DOI:
10.1039/D3SC03083K
(Edge Article)
Chem. Sci., 2023,
14, 9885-9891
Synthesis of polysubstituted bicyclo[2.1.1]hexanes enabling access to new chemical space†
Received
16th June 2023
, Accepted 30th August 2023
First published on 30th August 2023
Abstract
Saturated bridged-bicyclic compounds are currently under intense investigation as building blocks for pharmaceutical drug design. However, the most common methods for their preparation only provide access to bridgehead-substituted structures. The synthesis of bridge-functionalised species is highly challenging but would open up many new opportunities for molecular design. We describe a photocatalytic cycloaddition reaction that provides unified access to bicyclo[2.1.1]hexanes with 11 distinct substitution patterns. Bridge-substituted structures that represent ortho-, meta-, and polysubstituted benzene bioisosteres, as well as those that enable the investigation of chemical space inaccessible to aromatic motifs can all be prepared using this operationally simple protocol. Proof-of-concept examples of the application of the method to the synthesis of saturated analogues of biorelevant trisubstituted benzenes are also presented.
Introduction
The inclusion of more saturated and three-dimensional structures in drug-discovery programmes has been identified as a key goal of future pharmaceutical research.1,2 In this context, the use of bridgehead-disubstituted bridged bicyclic compounds has seen enormous growth in recent years (Scheme 1A). Building on early work on the preparation of para-disubstituted cubanes,3,4 bridgehead disubstituted bicyclo[1.1.1]pentanes are now frequently employed as bioisosteres of para-substituted benzenes5,6 and more recently, bridgehead disubstituted bicyclo[3.1.1]heptanes7 and bicyclo[2.1.1]hexanes8 have been prepared as bioisosteres of meta-substituted benzene.9,10 However, in these examples, the bridge positions of the polycyclic scaffold remain untouched and therefore unutilised, despite the opportunities that their functionalisation would provide for drug design (Scheme 1B). For example, substitution of the bridge positions of bicyclo[2.1.1]hexanes (BCHs) could be used to extend the bioisostere concept to benzenes containing three or more substituents (328 U.S. Food and Drug Administration approved drugs contain a 1,2,4-trisubstituted benzene)11,12 and the additional exit vectors from the bridge positions provide opportunities to explore chemical space that is inaccessible to aromatic motifs. As just one example, the apical substituent of a polysubstituted BCH would occupy the space of a hypothetical substituent above the π-system of a benzene ring.
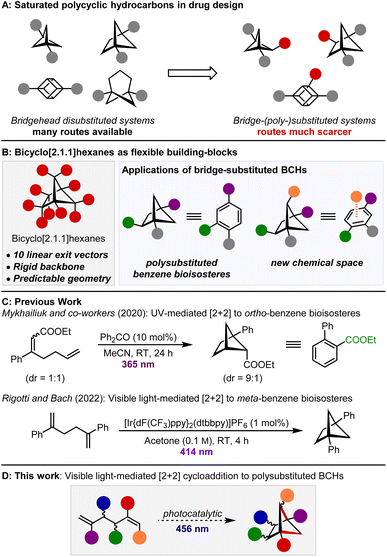 |
| Scheme 1 Bioisosteres of benzene and intramolecular crossed [2 + 2] cycloaddition as a strategy to access polysubstituted bicyclo[2.1.1]hexanes. | |
Exploration of these opportunities is currently hampered by the scarcity of methods that enable the preparation of the required compounds. Selective functionalisation of the bridge positions via C–H functionalisation or cross-coupling approaches is challenging, with only a handful of examples for bicyclo[1.1.1]pentanes13,14 and the related cubanes reported.15 For example, MacMillan and co-workers reported a two-step bridge functionalisation of bicyclo[1.1.1]pentanes via bromination and subsequent metallaphotoredox amination or arylation.13a Examples of the functionalisation of BCHs are particularly rare.16De novo approaches which install additional substituents directly and offer a complementary approach to this challenge are emerging, but remain uncommon.17 The recent reports of fragment insertion into bicyclo[1.1.0]butanes are powerful examples of the concept.18,19 Cleavage of the central C–C bond enables insertion of alkenes,18a–e,g,i,k ketones18h or even ring-opened cyclopropanes18j,f and leads to a range of bridge-substituted structures. Despite these important advances, the above methods typically only open up one or two new substitution patterns. The ideal strategy would offer greater flexibility and provide predictable access to multiple different substitution patterns using a common disconnection. This would streamline the synthesis of these compounds and be extremely powerful for evaluating their use in drug discovery.
We recently published an organophotocatalytic [2 + 2] cycloaddition of 1,6-heptadienes to fused bicyclo[3.2.0]heptanes.20 The 1,5-hexadiene homologues are known to instead undergo crossed [2 + 2] cycloaddition to give the bridged BCHs, either by direct irradiation of the substrate21 or triplet sensitization with ultraviolet (UV) light.22 Recently, Mykhailiuk and co-workers reported a UV light/benzophenone-mediated protocol to bridge-substituted 1,5-disubstituted BCHs that could function as ortho-substituted benzene bioisosteres (Scheme 1C).17b,23,24 However, this reactivity platform is extremely versatile and we believed it could be used to give targeted access to the 10 different exit vectors of BCHs (Scheme 1D). This in turn would enable preparation of a whole range of differently-substituted BCHs, containing various combinations of bridge and bridgehead substituents, consistent with the reaction design criteria outlined above. In addition, we wanted to develop a visible light-mediated procedure that would eliminate the need to use UV light sources and tolerate a broad range of functional groups.
