DOI:
10.1039/D2SC06497A
(Edge Article)
Chem. Sci., 2023,
14, 2447-2454
Metal-free photosensitized radical relay 1,4-carboimination across two distinct olefins†
Received
24th November 2022
, Accepted 3rd February 2023
First published on 3rd February 2023
Abstract
Intermolecular carboamination of olefins offers a powerful platform for the rapid construction of structurally complex amines from abundant feedstocks. However, these reactions often require transition-metal catalysis, and are mainly limited to 1,2-carboamination. Herein, we report a novel radical relay 1,4-carboimination across two distinct olefins with alkyl carboxylic acid-derived bifunctional oxime esters via energy transfer catalysis. The reaction is highly chemo- and regioselective, and multiple C–C and C–N bonds were formed in a single orchestrated operation. This mild and metal-free method features a remarkably broad substrate scope with excellent tolerance of sensitive functional groups, therefore providing easy access to structurally diverse 1,4-carboiminated products. Moreover, the obtained imines could be easily converted into valuable biologically relevant free γ-amino acids.
Introduction
Nitrogen-containing organic molecules are widely found in natural products, agrochemicals, pharmaceutical agents, and functional materials.1 Consequently, the selective incorporation of amine functionalities into small molecules has received considerable attention in recent years.2 In this context, the carboamination of olefins represents a vibrant research area in organic synthesis, as such transformations allow for the simultaneous installation of C–C and C–N bonds across simple olefins in a single operation, thereby constructing complex molecules in a modular manner from abundant feedstocks.3,4 However, despite significant achievements made over the past decades, some fundamental issues still remain to be solved in this field. First, these reactions often require transition-metal catalysis, rendering them costly and environmentally harmful. Second, the current studies are mainly limited to 1,2-carboamination of olefins, namely introducing carbon and amine functionalities across one olefin at its vicinal positions (Scheme 1A). Undoubtedly, from a synthetic perspective, the development of controllable remote 1,n-carboamination across more than one olefin to modularly access more structurally diverse amines, ideally under mild and metal-free conditions, is highly desirable.
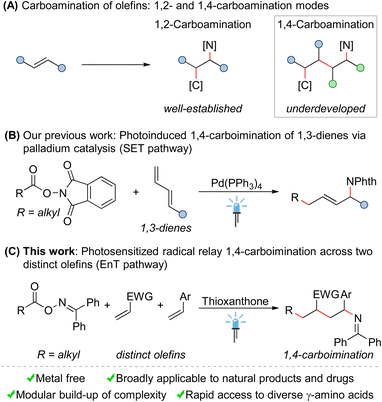 |
| Scheme 1 The development of 1,4-carboamination of olefins. (A) Carboamination of olefins: 1,2- and 1,4-carboamination modes. (B) Our previous work: photoinduced 1,4-carboimination of 1,3-dienes via palladium catalysis (SET pathway). (C) This work: photosensitized radical relay 1,4-carboimination across two distinct olefins (EnT pathway). SET: single electron transfer. EnT: energy transfer. NPhth: phthalimido. | |
Compared to the vigorous growth and abundant achievements in 1,2-carboamination chemistry of olefins, remote carboamination reactions are so far rarely developed. Comparatively few examples are transition-metal (Pd, Rh or Cu)-catalyzed 1,4-carboaminations of 1,3-dienes.5 Moreover, with the development of photochemistry,6 modern visible light-mediated methods for 1,4-carboaminations have recently gradually emerged.7–9 In a notable example, Sarlah and coworkers demonstrated an elegant strategy for the stepwise syn-1,4-carboamination of simple arenes by the combination of photoinduced cycloaddition and palladium-catalyzed ring-opening substitution.7 Very recently, we have realized a palladium-catalyzed 1,4-carboimination of 1,3-dienes with alkylcarboxylic acid-derived bifunctional reagents through a single electron transfer (SET) approach mediated by visible light (Scheme 1B).8 Based on this strategy, the palladium-catalyzed three component 1,4-carboamination of 1,3-dienes with haloalkanes and nitrogen nucleophiles has been subsequently achieved by other groups and us.9 Although these reactions are versatile, they require the use of transition metals (especially palladium), as catalysts. Moreover, the utilization of unstable 1,3-dienes as starting materials further restricts the general appeal of these protocols.
