic matter present in pond water52,53 and the possible inhibition of SBP, as already observed in previous studies concerning the degradation of pentachlorophenol in tap water.33
3.3. Hydrogel reuse
The possible reuse of HY-AC-SBP for further contaminants removal experiments was tested by undergoing hydrogels to 8 reaction cycles in ultra-pure water. Although a slight decrease in the removal rate of contaminants can be observed after 120 min of treatment, the compounds are completely abated after 300 min of treatment also in the eighth reaction cycle (Fig. S7†), so indicating that the system maintains a high efficiency and stability even after repeated use (Fig. 5). This fact also emerges when comparing the kinetic parameters calculated for the first and eighth cycles (Table S1†). The kobs values decrease whereas half-life times increase, but this effect does not significantly influence the possibility to obtain the complete removal of the pollutants at the end of the treatments.
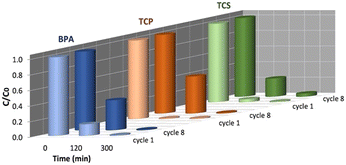 |
| Fig. 5 Different results in BPA, TCP and TCS removal at 120 and 300 min. Comparison between the first and eighth cycle of HY-AC-SBP treatment. | |
3.4. Toxicity test
Acute toxicity of contaminants mixture as a function of reaction time, was evaluated in ultrapure water by following the bioluminescence produced by Vibrio fischeri bacteria (Fig. 6). The parent compounds exhibit a high toxicity, as assessed by the very high percentage of inhibition effect (close to 100%) at 0 min. The progressive decrease in the pollutants concentration during the reaction with HY-AC-SBP led to a consistent decrease in toxicity, as the luminescence inhibition percentage is reduced to about 21% after 300 min. These results suggest that the removal of the initial pollutants proceeded through the formation of less hazardous compounds, in agreement with literature data showing the lower toxicity of the reaction products with respect to the parent molecules.50,54–56
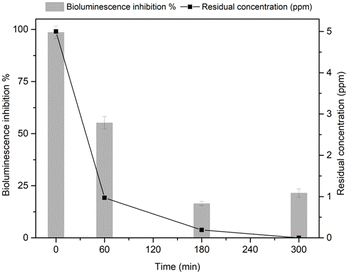 |
| Fig. 6 Comparison between the residual total concentration of BPA, TCP and TCS and bioluminescence inhibition percentage as function of reaction time with the HY-AC-SBP/H2O2 system. | |
3.5. Biodegradation test
One of the advantages of using bio-based materials for the remediation of contaminated environmental matrices is a possible biological treatment for their disposal. For this purpose, we subjected the hydrogels to preliminary biodegradation tests conducted by burial in Florawiva® compost. For comparison, control experiments were also carried out in sterilised soil. An experimental setup was realised, taking into account previous studies for similar materials.35–38
Fig. 7A–C show the macroscopic changes that occurred to HY-AC-SBP hydrogels during 20 days of burial. It is noteworthy that the action of soil microorganisms and the formation of mould on the surface of the beads is clearly visible after only 4 days. Other modifications can also be observed by handling the materials, which became less compact and easily broke when taken with tweezers. On the other hand, in sterilised soil, mould formation did not occur, and the hydrogels seem to keep their structure unchanged (Fig. 7D).
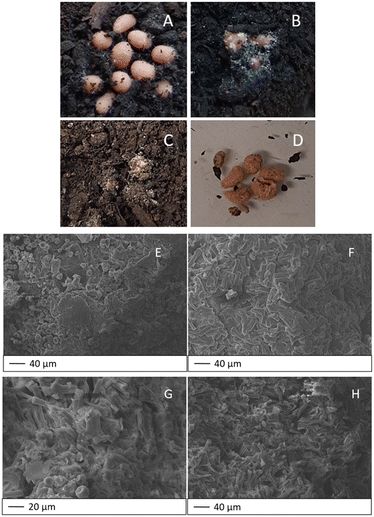 |
| Fig. 7 (A) HY-AC-SBP after 4 days of burial; (B) HY-AC-SBP after 7 days of burial; (C) HY-AC-SBP after 20 days of burial; (D) HY-AC-SBP in sterilised soil after 20 days of burial; (E) SEM image of HY-AC-SBP surface after 20 days of burial in compost; (F) SEM image of HY-AC-SBP surface after 20 days of burial in sterilised soil; (G) SEM image of HY-AC-SBP bulk after 20 days of burial in compost; (H) SEM image of HY-AC-SBP bulk after 20 days of burial in sterilised soil. | |
Fig. 7E shows the surface morphology of HY-AC-SBP after 20 days of burial in compost, confirming the strong adhesion of the compost matter on the beads. On the contrary, in the case of the sterilised soil, the surface of the beads appears free of extraneous particles (Fig. 7F).