During the course of our investigation, Fessard, Salomé and co-workers16c and Rigotti and Bach (Scheme 1C)8a reported related [2 + 2] cycloadditions of 1,5-hexadienes. While these methods are limited to 1,2- and 1,4-disubstituted BCHs respectively, we are able to prepare BCHs with in total 11 distinct substitution patterns, and provide examples of the use of the method to the preparation of saturated analogues of biorelevant trisubstituted benzenes.
Results and discussion
We began with model diene 1a, and reaction optimization revealed that [Ir{dF(CF3)ppy}2(dtbbpy)]PF6 could catalyse the desired [2 + 2] cycloaddition with irradiation at 456 nm (for further details of the optimisation, see ESI†). Under these conditions, diene 1a was transformed into BCH 2a in near quantitative yield (Table 1, entry 1). For comparison with other commonly used photocatalysts, reaction with [Ru(bpy)3]Cl2 afforded none of desired BCH 2a (entry 2), but the organic dye 4CzIPN allowed for moderate conversion over an extended reaction time (entry 3). Reducing the catalyst loading led to a lower conversion (entry 4), and control experiments in the absence of photocatalyst (entry 5) or irradiation (entry 6) led to no formation of BCH 2a. Performing the reaction on larger scales was also successful, with BCH 2a being isolated in 94% yield on 0.19 mmol scale (entry 7) and 91% yield on 1.00 mmol scale (entry 8).
Table 1 Optimization of reaction conditions

|
Entrya |
Deviation from standard conditions |
Yieldb (%) |
The reactions were performed on 10 mg (of 1a) scale and were degassed by three freeze–pump–thaw cycles prior to irradiation. For further details see ESI.
Yield estimated from the 1H NMR spectrum of the reaction mixture relative to 1,3,5-trimethoxybenzene as internal standard.
Conversion estimated by 1H NMR spectroscopy relative to unreacted 1a.
Isolated yield. [Ir] = [Ir{dF(CF3)ppy}2(dtbbpy)]PF6.
|
1 |
None |
96 |
2 |
[Ru(bpy)3]Cl2 |
<5 |
3 |
4CzIPN, 48 h |
55 |
4 |
1 mol% [Ir] |
27c |
5 |
No photocatalyst |
<5 |
6 |
Reaction in the dark |
<5 |
7 |
44.5 mg, 0.19 mmol |
94d |
8 |
234 mg, 1.00 mmol, 66 h |
91d |
With optimized conditions in hand, we turned our attention to the substrate scope of the reaction (Scheme 2). A range of disubstituted dienes 1b–1ab were prepared (see ESI for details†) and subjected to the optimized reaction conditions. All contained at least one styrene-type motif, which is required for photoexcitation. To make comparison between differently substituted BCHs simpler, the numbered positions of the substituents are given alongside the formed structure.
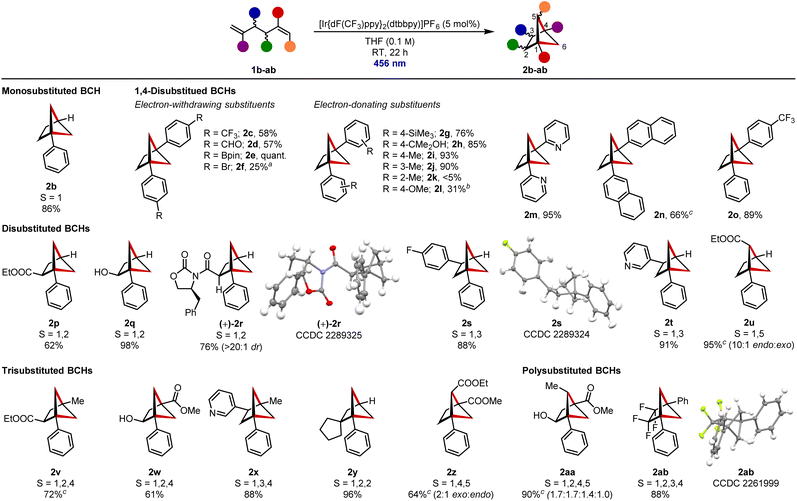 |
| Scheme 2 Scope of the photocatalytic crossed [2 + 2] cycloaddition. Reactions were performed on 0.20 mmol scale. Yields refer to isolated material after flash column chromatography. S = substituent position. a Yield estimated from the 1H NMR spectrum of the reaction mixture relative to 1,3,5-trimethoxybenzene as internal standard. b Isolated together with unreacted diene 1l. c 66 h reaction time. Displacement ellipsoids are drawn at 50% probability level. | |
To begin with, monosubstituted BCH 2b, bearing a single bridgehead phenyl group, was formed in 86% yield.22b A selection of disubstituted BCHs were then prepared to enable assessment of the functional group tolerance of the reaction. Electron-withdrawing substituents including a trifluoromethyl group (in 2c), aldehyde (in 2d) and boronate ester (in 2e) were well tolerated. Boronate ester 2e was a standout, being prepared in quantitative yield. Bromide substituents could also be incorporated but resulted in lower conversion to the target BCH 2f.