Seeking to address these limitations, we herein disclose an unprecedented radical relay 1,4-carboimination across two distinct olefins with alkyl carboxylic acid-derived bifunctional oxime esters via energy transfer (EnT) catalysis10 (Scheme 1C). This mild and metal-free reaction is highly chemo- and regioselective, and multiple C–C and C–N bonds were formed in a single operation in an orchestrated manner. Moreover, due to the direct utilization of two distinct olefins as starting materials, this method provides modular access to structurally diverse 1,4-carboiminated products, which could be easily converted into valuable biologically relevant free γ-amino acids.11
Results and discussion
Reaction development
Bifunctional reagents have gained a preeminent role within organic synthesis, thanks to their versatility, atom economy, and efficient activation modes.12 Recently, we and others have identified a class of benzophenone-based oxime esters as a suitable bifunctional source for supplying both carbon- and nitrogen-centered radicals through energy transfer catalysis.13–15 Our investigation commenced with the reaction of benzophenone O-pivaloyl oxime (1a), ethene-1,1-diyldibenzene (2a), and acrylonitrile (3a) under visible-light-sensitized conditions (Table 1).
Table 1 Optimization of the reaction conditionsa,b
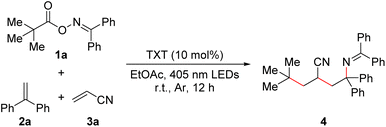
|
Entry |
Variation |
Yieldb [%] |
|
Standard conditions: 1a (0.3 mmol, 1.5 equiv.), 2a (0.2 mmol, 1.0 equiv.), 3a (0.4 mmol, 2.0 equiv.), and thioxanthone (10 mol%) in EtOAc (0.1 M, 2.0 mL), irradiation with 18 W blue LEDs (λmax = 405 nm) under argon atmosphere at room temperature for 12 h.
NMR yields are given.
Isolated yield.
[Ir–F] = [Ir(dF(CF3)ppy)2(dtbbpy)](PF6).
|
1 |
None |
63, 60c |
|
2 |
w/o TXT |
N.R. |
3 |
w/o light |
N.R. |
4 |
450 nm LEDs |
N.R. |
5 |
CH2Cl2 as solvent |
56 |
6 |
Acetone as solvent |
54 |
|
7 |
MeCN as solvent |
57 |
8 |
DCE as solvent |
48 |
9 |
[Ir–F]d (1.0 mol%) instead of TXT and 450 nm LEDs |
50 |
10 |
EtOAc (0.05 M) |
53 |
11 |
1.5 equiv. of 3a |
58 |
12 |
1.0 equiv. of 1a, 2.0 equiv. of 2a and 3a |
53 |
To our delight, after a systematic evaluation of the reaction parameters, the best yield for the desired product 4 reached 63% under the optimized reaction conditions: 1a (0.3 mmol, 1.5 equiv.), 2a (0.2 mmol, 1.0 equiv.), 3a (0.4 mmol, 2.0 equiv.), and thioxanthone (10 mol%) in EtOAc (0.1 M, 2.0 mL), and irradiation with 18 W blue LEDs (λmax = 405 nm) under argon atmosphere at room temperature for 12 h (Table 1, entry 1). Control experiments demonstrated that both thioxanthone and blue light (λmax = 405 nm) were indispensable for this reaction (Table 1, entries 2 and 3). As expected, the reaction did not proceed when switching to a blue light with a wavelength maximum of 450 nm (Table 1, entry 4). This protocol appeared to be relatively robust regarding the choice of solvents, as replacing EtOAc with CH2Cl2, acetone, MeCN, or DCE still provided 4 in similar yields (Table 1, entries 5–8). In addition, [Ir(dF(CF3)ppy)2(dtbbpy)](PF6) proved to be a suitable catalyst for the reaction as well, giving product 4 in 50% yield (Table 1, entry 9). Other variations from the standard conditions, such as decreasing the concentration of the reaction or changing the substrate ratio, led to slightly diminished yields of product 4 (Table 1, entries 10–12). Furthermore, a reaction condition-based sensitivity screening was conducted, showing that the reaction was relatively robust towards moisture, light intensity, scale-up, and small changes in concentration and temperature, but sensitive towards high oxygen level (Table 1, see ESI† for the details).16
Mechanistic analysis