Analysing the bead bulk, the cellulose fibres within the gel matrix, both in contact with compost (Fig. 7G) and with sterilised soil (Fig. 7H), maintain a pretty unvaried morphology after 20 days of burial. The foreign particles present in Fig. 7G are probably due to residual compost transferred during the sample pre-processing for SEM analysis.
From these preliminary results, we can infer that these fully bio-based materials are clearly subjected to the action of soil microorganisms, but in this case the common visible effects of surface erosion and formation of holes and cracks in the internal structure reported in the literature57,58 seem not to be applicable. Although the presence of a massive amount of cellulose fibres and stuck compost particles hinders the possibility of detecting those damages in the hydrogel matrix, SEM images show as the microorganism action initially affects the most superficial part of the hydrogels and only later their internal structure. However, further studies at longer burial times are needed to verify the mechanism of complete biodegradation of the hydrogels.
Conclusions
In this work, we demonstrated the possibility of valorising a widely spread agro-industrial waste, soybean hulls, using it as a starting point to obtain efficient materials for the treatment of water contaminated by organic compounds.
We synthesised and characterized hydrogels containing immobilised soybean peroxidase on cellulose, studying their removal efficiency towards triclosan, bisphenol A and 2,4,6-trichlorophenol, separately and in a mixture.
With these bio-based hydrogels, the removal can be ascribed to two different processes: adsorption, due to the presence of alginate and cellulose that are biosorbents,59–61 and enzymatic catalysis promoted by the immobilised SBP.
Both the extent of adsorption and the rates of contaminant abatement depend on the nature of the pollutants and their affinity for the enzyme. Experimental data show different trends for the two phenomena; however, the combination of the two effects led to the complete removal of BPA, TCP and TCS after five hours of treatment, both in the single solution and in their mixture.
We also demonstrate that this system maintains a high removal efficiency also after 8 cycles of reuse and that the presence of organic matter in pond water does not significantly affect the possibility to obtain the complete removal of pollutants.
These fully bio-based hydrogels are easily and quickly degraded in soil by microorganisms' action. These encouraging biodegradation results also suggest a possible environmentally friendly end of life of these materials guaranteeing a high eco-compatibility of the whole process.
Author contributions
Conceptualisation: P. Calza and E. Laurenti; data curation: M. Rigoletto; investigation: M. Rigoletto, V. Sederino, A. Santuchi da Cunha, D. Fabbri, M. L. Tummino; writing – original draft: M. Rigoletto and E. Laurenti writing – review & editing: E. Laurenti and P. Calza.
Conflicts of interest
There are no conflicts to declare.
Acknowledgements
This paper is part of a project that has received funding from the European Union's Horizon 2020 research and innovation programme under the Marie Skłodowska-Curie grant agreement n. 101007578 (SusWater). The authors acknowledge support from Project CH4.0 under MUR (Italian Ministry for the University) program “Dipartimenti di Eccellenza 2023-2027” (CUP: D13C22003520001). Acea Pinerolese S.p.A. is gratefully acknowledged for the supply of Florawiva® compost.
Notes and references
- J. Wang and H. Chen, Sci. Total Environ., 2020, 704, 135249 CrossRef CAS PubMed.
- Y. Zhang, Y.-G. Zhao, F. Maqbool and Y. Hu, J. Water Process Eng., 2022, 45, 102496 CrossRef.
- A. Daverey, D. Pandey, P. Verma, S. Verma, V. Shah, K. Dutta and K. Arunachalam, Bioresour. Technol. Rep., 2019, 7, 100252 CrossRef.
- G. Crini and E. Lichtfouse, Environ. Chem. Lett., 2019, 17, 145–155 CrossRef CAS.
- B. S. Rathi, P. S. Kumar and P.-L. Show, J. Hazard. Mater., 2021, 409, 124413 CrossRef CAS PubMed.
-
Transforming our world: the 2030 Agenda for Sustainable Development | Department of Economic and Social Affairs, https://sdgs.un.org/2030agenda, (accessed December 5, 2022) Search PubMed.
- M. Rigoletto, P. Calza, E. Gaggero and E. Laurenti, Chem. Eng. J. Adv., 2022, 10, 100252 CrossRef CAS.
- R. A. Sheldon and S. van Pelt, Chem. Soc. Rev., 2013, 42, 6223–6235 RSC.
- T. Marchis, G. Cerrato, G. Magnacca, V. Crocellà and E. Laurenti, Biochem. Eng. J., 2012, 67, 28–34 CrossRef CAS.