Electron-donating substituents such as a trimethylsilyl group (in 2g) and an unprotected tertiary alcohol (in 2h) were also tolerated. A series of tolyl derivatized dienes 1i–k was prepared to establish the effect of increased steric hindrance on the reaction; both para-tolyl- (in 2i) and meta-tolyl-substituted BCHs (in 2j) could be prepared, but the ortho-tolyl substituents of diene 1k prevented the desired cycloaddition. Methoxy substituents (in 2l) led to lower conversions. Heteroaromatic rings such as pyridines (in 2m) as well as extended aromatic systems (in 2n) could, however, be successfully used in place of phenyl substituents to facilitate photochemical activation. Rigotti and Bach have previously prepared a wide range of 1,4-disubstituted BCHs bearing two different substituents.8a We additionally prepared heterodiaryl-1,4-disubstituted BCH 2o in 89% yield.
Our focus then shifted to the preparation of disubstituted BCHs that could be used as ortho- or meta-benzene bioisosteres.9c 1,2-Disubstituted BCH 2p, containing a useful ester function group handle at the bridge 2-position, could be accessed in 62% yield. An alcohol functional group could also be installed in this position, with BCH 2q then being isolated in 98% yield. Importantly, we were able to demonstrate that stereochemical information in the carbon backbone was retained through the cycloaddition. BCH (+)-2r was prepared in 76% yield and as a single diastereomer from the corresponding diene (also single diastereomer) and no evidence of epimerisation of the α-carbonyl stereocentre could be identified by 1H NMR spectroscopy. The absolute (S,S) stereochemistry was additionally confirmed by X-ray analysis. 1,3-Disubstituted BCHs 2s and 2t, bearing a fluorinated aryl ring and a pyridine respectively at the bridge 3-position, were formed in 88% and 91% yield. 1,5-Disubstituted BCH 2u, with an ester on a single-carbon bridge, was formed in high selectively (10
:
1) as the endo diastereomer (endo diastereomer has the ester substituent pointing into the larger, 5-membered ring) and was isolated in an excellent 95% yield.17b
Moving onto trisubstituted BCH frameworks, 1,2,4-trisubstituted BCH 2v, which contains both aromatic and aliphatic bridgehead substituents as well as an ester at the bridge 2-position, was formed in 72% yield. 1,2,4-Trisubstituted BCH 2w, bearing an unprotected alcohol at the bridge 2-position, was prepared in 61% yield and 1,3,4-trisubstituted BCH 2x with a pyridine at the bridge 3-position was isolated in 88% yield. These structures could be considered as potential bioisosteres of the corresponding trisubstituted benzenes. We were also able to prepare 1,2,2-substituted BCH 2y in 96% yield, which contains a spirocyclic centre at the bridge 2-position. 1,4,5-Trisubstituted BCH 2z, bearing a methyl ester at the bridgehead position and ethyl ester on the single-carbon bridge, was prepared in 64% yield and 2
:
1 (exo
:
endo) selectivity.25 Here, the bridge ethyl ester adopts an ortho-like position, but is laterally offset relative to the plane of the meta-like bridgehead substituents, a spatial disposition inaccessible in aromatic chemical space. Finally, we wanted to demonstrate that the method could be used to prepare BCHs containing more than three substituents. 1,2,4,5-Tetrasubstituted BCH 2aa, which contains four different substituents at four different positions including an unprotected alcohol at the bridge 2-position, an ester, and an apical alkyl group at the bridge 5-position, was prepared in 90% yield as a mixture of diastereomers. This substituent pattern occupies chemical space not accessible to planar aromatic systems, and highlights the opportunities for molecular design that are available with these types of structures. Fluorinated bicyclo[1.1.1]pentanes are highly desirable building blocks in medicinal chemistry and we were able to access related 2,2,3,3-bridge-tetrafluorinated BCH 2ab in 88% yield.26
We were also able to obtain crystal structures of a number of the BCH derivatives and perform a comparative exit vector analysis (Scheme 3). For the meta-isosteres, comparison was made to m-terphenyl27 and for ortho-isostere (+)-2r, comparison was made to Telmisartan, which bears a similar ortho-carbonyl substitution pattern.28 For the meta-isosteres, 1,4-disubstituted BCHs 2a and 2ab, and 1,3-disubstituted BCH 2s could be analysed. All showed similar inter-substituent distances compared to m-terphenyl, but the substituent angles are arguably a better match with the 1,4-disubstituted derivatives, particularly with respect to the substituent torsion angle (near 0° for 2a and 2ab but 78.2° for 2s). For ortho-isostere (+)-2r, the inter-substituent distance was an extremely good match for Telmisartan, although the angle between them in (+)-2r was smaller than for the parent aromatic. As expected, a torsion angle of 58° between the two substituents could be measured.