In order to obtain insights into the underlying reaction mechanism, we conducted a series of mechanistic experiments. Only trace amounts of 4 were observed when conducting the standard reaction in the presence of TEMPO ((2,2,6,6-tetramethylpiperidin-1-yl)oxyl), and compound 5 was detected in the reaction mixture by high-resolution mass spectrometry (Fig. 1A). Furthermore, the reaction was carried out under direct excitation conditions (irradiation at 365 nm instead of 405 nm; no photocatalyst), delivering product 4 in 38% NMR yield (see ESI† for the details). These results are consistent with an energy transfer-based radical pathway and an initial radical attack of the generated carbon-centered radical B to 3a. Subsequently, we turned our attention towards the corresponding two-component 1,2-carboiminations of alkenes 2a or 3a with oxime ester 1a (Fig. 1B). Both reactions delivered the corresponding 1,2-carboiminated products (6 and 7) in good yields, even though these compounds were only observed as minor side products in the three-component reaction of 1a, 2a, and 3a (see ESI† for the details). To further probe the origin of the regioselectivity observed for the assembly of 4, we conducted preliminary kinetic studies on both two-component reactions. The initial formation of 7 was found to be more than twice as fast than the formation of 6 (Fig. 1C), which is a hint that the formation of 4 might initiate with fast radical attack to 3a. Lastly, the quantum yield of the reaction of 1a, 2a, and 3a was determined. A value of 0.40 was obtained, rendering a pure radical chain mechanism unlikely (Fig. 1D).15c However, a mixed radical chain/radical recombination mechanism cannot be ruled out.
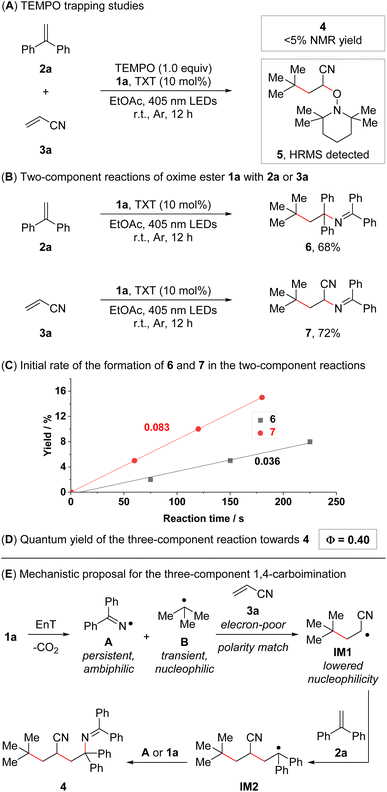 |
| Fig. 1 Mechanistic studies and mechanistic proposal. (A) TEMPO trapping experiment. Reaction conditions: 1a (0.15 mmol, 1.5 equiv.), 2a (0.1 mmol, 1.0 equiv.), 3a (0.2 mmol, 2.0 equiv.), thioxanthone (TXT, 10 mol%), and TEMPO (0.1 mmol, 1.0 equiv.) in EtOAc (0.1 M, 1.0 mL), 18 W blue LEDs (λmax = 405 nm), r.t., 12 h. (B) Two-component reactions of 1a with 2a or 3a. Reaction conditions: 1a (0.3 mmol, 1.5 equiv.), 2a or 3a (0.2 mmol, 1.0 equiv.), and thioxanthone (10 mol%) in EtOAc (0.1 M, 2.0 mL), 18 W blue LEDs (λmax = 405 nm), r.t., 12 h. Isolated yields given. (C) Initial course and rate of the reaction between 1a and 2a and the reaction between 1a and 3a (see ESI† for the details). (D) Quantum yield of the reaction between 1a, 2a, and 3a (see ESI† for the details). (E) Mechanistic proposal for the intermolecular 1,4-carboimination of 2a and 3a with 1a. | |
Based on these experiments and our previous studies,13,14 a mechanistic analysis of the selective 1,4-carboimination of 1a, 2a, and 3a was developed (Fig. 1E, see ESI† for details). The reaction initiates with an energy transfer-enabled homolysis of 1a to provide the persistent N-centered iminyl radical A and the transient C-centered alkyl radical B, which are of ambiphilic and nucleophilic properties, respectively. Then, steered by polarity matching, persistent radical effects (PRE),17 and a higher concentration of 3a compared to 2a, the nucleophilic C-centered alkyl radical B preferentially adds to electron poor olefin 3a to form an α-cyano-containing C-centered alkyl radical with reduced nucleophilicity (IM1). Subsequently, addition of IM1 to 2a gives another benzyl stabilized C-centered alkyl radical (IM2), which is further trapped by the persistent N-centered iminyl radical A to furnish the desired 1,4-carboimination product 4via radical recombination. Alternatively, due to low concentrations of the radical species and a relatively high concentration of oxime ester 1a, IM2 can form product 4via radical attack to oxime ester 1a and subsequent fragmentation, representing a radical chain mechanism.15c,18
This mechanism is therefore related to our previous work, in which we used oxime carbonates as a source for electrophilic oxygen-centered radicals to achieve a three-component 1,4-oxyimination.18 In both cases, the desired regioselectivity was achieved by rational reaction design based on the philicity of the generated radicals and the polarity of the olefins. Notably, the different philicities of carbon- and oxygen-centered radicals cause a different order of building block assembly depending on the selected reagent.