- T. Marchis, P. Avetta, A. Bianco-Prevot, D. Fabbri, G. Viscardi and E. Laurenti, J. Inorg. Biochem., 2011, 105, 321–327 CrossRef CAS PubMed.
- P. Calza, D. Zacchigna and E. Laurenti, Environ. Sci. Pollut. Res., 2016, 23, 23742–23749 CrossRef CAS PubMed.
- V. Katheresan, J. Kansedo and S. Y. Lau, J. Environ. Chem. Eng., 2018, 6, 4676–4697 CrossRef CAS.
- Q. Husain, Rev. Environ. Sci. Biotechnol., 2010, 9, 117–140 CrossRef CAS.
- J. K. A. Kamal and D. V. Behere, Biochemistry, 2002, 41, 9034–9042 CrossRef CAS PubMed.
- B. Boscolo, E. Laurenti and E. Ghibaudi, Protein J., 2006, 25, 379–390 CrossRef CAS PubMed.
- M. L. Tummino, V. Tolardo, M. Malandrino, R. Sadraei, G. Magnacca and E. Laurenti, Front. Chem., 2020, 8, 763 CrossRef CAS PubMed.
- F. Zhang, M. Lian, A. Alhadhrami, M. Huang, B. Li, G. A. M. Mersal, M. M. Ibrahim and M. Xu, Adv. Compos. Hybrid Mater., 2022, 5, 1852–1864 CrossRef CAS.
- S. Sulaiman, M. N. Mokhtar, M. N. Naim, A. S. Baharuddin and A. Sulaiman, Appl. Biochem. Biotechnol., 2015, 175, 1817–1842 CrossRef CAS PubMed.
- Y. Liu and J. Y. Chen, J. Bioact. Compat. Polym., 2016, 31, 553–567 CrossRef CAS.
- M. Bilal, D. Barceló and H. M. N. Iqbal, Sci. Total Environ., 2020, 735, 139194 CrossRef CAS PubMed.
- M. Sarro, N. P. Gule, E. Laurenti, R. Gamberini, M. C. Paganini, P. E. Mallon and P. Calza, Chem. Eng. J., 2018, 334, 2530–2538 CrossRef CAS.
- A. S. da Cunha, A. dos S. Vianna Jr. and E. Laurenti, Braz. J. Chem. Eng., 2021, 38, 719–730 CrossRef CAS.
-
Redazione, Risorse e servizi - L13 - manuale dei metodi, https://www.irsa.cnr.it/wp/?page_id=5435, (accessed December 23, 2022) Search PubMed.
- A. Alemdar and M. Sain, Bioresour. Technol., 2008, 99, 1664–1671 CrossRef CAS PubMed.
- A. Sinclair, L. Jiang, D. Bajwa, S. Bajwa, S. Tangpong and X. Wang, J. Appl. Polym. Sci., 2018, 135, 46304 CrossRef.
- G. Magnacca, E. Laurenti, E. Vigna, F. Franzoso, L. Tomasso, E. Montoneri and V. Boffa, Process Biochem., 2012, 47, 2025–2031 CrossRef CAS.
- S. Farias, D. A. Mayer, D. de Oliveira, S. M. A. G. U. de Souza and A. A. U. de Souza, Appl. Biochem. Biotechnol., 2017, 182, 1290–1306 CrossRef CAS PubMed.
- I. Alemzadeh and S. Nejati, Iran. J. Chem. Chem. Eng., 2009, 28, 43–49 CAS.
- Y. Fei, Y. Li, S. Han and J. Ma, J. Colloid Interface Sci., 2016, 484, 196–204 CrossRef CAS PubMed.
- J. Tavakoli, E. Laisak, M. Gao and Y. Tang, Mater. Sci. Eng., C, 2019, 104, 109951 CrossRef CAS PubMed.
- A. Dodero, L. Pianella, S. Vicini, M. Alloisio, M. Ottonelli and M. Castellano, Eur. Polym. J., 2019, 118, 586–594 CrossRef CAS.
- C. Noè, C. Tonda-Turo, A. Chiappone, M. Sangermano and M. Hakkarainen, Polymers, 2020, 12, 1359 CrossRef PubMed.
- V. Tolardo, S. García-Ballesteros, L. Santos-Juanes, R. Vercher, A. M. Amat, A. Arques and E. Laurenti, Water, Air, Soil Pollut., 2019, 230, 140 CrossRef.
- T. T. Ngo and H. M. Lenhoff, Anal. Biochem., 1980, 105, 389–397 CrossRef CAS PubMed.
- B. Deepa, E. Abraham, L. A. Pothan, N. Cordeiro, M. Faria and S. Thomas, Materials, 2016, 9, 50 CrossRef PubMed.