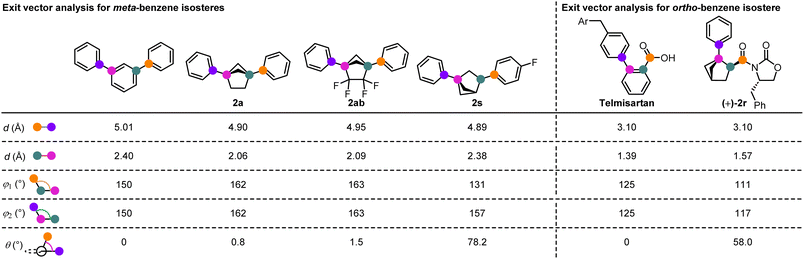 |
| Scheme 3 Exit vector analysis for selected ortho- and meta-benzene isosteres in comparison to related aromatic systems. | |
We were then able to demonstrate the viability of this method to access saturated analogues of biorelevant trisubstituted benzenes (Scheme 4A). We identified amide 5 and fendizoic acid (7) as model trisubstituted benzenes and BCHs 4 and 6 as simplified saturated analogues that reflected the substitution pattern of the central benzene ring. Amide 5 is a monoacylglycerol lipase (MGL) inhibitor developed by Janssen as an analgesic29 and salts of fendizoic acid (7) are sold as the cough suppressant cloperastine fendizoate.30 Starting from BCH 2w, conversion of the methyl ester moiety into a N,N-dimethylamide led to amide 3, which served as a shared intermediate towards BCHs 4 and 6. Methylation of the alcohol led to methyl ether 3 and addition of phenyl lithium into the amide moiety provided aryl ketone 6. Additionally, derivatisation of some of the compounds prepared was also possible (Scheme 4B).31 Reductive cleavage of the Evans auxiliary allowed conversion of (+)-2r to alcohol (+)-8 and provides an entry to enantioenriched BCHs. Acylation of the pendant alcohol of 2q was possible in 96% yield, as was saponification of the ethyl ester in 2u.17b
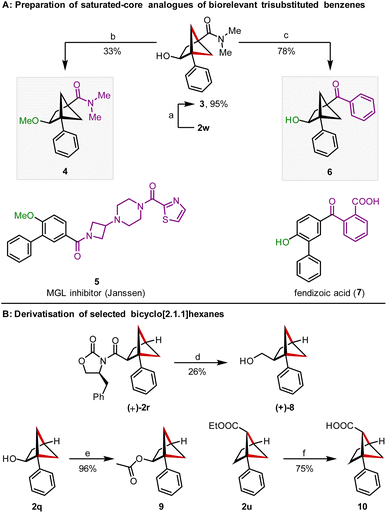 |
| Scheme 4 The preparation of saturated analogues of MGL inhibitor 7 and fendizoic acid (9) and derivatization of selected bicyclo[2.1.1]hexanes. Reagents and conditions: (a) Me2NH (2 equiv), iPrMgCl (3.5 equiv), THF, −5 °C → RT, 2 h, 95%; (b) NaH (2 equiv), MeI (2 equiv), THF, RT, 4 days, 33%; (c) PhLi (3 equiv), THF, 0 °C, 45 min, 78%; (d) LiAlH4 (2.1 equiv), Et2O, 0 °C → RT, overnight, 26%; (e) Ac2O (5 equiv), Et3N (5 equiv), DMAP (0.5 equiv), CH2Cl2, RT, overnight, 96%; (f) NaOH (3.2 equiv), EtOH : H2O (4 : 1), RT, overnight, 75%. | |
Finally, attention turned to investigating the reaction mechanism. Using cyclic voltammetry (for details see ESI†), the oxidation potential of diene 1a was measured to be E(1a+/1a) = +1.55 V vs. SCE, outside the range of the Iridium photocatalyst [E(Ir*/Ir−) = +1.21 V vs. SCE] (Fig. 1A).32 The reduction potential of 1a was measured to be E(1a/1a−) = −2.70 V vs. SCE, again outside the range of the Iridium photocatalyst [E(Ir*/Ir+) = −0.89 V vs. SCE].32 This suggests that a redox mechanism is unlikely. However, the reported excited state energy of styrenes lies at approximately 61.7 kcal mol−1,33 which should be accessible to the photocatalyst (excited state energy = 61.8 kcal mol−1).33 In line with this, addition of the known triplet quencher isoprene led to a reduced conversion of diene 1a (Fig. 1B). We therefore propose the following mechanism for the reaction (Fig. 1C). The iridium photocatalyst is excited by the 456 nm LEDs and energy transfer from the photoexcited state to diene 1a leads to photoexcited 1a*. The diradical nature of this intermediate leads to a 5-exo-trig cyclisation to diradical 11 and lastly, radical recombination gives BCH 2a.