Synthetic scope
With the optimized reaction conditions in hand, we conducted an additive-based robustness screening to initially assess the reaction's functional group compatibility. The obtained results highlighted the outstanding tolerance of heterocycles and functional groups and already hinted towards the broadness of the substrate scope (see ESI† for the details).19 Next, the substrate scope with respect to the three reaction partners was examined (Table 2). First, a range of alkyl carboxylic acid-derived bifunctional oxime esters was explored. Tertiary alkyl carboxylic acids smoothly underwent this radical relay 1,4-carboimination reaction, providing the corresponding products in moderate to good yields (Table 2A, 4 and 8–12). Notably, these reactions were highly chemo- and regioselective, and two quaternary carbon centers were easily created, demonstrating the appeal of our method in building complex molecules. Then, a series of secondary and primary carboxylic acids was tested (Table 2B, 13–24). Various cyclic and acyclic carboxylic acids were all well compatible with this protocol, delivering the desired products in moderate to good yields. The structure of product 19 was further confirmed by X-ray crystallography (Table 2B).20 Subsequently, we turned our attention to the compatibility of olefins. As shown in Table 2C, diverse styrene derivatives with different electron-donating or electron-withdrawing substituents in various positions of the aromatic ring were found to be effective substrates for this 1,4-carboimination reaction, producing the corresponding products in moderate to good yields (Table 2C, 25–36). Functional groups such as bromide, acetoxy and an ester containing a tosyl group were well tolerated, offering opportunities for further functionalization. Moreover, a series of Michael acceptors such as acrylates and vinyl phosphate were also suitable for this transformation (Table 2D, 37–46). Satisfactorily, a variety of sensitive functional groups like alkenyl fluoride, trifluoromethyl, alkynyl, ester, phosphonate, and even a free alcohol were well tolerated in this method. Due to polar mismatch, electron rich and unactivated olefins were not suitable for this protocol. We further tested oxime esters with different imine moieties leading to products 47–50. Benzophenone-derived oxime esters containing fluoride, bromide, or methoxy groups were tolerated. Notably, an acetophenone-derived oxime ester delivered product 50 in moderate yield with well-controlled E/Z-selectivity.
Table 2 Scope of alkyl carboxylic acid-derived bifunctional oxime esters and olefinsa,b
Reaction conditions: Oxime esters 1 (0.3 mmol, 1.5 equiv.), styrene derivatives 2 (0.2 mmol, 1.0 equiv.), Michael acceptors 3 (0.4 mmol, 2.0 equiv.), and thioxanthone (10 mol%) in EtOAc (0.1 M, 2.0 mL), irradiation with 18 W blue LEDs (λmax = 405 nm) under argon atmosphere at room temperature for 12 h.
Isolated yields are given. The d.r. and E/Z values were determined by 1H NMR analysis.
|
|
Among various potential carbon-centered radical precursors, alkyl carboxylic acids represent an ideal starting material as these stable compounds are abundant, cheap, and are found in numerous natural products and pharmaceuticals.21 To demonstrate the broad applicability of the protocol, its capability to couple structurally complex natural products and pharmaceuticals with olefins 2a and 3a was investigated. As summarized in Table 3, many amino acids and peptides such as Z-Gly-OH (51), Z-D-Asp-OMe (52), Boc-Gly-Gly-OH (53), and Z-Gly-Gly-Gly-OH (54) were examined, giving the corresponding products in good yields. Moreover, a series of naturally occurring alkyl carboxylic acids including stearic acid (55), oleic acid (56), erucic acid (57), and linoleic acid (58) smoothly reacted with olefins 2a and 3a to afford good yields of the 1,4-carboimination products. Notably, the contained C–C double bonds remained untouched. (1S)-(−)-Camphanic acid (59) and diprogulic acid (63) were also smoothly engaged in the 1,4-carboimination reaction. Pharmaceuticals like sulbactam (60), gemfibrozil (61), and ciprofibrate (62) were successfully utilized as well, generating the desired imines in good yields. Finally, various complex steroid molecules, such as oleanolic acid (64), dehydroabietic acid (65), glycyrrhetinic acid (66), dehydrocholic acid (67), and lithocholic acid (68) were also suitable for this transformation. Overall, these examples clearly highlight the broadness of the developed reaction and its compatibility with complex molecules.