- P. Dalev, E. Staromanova, D. Dalev, R. D. Patil, J. E. Mark, E. Vassileva and S. Fakirov, Biotechnol. Biotechnol. Equip., 2001, 15, 116–123 CrossRef CAS.
- H. Doh, K. D. Dunno and W. S. Whiteside, Food Biosci., 2020, 38, 100795 CrossRef CAS.
- J. F. Martucci and R. A. Ruseckaite, Polym. Degrad. Stab., 2009, 94, 1307–1313 CrossRef CAS.
- F. J. L. Ferreira, L. S. Silva, M. S. da Silva, J. A. Osajima, A. B. Meneguin, S. H. Santagneli, H. S. Barud, R. D. S. Bezerra and E. C. Silva-Filho, Carbohydr. Polym., 2019, 225, 115246 CrossRef PubMed.
- A. Merci, A. Urbano, M. V. E. Grossmann, C. A. Tischer and S. Mali, Food Res. Int., 2015, 73, 38–43 CrossRef CAS.
- M. L. Tummino, R. Nisticò, C. Riedo, D. Fabbri, M. Cerruti and G. Magnacca, Chem. – Eur. J., 2021, 27, 660–668 CrossRef CAS PubMed.
- R. Cai, Y. Zhou, J. Hu, J. Lu, X. Fan, Y. Chen, M. Ding, J. Rong, W. Liu and Y. Chen, J. Water Process Eng., 2022, 50, 103303 CrossRef.
- Y. Li, C. Wang, Y. Luan, W. Liu, T. Chen, P. Liu and Z. Liu, J. Appl. Polym. Sci., 2022, 139, 51647 CrossRef CAS.
- A. Henriksen, O. Mirza, C. Indiani, K. Teilum, G. Smulevich, K. G. Welinder and M. Gajhede, Protein Sci., 2001, 10, 108–115 CrossRef CAS PubMed.
- E. Fernández, D. López, E. López-Cabarcos and C. Mijangos, Polymer, 2005, 46, 2211–2217 CrossRef.
- C. Horn, D. Pospiech, P. J. Allertz, M. Müller, K. Salchert and R. Hommel, ACS Appl. Polym. Mater., 2021, 3, 2823–2834 CrossRef CAS.
-
Hazardous Substances Data Bank (HSDB) - PubChem Data Source, https://pubchem.ncbi.nlm.nih.gov/source/11933, (accessed December 6, 2022) Search PubMed.
- R. P. Ferrari, E. Laurenti and F. Trotta, J. Biol. Inorg. Chem., 1999, 4, 232–237 CrossRef CAS PubMed.
- K. E. Hammel and P. J. Tardone, Biochemistry, 1988, 27, 6563–6568 CrossRef CAS.
- J. Li, J. Peng, Y. Zhang, Y. Ji, H. Shi, L. Mao and S. Gao, J. Hazard. Mater., 2016, 310, 152–160 CrossRef CAS PubMed.
- Q. Huang and W. J. Weber, Environ. Sci. Technol., 2005, 39, 6029–6036 CrossRef CAS PubMed.
- J. Li, Y. Zhang, J. Peng, X. Wu, S. Gao and L. Mao, Chemosphere, 2017, 172, 399–407 CrossRef CAS PubMed.
- Y. Yang, J. Li, H. Shi, L. Zhai, X. Wang and S. Gao, J. Hazard. Mater., 2018, 356, 9–16 CrossRef CAS PubMed.
- H. Catherine, M. Penninckx and D. Frédéric, Environ. Technol. Innovation, 2016, 5, 250–266 CrossRef.
- C. F. Melo and M. Dezotti, J. Chem. Technol. Biotechnol., 2013, 88, 930–936 CrossRef CAS.
- A. Karci, Chemosphere, 2014, 99, 1–18 CrossRef CAS PubMed.
- S. Durpekova, A. Di Martino, M. Dusankova, P. Drohsler and V. Sedlarik, Polymer, 2021, 13, 3274 CAS.
- Ch. J. Mate, S. Mishra and P. K. Srivastava, Polym. Bull., 2021, 78, 3459–3487 CrossRef CAS.
- S. Hokkanen, A. Bhatnagar and M. Sillanpää, Water Res., 2016, 91, 156–173 CrossRef CAS PubMed.
- S. Asadi, S. Eris and S. Azizian, ACS Omega, 2018, 3, 15140–15148 CrossRef CAS PubMed.
- S. A. Qamar, M. Qamar, A. Basharat, M. Bilal, H. Cheng and H. M. N. Iqbal, Chemosphere, 2022, 288, 132618 CrossRef CAS PubMed.
|
This journal is © The Royal Society of Chemistry 2023 |
Click here to see how this site uses Cookies. View our privacy policy here.