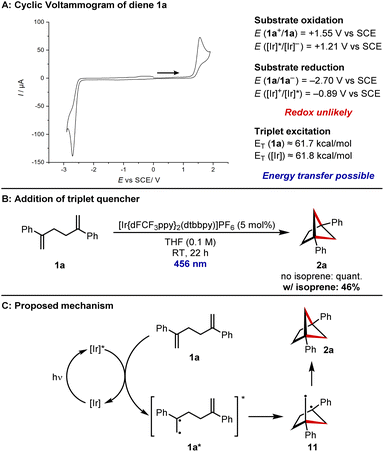 |
| Fig. 1 Mechanistic studies and proposed mechanism. (A) Cyclic voltammogram of diene 1a and comparison of redox potentials to those of the photoexcited catalyst (for experimental details see ESI†). (B) Control experiment with the addition of the triplet quencher isoprene. (C) Proposed mechanism for the [2 + 2] cycloaddition reaction. | |
Conclusions
In conclusion, we have developed a visible light-mediated photocatalytic [2 + 2] cycloaddition reaction of 1,5-hexadienes that provides unified and flexible access to BCHs containing 11 different substitution patterns. Structures that could be used as ortho-, meta-, and polysubstituted benzene bioisosteres can be prepared, along with BCHs with substituent geometries not found in aromatic chemical space. Importantly, the method allows for the predictable installation of rare bridge substituents on the BCH scaffold, and through judicious design of the starting 1,5-hexadiene, the location of the bridge substituent in the final structure can be controlled. The method speaks to the current desire for reactions that enable rapid complexity generation in three-dimensional space and we hope it will find application in the near future.
Data availability
The datasets supporting this article have been uploaded as part of the ESI.†
Author contributions
J. C. L. W. conceptualised the project. M. R., J. S., and J. C. L. W. conceptualised and performed the experiments. C. G. performed the X-ray analyses. M. R. and J. C. L. W. wrote the original draft of the manuscript and all authors were involved in review and editing of the manuscript and have given approval to the final version of the manuscript.
Conflicts of interest
There are no conflicts to declare.
Acknowledgements
We wish to thank Martin Simon, University of Göttingen for his assistance with HPLC chromatography. M. R thanks the Stiftung Industrieforschung for a scholarship. J. C. L. W. thanks the Fonds der Chemischen Industrie for the award of a Material Cost Allowance. J. C. L. W and M. R thank the Georg-August-Universität Göttingen for financial support. J. C. L. W thanks Prof. Manuel Alcarazo for his continuous generous support and guidance.
Notes and references
-
(a) D. G. Brown and J. Boström, J. Med. Chem., 2016, 59, 4443–4458 CrossRef CAS PubMed;
(b) J. Boström, D. G. Brown, R. J. Young and G. M. Keserü, Nat. Rev. Drug Discovery, 2018, 17, 709–727 CrossRef PubMed;
(c) D. C. Blakemore, L. Castro, I. Churcher, D. C. Rees, A. W. Thomas, D. M. Wilson and A. Wood, Nat. Chem., 2018, 10, 383–394 CrossRef CAS PubMed.
-
(a) F. Lovering, J. Bikker and C. Humblet, J. Med. Chem., 2009, 52, 6752–6756 CrossRef CAS PubMed;
(b) F. Lovering, Med. Chem. Commun., 2013, 4, 515–519 RSC.
- For reviews on the application of cubanes in medicinal chemistry, see:
(a) T. A. Reekie, C. M. Williams, L. M. Rendina and M. Kassiou, J. Med. Chem., 2019, 62, 1078–1095 CrossRef CAS PubMed;
(b) K. F. Biegasiewicz, J. R. Griffiths, G. P. Savage, J. Tsanaktsidis and R. Priefer, Chem. Rev., 2015, 115, 6719–6745 CrossRef CAS PubMed;
(c) G. W. Griffin and A. P. Marchand, Chem. Rev., 1989, 89, 997–1010 CrossRef CAS.
-
(a) B. A. Chalmers, H. Xing, S. Houston, C. Clark, S. Ghassabian, A. Kuo, B. Cao, A. Reitsma, C.-E. P. Murray, J. E. Stok, G. M. Boyle, C. J. Pierce, S. W. Littler, D. A. Winkler, P. V. Bernhardt, C. Pasay, J. J. De Voss, J. McCarthy, P. G. Parsons, G. H. Walter, M. T. Smith, H. M. Cooper, S. K. Nilsson, J. Tsanaktsidis, G. P. Savage and C. M. Williams, Angew. Chem., Int. Ed., 2016, 55, 3580–3585 CrossRef CAS PubMed;
(b) S. D. Houston, T. Fahrenhorst-Jones, H. Xing, B. A. Chalmers, M. L. Sykes, J. E. Stok, C. Farfan Soto, J. M. Burns, P. V. Bernhardt, J. J. De Voss, G. M. Boyle, M. T. Smith, J. Tsanaktsidis, G. P. Savage, V. M. Avery and C. M. Williams, Org. Biomol. Chem., 2019, 17, 6790–6798 RSC.
- For reviews on the chemistry of bicyclo[1.1.1]pentanes, see:
(a) J. Turkowska, J. Durka and D. Gryko, Chem. Commun., 2020, 56, 5718–5734 RSC;
(b) X. Ma and L. N. Pham, Asian J. Org. Chem., 2020, 9, 8–22 CrossRef CAS;
(c) J. Kanazawa and M. Uchiyama, Synlett, 2019, 30, 1–11 CrossRef CAS;
(d) M. D. Levin, P. Kaszynski and J. Michl, Chem. Rev., 2000, 100, 169–234 CrossRef CAS PubMed;
(e) E. W. Delia and I. J. Lochert, Org. Prep. Proced. Int., 1996, 28, 411–441 CrossRef.
- N. D. Measom, K. D. Down, D. J. Hirst, C. Jamieson, E. S. Manas, V. K. Patel and D. O. Somers, ACS Med. Chem. Lett., 2017, 8, 43–48 CrossRef CAS PubMed.