Table 3 Modification of natural products and drugsa,b
Reaction conditions: oxime esters 1 (0.3 mmol, 1.5 equiv.), ethene-1,1-diyldibenzene 2a (0.2 mmol, 1.0 equiv.), acrylonitrile 3a (0.4 mmol, 2.0 equiv.), and thioxanthone (10 mol%) in EtOAc (0.1 M, 2.0 mL), irradiation with 18 W blue LEDs (λmax = 405 nm) under argon atmosphere at room temperature for 12 h.
Isolated yields are given. The d.r. values were determined by 1H NMR analysis.
Oxime ester 1ad (0.2 mmol, 1.0 equiv.), ethene-1,1-diyldibenzene 2a (0.4 mmol, 2.0 equiv.), acrylonitrile 3a (0.4 mmol, 2.0 equiv.) were used.
|
|
To further demonstrate the synthetic practicality of this protocol, a gram scale reaction of 1y, 2a, and 3a was performed, affording the desired product 57 in 42% yield (1.48 g). Dihydroxylation of product 57 was conducted,8 furnishing product 69 in 78% yield (Scheme 2A). Treating selected 1,4-carboimination products (21 or 68) with 6 N HCl at 100 °C for 12 h provided the corresponding biologically relevant free γ-amino acids (70 and 71), further highlighting the utility of this method (Scheme 2B).
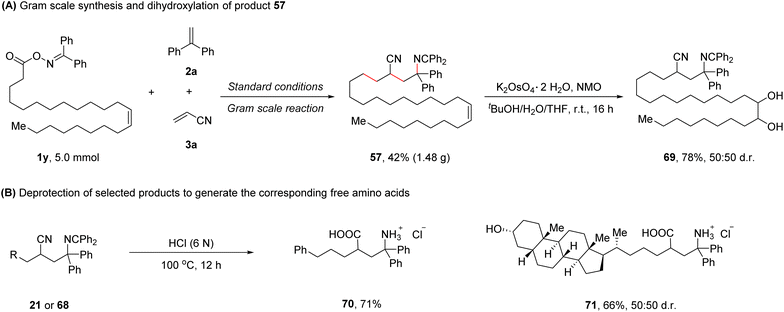 |
| Scheme 2 Synthetic applications. (A) Gram scale synthesis and dihydroxylation of product 57. (B) Deprotection of selected products to generate the corresponding free amino acids. NMO: N-methylmorpholine N-oxide. | |
Conclusions
In summary, we have developed a novel radical relay 1,4-carboimination across two distinct olefins with alkyl carboxylic acid-derived bifunctional oxime esters. Metal-free energy transfer catalysis enables the well-choreographed, highly chemo- and regioselective formation of three bonds in one single operation. The presented protocol is characterized by a remarkably broad substrate scope with excellent tolerance of sensitive functional groups, therefore allowing for the modular construction of a large variety of highly complex imines which could be easily converted into valuable biologically relevant free γ-amino acids. We believe that this work will be of interest to the synthetic community, and will inspire researchers to further explore other remote 1,n-difunctionalization reactions.
Data availability
The authors declare that the data supporting the findings of this study are available within the paper and the ESI,† as well as from the authors upon request.
Author contributions
G. T., F. P. and F. G. conceived the project; G. T., F. P. and A. P. performed the experiments and analyzed the data; M.-A. W. and A. L. prepared some of the starting materials; C. D. characterized the X-ray structure of 19; G. T., F. P. and F. G. wrote the manuscript with contributions from all authors; F. G. supervised the project.
Conflicts of interest
There are no conflicts to declare.
Acknowledgements
We thank the Alexander von Humboldt Foundation (G. T.; A. P.) and the Deutsche Forschungsgemeinschaft (Leibniz Award, F. G.; GRK 2678-437785492, F. P.) for supporting this work.
Notes and references
-
A. Ricci, Amino Group Chemistry: From Synthesis to the Life Sciences, Wiley-VCH, Weinheim, 2008 Search PubMed.
- For selected reviews, see:
(a) J. P. Wolfe, S. Wagaw, J.-F. Marcoux and S. L. Buchwald, Acc. Chem. Res., 1998, 31, 805 CrossRef CAS;
(b) J. F. Hartwig, Nature, 2008, 455, 314 CrossRef CAS PubMed;
(c) T. E. Müller, K. C. Hultzsch, M. Yus, F. Foubelo and M. Tada, Chem. Rev., 2008, 108, 3795 CrossRef PubMed;
(d) M.-L. Louillat and F. W. Patureau, Chem. Soc. Rev., 2014, 43, 901 RSC;
(e) J. Jiao, K. Murakami and K. Itami, ACS Catal., 2016, 6, 610 CrossRef CAS;
(f) Y. Park, Y. Kim and S. Chang, Chem. Rev., 2017, 117, 9247 CrossRef CAS PubMed.
- For selected recent reviews on carboamination of olefins, see:
(a) H. Jiang and A. Studer, Chem. Soc. Rev., 2020, 49, 1790 RSC;
(b) X. Chen, F. Xiao and W.-M. He, Org. Chem. Front., 2021, 8, 5206 RSC;
(c) K. Hirano and M. Miura, J. Am. Chem. Soc., 2022, 144, 648 CrossRef CAS PubMed.
- For selected examples on intermolecular 1,2-carboamination of olefins, see:
(a) D. N. Mai and J. P. Wolfe, J. Am. Chem. Soc., 2010, 132, 12157 CrossRef CAS PubMed;
(b) K. Weidner, A. Giroult, P. Panchaud and P. Renaud, J. Am. Chem. Soc., 2010, 132, 17511 CrossRef CAS PubMed;
(c) H. Zhang, W. Pu, T. Xiong, Y. Li, X. Zhou, K. Sun, Q. Liu and Q. Zhang, Angew. Chem., Int. Ed., 2013, 52, 2529 CrossRef CAS PubMed;
(d) D. Prasad Hari, T. Hering and B. König, Angew. Chem., Int. Ed., 2014, 53, 725 CrossRef CAS PubMed;
(e) A. Faulkner, J. S. Scott and J. F. Bower, J. Am. Chem. Soc., 2015, 137, 7224 CrossRef CAS PubMed;
(f) J. Cheng, X. Qi, M. Li, P. Chen and G. Liu, J. Am. Chem. Soc., 2015, 137, 2480 CrossRef CAS PubMed;
(g) T. Piou and T. Rovis, Nature, 2015, 527, 86 CrossRef CAS PubMed;
(h) S. Kawamura, H. Egami and M. Sodeoka, J. Am. Chem. Soc., 2015, 137, 4865 CrossRef CAS PubMed;
(i) J.-S. Lin, X.-Y. Dong, T.-T. Li, N.-C. Jiang, B. Tan and X.-Y. Liu, J. Am. Chem. Soc., 2016, 138, 9357 CrossRef CAS PubMed;
(j) A. Lerchen, T. Knecht, C. G. Daniliuc and F. Glorius, Angew. Chem., Int. Ed., 2016, 55, 15166 CrossRef CAS PubMed;
(k) Y.-Y. Liu, X.-H. Yang, R.-J. Song, S. Luo and J.-H. Li, Nat. Commun., 2017, 8, 14720 CrossRef PubMed;
(l) Z. Liu, Y. Wang, Z. Wang, T. Zeng, P. Liu and K. M. Engle, J. Am. Chem. Soc., 2017, 139, 11261 CrossRef CAS PubMed;
(m) B. Qian, S. Chen, T. Wang, X. Zhang and H. Bao, J. Am. Chem. Soc., 2017, 139, 13076 CrossRef CAS PubMed;
(n) J. Davies, N. S. Sheikh and D. Leonori, Angew. Chem., Int. Ed., 2017, 56, 13361 CrossRef CAS PubMed;