-
(a) N. Frank, J. Nugent, B. R. Shire, H. D. Pickford, P. Rabe, A. J. Sterling, T. Zarganes-Tzitzikas, T. Grimes, A. L. Thompson, R. C. Smith, C. J. Schofield, P. E. Brennan, F. Duarte and E. A. Anderson, Nature, 2022, 611, 721–726 CrossRef CAS PubMed;
(b) T. Iida, J. Kanazawa, T. Matsunaga, K. Miyamoto, K. Hirano and M. Uchiyama, J. Am. Chem. Soc., 2022, 144, 21848–21852 CrossRef CAS PubMed.
-
(a) T. Rigotti and T. Bach, Org. Lett., 2022, 24, 8821–8825 CrossRef CAS PubMed;
(b) V. V. Levterov, Y. Panasyuk, V. O. Pivnytska and P. K. Mykhailiuk, Angew. Chem., Int. Ed., 2020, 59, 7161–7167 CrossRef CAS PubMed.
- For general reviews on the concept of bioisosteres in medicinal chemistry, see:
(a) M. A. M. Subbaiah and N. A. Meanwell, J. Med. Chem., 2021, 64, 14046–14128 CrossRef CAS PubMed;
(b) E. G. Tse, S. D. Houston, C. M. Williams, G. P. Savage, L. M. Rendina, I. Hallyburton, M. Anderson, R. Sharma, G. S. Walker, R. S. Obach and M. H. Todd, J. Med. Chem., 2020, 63, 11585–11601 CrossRef CAS PubMed;
(c) P. K. Mykhailiuk, Org. Biomol. Chem., 2019, 17, 2839–2849 RSC;
(d) G. M. Locke, S. S. R. Bernhard and M. O. Senge, Chem. – Eur. J., 2019, 25, 4590–4647 CrossRef CAS PubMed;
(e) Y. P. Auberson, C. Brocklehurst, M. Furegati, T. C. Fessard, G. Koch, A. Decker, L. La Vecchia and E. Briard, ChemMedChem, 2017, 12, 590–598 CrossRef CAS PubMed.
- A recent report also demonstrated that bicyclo[2.2.0]hexanes can be used as meta-benzene bioisosteres, see: R. C. Epplin, S. Paul, L. Herter, C. Salome, E. N. Hancock, J. F. Larrow, E. W. Baum, D. R. Dunstan, C. Ginsburg-Moraff, T. C. Fessard and M. K. Brown, Nat. Commun., 2022, 13, 6056 CrossRef CAS PubMed.
- According to a search of the database https://www.drugbank.ca on 21/02/2023.
- A. Nilova, L.-C. Campeau, E. C. Sherer and D. R. Stuart, J. Med. Chem., 2020, 63, 13389–13396 CrossRef CAS PubMed.
-
(a) O. L. Garry, M. Heilmann, J. Chen, Y. Liang, X. Zhang, X. Ma, C. S. Yeung, D. J. Bennett and D. W. C. MacMillan, J. Am. Chem. Soc., 2023, 145, 3092–3100 CrossRef CAS PubMed;
(b) J. M. Anderson, N. D. Measom, J. A. Murphy and D. L. Poole, Org. Lett., 2023, 25, 2053–2057 CrossRef CAS PubMed.
- For a review of routes to bridge-functionalised bicyclo[1.1.1]pentanes, see: J. M. Anderson, N. D. Measom, J. A. Murphy and D. L. Poole, Angew. Chem., Int. Ed., 2021, 60, 24754–24769 CrossRef CAS PubMed.
- For recent examples of cubane functionalization, see:
(a) Y. Kato, C. M. Williams, M. Uchiyama and S. Matsubara, Org. Lett., 2019, 21, 473–475 CrossRef CAS PubMed;
(b) R. Okude, G. Mori, A. Yagi and K. Itami, Chem. Sci., 2020, 11, 7672–7675 RSC;
(c) N. Yoshino, Y. Kato, T. Mabit, Y. Nagata, C. M. Williams, M. Harada, A. Muranaka, M. Uchiyama and S. Matsubara, Org. Lett., 2020, 22, 4083–4087 CrossRef CAS PubMed;
(d) D. E. Collin, K. Kovacic, M. E. Light and B. Linclau, Org. Lett., 2021, 23, 5164–5169 CrossRef CAS PubMed;
(e) H. Takebe, N. Yoshino, Y. Shimada, C. M. Williams and S. Matsubara, Org. Lett., 2023, 25, 27–30 CrossRef CAS PubMed;
(f) M. P. Wiesenfeldt, J. A. Rossi-Ashton, I. B. Perry, J. Diesel, O. L. Garry, F. Bartels, S. C. Coote, X. Ma, C. S. Yeung, D. J. Bennett and D. W. C. MacMillan, Nature, 2023, 618, 513–518 CrossRef CAS PubMed.
-
(a) C. Yang, W. Xa, J. R. Scheffer and M. Botoshansky, Angew. Chem., Int. Ed., 2005, 44, 5087–5089 CrossRef CAS PubMed;
(b) G. Zhao, C. Yang, Q. Chen, J. Jin, X. Zhang, L. Zhao and W. Xa, Tetrahedron, 2009, 65, 9952–9955 CrossRef CAS;
(c) L. Herter, I. Koutsopetras, L. Turelli, T. Fessard and C. Salomé, Org. Biomol. Chem., 2022, 20, 9108–9111 RSC.