(o) A. Bunescu, T. M. Ha, Q. Wang and J. Zhu, Angew. Chem., Int. Ed., 2017, 56, 10555 CrossRef CAS PubMed;
(p) Y. Zhang, H. Liu, L. Tang, H.-J. Tang, L. Wang, C. Zhu and C. Feng, J. Am. Chem. Soc., 2018, 140, 10695 CrossRef CAS PubMed;
(q) T. M. Monos, R. C. McAtee and C. R. J. Stephenson, Science, 2018, 361, 1369 CrossRef CAS PubMed;
(r) X. Bao, T. Yokoe, T. M. Ha, Q. Wang and J. Zhu, Nat. Commun., 2018, 9, 3725 CrossRef PubMed;
(s) S. N. Gockel, T. L. Buchanan and K. L. Hull, J. Am. Chem. Soc., 2018, 140, 58 CrossRef CAS PubMed;
(t) H. Xiao, H. Shen, L. Zhu and C. Li, J. Am. Chem. Soc., 2019, 141, 11440 CrossRef CAS PubMed;
(u) Y. Moon, B. Park, I. Kim, G. Kang, S. Shin, D. Kang, M.-H. Baik and S. Hong, Nat. Commun., 2019, 10, 4117 CrossRef PubMed;
(v) H. Jiang, G. Seidler and A. Studer, Angew. Chem., Int. Ed., 2019, 58, 16528 CrossRef CAS PubMed;
(w) H. Jiang, X. Yu, C. G. Daniliuc and A. Studer, Angew. Chem., Int. Ed., 2021, 60, 14399 CrossRef CAS PubMed.
- For transition metals-catalyzed 1,4-carboamination of 1,3-dienes, see:
(a) B. A. Patel, J. E. Dickerson and R. F. Heck, J. Org. Chem., 1978, 43, 5018 CrossRef CAS;
(b) B. A. Patel, L.-C. Kao, N. A. Cortese, J. V. Minkiewicz and R. F. Heck, J. Org. Chem., 1979, 44, 918 CrossRef CAS;
(c) J. M. O'Connor, B. J. Stallman, W. G. Clark, A. Y. L. Shu, R. E. Spada, T. M. Stevenson and H. A. Dieck, J. Org. Chem., 1983, 48, 807 CrossRef;
(d) H. Bao, L. Bayeh and U. K. Tambar, Chem. Sci., 2014, 5, 4863 RSC;
(e) T. Pinkert, T. Wegner, S. Mondal and F. Glorius, Angew. Chem., Int. Ed., 2019, 58, 15041 CrossRef CAS PubMed.
- For selected reviews and book on photochemistry, see:
(a) J. M. R. Narayanam and C. R. J. Stephenson, Chem. Soc. Rev., 2011, 40, 102 RSC;
(b) J. Xuan and W.-J. Xiao, Angew. Chem., Int. Ed., 2012, 51, 6828 CrossRef CAS PubMed;
(c) C. K. Prier, D. A. Rankic and D. W. C. MacMillan, Chem. Rev., 2013, 113, 5322 CrossRef CAS PubMed;
(d) K. L. Skubi, T. R. Blum and T. P. Yoon, Chem. Rev., 2016, 116, 10035 CrossRef CAS PubMed;
(e) M. Silvi and P. Melchiorre, Nature, 2018, 554, 41 CrossRef CAS PubMed;
(f)
C. R. J. Stephenson, T. Yoon and D. W. C. MacMillan, Visible Light Photocatalysis in Organic Chemistry, Wiley-VCH, Weinheim, 2018 CrossRef.
- For stepwise dearomative 1,4-carboaminations, see:
(a) M. Okumura, A. S. Shved and D. Sarlah, J. Am. Chem. Soc., 2017, 139, 17787 CrossRef CAS PubMed;
(b) C. Tang, M. Okumura, Y. Zhu, A. R. Hooper, Y.-H. Lee and D. Sarlah, Angew. Chem., Int. Ed., 2019, 58, 10245 CrossRef CAS PubMed.
- H.-M. Huang, M. Koy, E. Serrano, P. M. Pflüger, J. L. Schwarz and F. Glorius, Nat. Catal., 2020, 3, 393 CrossRef CAS.
-
(a) H.-M. Huang, P. Bellotti, P. M. Pflüger, J. L. Schwarz, B. Heidrich and F. Glorius, J. Am. Chem. Soc., 2020, 142, 10173 CrossRef CAS PubMed;
(b) P. Bellotti, M. Koy, C. Gutheil, S. Heuvel and F. Glorius, Chem. Sci., 2021, 12, 1810 RSC;
(c) H.-M. Huang, P. Bellotti, S. Kim, X. Zhang and F. Glorius, Nat. Synth., 2022, 1, 464 CrossRef;
(d) Z.-L. Liu, Z.-P. Ye, Y.-X. Chen, Y. Zheng, Z.-Z. Xie, J.-P. Guan, J.-A. Xiao, K. Chen, H.-Y. Xiang and H. Yang, Org. Lett., 2022, 24, 924 CrossRef CAS PubMed;
(e) C. Fu, Z. Zhang, Y. Li, D. Gao, Z.-N. Cui and Z. Li, Chem. Commun., 2022, 58, 5614 RSC.
- For selected reviews on energy transfer catalysis, see:
(a) F. Strieth-Kalthoff, M. J. James, M. Teders, L. Pitzer and F. Glorius, Chem. Soc. Rev., 2018, 47, 7190 RSC;
(b) Q.-Q. Zhou, Y.-Q. Zou, L.-Q. Lu and W.-J. Xiao, Angew. Chem., Int. Ed., 2019, 58, 1586 CrossRef CAS PubMed;
(c) F. Strieth-Kalthoff and F. Glorius, Chem, 2020, 6, 1888 CrossRef CAS.
-
P. Spiteller, Amino acids, peptides and proteins in organic chemistry, Wiley-VCH, Weinheim, 2009 Search PubMed.
- H.-M. Huang, P. Bellotti, J. Ma, T. Dalton and F. Glorius, Nat. Rev. Chem., 2021, 5, 301 CrossRef CAS.
- T. Patra, P. Bellotti, F. Strieth-Kalthoff and F. Glorius, Angew. Chem., Int. Ed., 2020, 59, 3172 CrossRef CAS PubMed.
- G. Tan, M. Das, H. Keum, P. Bellotti, C. Daniliuc and F. Glorius, Nat. Chem., 2022, 14, 1174 CrossRef CAS PubMed.
-
(a) P.-J. Xia, F. Liu, Y.-M. Pan, M.-P. Yang and Y.-Y. Yang, Org. Chem. Front., 2022, 9, 2522 RSC;
(b) X. Wang, Y. Chen, P. Liang, J.-Q. Chen and J. Wu, Org. Chem. Front., 2022, 9, 4328 RSC;
(c) J. Majhi, R. K. Dhungana, Á. Rentería-Gómez, M. Sharique, L. Li, W. Dong, O. Gutierrez and G. A. Molander, J. Am. Chem. Soc., 2022, 144, 15871 CrossRef CAS PubMed.
- L. Pitzer, F. Schäfers and F. Glorius, Angew. Chem., Int. Ed., 2019, 58, 8572 CrossRef CAS PubMed.
- For reviews on PRE effect, see:
(a) H. Fische, Chem. Rev., 2001, 101, 3581 CrossRef PubMed;
(b) D. Leifert and A. Studer, Angew. Chem., Int. Ed., 2020, 59, 74 CrossRef CAS PubMed.
- G. Tan, F. Paulus, Á. Rentería-Gómez, R. F. Lalisse, C. G. Daniliuc, O. Gutierrez and F. Glorius, J. Am. Chem. Soc., 2022, 144, 21664 CrossRef CAS PubMed.
- T. Gensch, M. Teders and F. Glorius, J. Org. Chem., 2017, 82, 9154 CrossRef CAS PubMed.
- CCDC 2207448 (19)†.
-
(a) N. Rodríguez and L. J. Goossen, Chem. Soc. Rev., 2011, 40, 5030 RSC;
(b) C. P. Johnston, R. T. Smith, S. Allmendinger and D. W. C. MacMillan, Nature, 2016, 536, 322 CrossRef CAS PubMed;
(c) T. Qin, J. Cornella, C. Li, L. R. Malins, J. T. Edwards, S. Kawamura, B. D. Maxwell, M. D. Eastgate and P. S. Baran, Science, 2016, 352, 801 CrossRef CAS PubMed.
Footnotes |
† Electronic supplementary information (ESI) available. CCDC 2207448. For ESI and crystallographic data in CIF or other electronic format see DOI: https://doi.org/10.1039/d2sc06497a |
‡ Equal contribution. |
|
This journal is © The Royal Society of Chemistry 2023 |
Click here to see how this site uses Cookies. View our privacy policy here.