- For recent examples, see:
(a) D. Staveness, T. M. Sodano, K. Li, E. A. Burnham, K. D. Jackson and C. R. J. Stephenson, Chem, 2019, 5, 215–226 CrossRef CAS PubMed;
(b) A. Denisenko, P. Garbuz, S. V. Shishkina, N. M. Voloshchuk and P. K. Mykhailiuk, Angew. Chem., Int. Ed., 2020, 59, 20515–20521 CrossRef CAS PubMed;
(c) X. Ma, Y. Han and D. J. Bennett, Org. Lett., 2020, 22, 9133–9138 CrossRef CAS PubMed;
(d) A. S. Harmata, T. E. Spiller, M. J. Sowden and C. R. J. Stephenson, J. Am. Chem. Soc., 2021, 143, 21223–21228 CrossRef CAS PubMed;
(e) Y. Yang, J. Tsien, J. M. E. Hughes, B. K. Peters, R. R. Merchant and T. Qin, Nat. Chem., 2021, 13, 950–955 CrossRef CAS PubMed;
(f) J.-X. Zhao, Y.-X. Chang, C. He, B. J. Burke, M. R. Collins, M. Del Bel, J. Elleraas, G. M. Gallego, T. P. Montgomery, J. J. Mousseau, S. K. Nair, M. A. Perry, J. E. Spangler, J. C. Vantourout and P. S. Baran, Proc. Natl. Acad. Sci. U.S.A., 2021, 118, e2108881118 CrossRef CAS PubMed;
(g) B. A. Wright, A. Matviitsuk, M. J. Black, P. García-Reynaga, L. E. Hanna, A. T. Herrmann, M. K. Ameriks, R. Sarpong and T. P. Lebold, J. Am. Chem. Soc., 2023, 145, 10960–10966 CrossRef CAS PubMed.
-
(a) A. Cairncross and E. P. Blanchard Jr., J. Am. Chem. Soc., 1966, 88, 496–504 CrossRef CAS;
(b) A. de Meijere, H. Wenck, F. Seyed-Mahdavi, H. G. Viehe, V. Gallez and I. Erden, Tetrahedron, 1986, 42, 1291–1297 CrossRef CAS;
(c) P. Wipf and M. A. A. Walczak, Angew. Chem., Int. Ed., 2006, 45, 4172–4175 CrossRef CAS PubMed;
(d) R. Kleinmans, T. Pinkert, S. Dutta, T. O. Paulisch, H. Keum, C. G. Daniliuc and F. Glorius, Nature, 2022, 605, 477–482 CrossRef CAS PubMed;
(e) R. Guo, Y.-C. Chang, L. Herter, C. Salome, S. E. Braley, T. C. Fessard and M. K. Brown, J. Am. Chem. Soc., 2022, 144, 7988–7994 CrossRef CAS PubMed;
(f) Y. Zheng, W. Huang, R. K. Dhungana, A. Granados, S. Keess, M. Makvandi and G. A. Molander, J. Am. Chem. Soc., 2022, 144, 23685–23690 CrossRef CAS PubMed;
(g) M. Xu, Z. Wang, Z. Sun, Z. Ouyang, Z. Ding, T. Yu, L. Xu and P. Li, Angew. Chem., Int. Ed., 2022, 61, e202214507 CrossRef CAS PubMed;
(h) Y. Liang, R. Kleinmans, C. G. Daniliuc and F. Glorius, J. Am. Chem. Soc., 2022, 144, 20207–20213 CrossRef CAS PubMed;
(i) S. Agasti, F. Beltran, E. Pye, N. Kaltsoyannis, G. E. M. Crisenza and D. J. Procter, Nat. Chem., 2023, 15, 535–541 CrossRef CAS PubMed;
(j) T. V. T. Nguyen, A. Bossonnet and J. Waser, ChemRxiv, 2023 DOI:10.26434/chemrxiv-2023-s8j30;
(k) Y. Liu, S. Lin, Y. Li, J.-H. Xue, Q. Li and H. Wang, ACS Catal., 2023, 13, 5096–5103 CrossRef CAS.
- For a review on the early examples of this type of reactivity, see: M. Golfmann and J. C. L. Walker, Commun. Chem., 2023, 6, 9 CrossRef CAS PubMed.
- M. Golfmann, L. Glagow, A. Giakoumidakis, C. Golz and J. C. L. Walker, Chem. – Eur. J., 2023, 29, e202202373 CrossRef CAS PubMed.
-
(a) K. J. Crowley, J. Am. Chem. Soc., 1964, 86, 5692–5693 CrossRef CAS;
(b) R. Srinivasan and K. H. Carlough, J. Am. Chem. Soc., 1967, 89, 4932–4936 CrossRef CAS;
(c) J. K. Crandall and C. F. Mayer, J. Org. Chem., 1970, 35, 3049–3053 CrossRef CAS;
(d) R. A. Kleinnijenhuis, B. J. J. Timmer, G. Lutteke, J. M. M. Smits, R. de Gelder, J. H. van Maarseveen and H. Hiemstra, Chem. – Eur. J., 2016, 22, 1266–1269 CrossRef CAS PubMed.
-
(a) R. S. H. Liu and G. S. Hammond, J. Am. Chem. Soc., 1967, 89, 4936–4944 CrossRef CAS;
(b) C. Yamada, M. Pahk and R. Liu, J. Chem. Soc. D, 1970, 882 RSC;
(c) J. J. Bloomfield and D. C. Owsley, Tetrahedron Lett., 1973, 21, 1795–1798 CrossRef;
(d) W. Adam, S. Grabowski, H. Platsch, K. Hannemann, J. Wirz and R. M. Wilson, J. Am. Chem. Soc., 1989, 111, 751–753 CrossRef CAS;
(e) A. Barbero, C. García and F. J. Pulido, Synlett, 2001, 6, 824–826 CrossRef.
- Kwon and co-workers reported a related route to benzobicyclo[3.1.1]heptanones, see: J. Zhao, J. L. Brosmer, Q. Zang, Z. Yang, K. N. Houk, P. L. Diaconescu and O. Kwon, J. Am. Chem. Soc., 2017, 139, 9807–9810 CrossRef CAS PubMed.
- Related crossed [2+2] cycloadditions to heterocyclic BCHs are also known. For selected recent examples, see:
(a) V. V. Levterov, Y. Panasyuk, V. O. Pivnytska and P. K. Mykhailiuk, Angew. Chem., Int. Ed., 2020, 59, 7161–7167 CrossRef CAS PubMed;
(b) T. Rigotti, D. P. Schwinger, R. Graßl, C. Jandl and T. Bach, Chem. Sci., 2022, 13, 2378–2384 RSC.
- We propose that the difference in endo
:
exo selectivity compared to 2u is derived from the different geometry of the respective dienes. Diene 1u was prepared in 98
:2 (Z
:
E) selectivity and led to 2u in 10
:
1 (endo
:
exo) selectivity. Diene 1z was prepared in >99
:
1 (E
:
Z) selectivity and led to 2z in 2
:
1 (exo
:
endo) selectivity. Mykhailiuk and co-workers (ref. 17b) also observed high 9
:
1 (endo
:
exo) selectivity for 2u, but starting from a 1
:
1 mixture of isomers. Plausible transition states from the Z- and E-dienes do lead to the endo and exo products respectively (see ESI†). We believe the following explanation would be consistent with the experimental observations: (1) E → Z isomerisation is a side reaction. (2) From the 1
:
1 E
:
Z mixture of 1u (ref. 17b), near complete E → Z isomerisation occurs before cyclisation and leads to high endo selectivity. (3) From the E-only isomer of 1z, only partial isomerisation occurs before cyclisation, leading to the mixture of exo and endo products..
-
(a) X. Ma, D. L. Sloman, Y. Han and D. J. Bennett, Org. Lett., 2019, 21, 7199–7203 CrossRef CAS PubMed;
(b) R. M. Bychek, V. Hutskalova, Y. P. Bas, O. A. Zaporozhets, S. Zozulya, V. V. Levterov and P. K. Mykhailiuk, J. Org. Chem., 2019, 84, 15106–15117 CrossRef CAS PubMed;
(c) X. Ma, Y. Han and D. J. Bennett, Org. Lett., 2020, 22, 9133–9138 CrossRef CAS PubMed.
- C. F. R. A. C. Lima, L. R. Gomes, L. M. N. B. F. Santos and J. N. Low, CSD Communication, 2012, 782202, DOI:10.5517/ccv7ybb.
-
(a) J.-R. Wang, X. Wang, L. Lu and X. Mei, Cryst. Growth Des., 2013, 13, 3261–3269 CrossRef CAS;
(b) R. Chadha, S. Bhandari, J. Haneef, S. Khullar and S. Mandal, CrystEngComm, 2014, 16, 8375–8389 RSC.
-
H. Bian, K. M. Chevalier, P. J. Connolly, C. M. Flores, S.-c. Lin, L. Liu, J. Mabus, M. J. Macielag, M. E. McDonnell, P. M. Pitis, Y.-m. Zhang, S.-p. Zhang, J. Clemente and B. Zhu, Janssen Pharmaceutica, World Intellectual Property Organisation, WO 2010/124114 A1, 2010 Search PubMed.
- M. A. Catania and S. Cuzzocrea, Ther. Clin. Risk Manage., 2011, 7, 83–92 Search PubMed.
- During preparation of this manuscript a further report on [2 + 2] crossed cycloadditions was published. The authors also reported a range of derivatisation reactions of the BCH scaffold, see: S. Paul, D. Adelfinsky, C. Salome, T. Fessard and M. K. Brown, Chem. Sci., 2023, 14, 8070–8075 RSC.
- C. K. Prier, D. A. Rankic and D. W. C. MacMillan, Chem. Rev., 2013, 113, 5322–5363 CrossRef CAS PubMed.
- F. Strieth-Kalthoff, M. J. James, M. Teders, L. Pitzer and F. Glorius, Chem. Soc. Rev., 2018, 47, 7190–7202 RSC.
|
This journal is © The Royal Society of Chemistry 2023 |
Click here to see how this site uses Cookies. View our privacy policy here.