DOI:
10.1039/D2SD00158F
(Paper)
Sens. Diagn., 2023,
2, 168-175
Salicylaldehyde-based molecular sensor with one facile step toward visual detection of viscosity†
Received
2nd September 2022
, Accepted 14th October 2022
First published on 17th November 2022
Abstract
Liquid safety has become one of the urgent affairs in global public health studies, and a noninvasive and effective viscosity inspection method is expected to ease the burden of continuously increasing health problems. In this study, one kind of activatable molecular sensor (DTPMP) based on a salicylaldehyde derivate has been synthesized via the Schiff base condensation reaction. This sensor is comprised of a donor–acceptor conjugated structure. We proposed the viscosity of the liquid microenvironment as a marker, and a rotatable conjugate structure was utilized as the recognition site. The molecular sensor was synthesized in a one-step facile way, and DTPMP displayed a longer emission wavelength and larger Stokes shift (142.5 nm in water, 116.8 nm in glycerol), which endows the sensor with the capacity of avoiding auto-fluorescence and achieving high signal-to-noise ratio imaging. The rotation of aromatic rings in DTPMP is restricted in a high-viscosity microenvironment, with the gradually increasing fluorescence emission signal at 532 nm. Moreover, the DTPMP sensor exhibits high adaptability, selectivity, sensitivity, and good photostability in various commercial liquids. Significantly, this sensor has successfully been applied not only in the determination of the thickening effects of food gum but also in the detection of viscosity enhancement during the liquid food spoilage process, which may be helpful in screening the metamorphic stage. We expected that this unique strategy will reinvigorate the continued perfection of the fluorescence technique toward liquid safety investigation.
Introduction
Viscosity is an essential microenvironment parameter in various liquid systems, not only in biological systems but also in commercial liquid beverages.1–4 Changes in liquid viscosity are related to some extent of deterioration, since various nutritious additives, vitamins, glucose, and amino acids exist in the beverages, which could induce the growth of bacteria, mould, and yeast.5,6 As a vital physical marker of liquid, the detection of microenvironment viscosity remains one of the challenges since various traditional analytical methods (including the capillary viscometer, rheometer, damping vibration viscometer, etc.) can only be used to measure the macroscopic viscosity.7–9 Complex pre-treatment procedures, longer test times, and destructiveness toward the sample are usually connected with these traditional methods.10,11 To this end, it is urgent to develop an effective strategy for monitoring liquid viscosity at the molecular level, especially without strongly depending on the device, and avoiding shortcomings.
A fluorescence imaging technique has intrinsic advantages, possessing excellent sensitivity, visualization, high sensitivity, in situ detection, etc. So far, most fluorescent probes have been reported in the biological field, such as mitochondrial viscosity tracking,12 lysosomal viscosity investigation,13 and physiological viscosity determination.14 They have several superior properties, but their applications are hindered due to the limitations associated with a short Stokes shift, a lower signal-to-noise ratio, and a lower viscosity coefficient, as shown in Table S1 (ESI†). Recently, fluorescent probes used for liquid safety have attracted attention as well, such as in toxic volatile amine investigation,15 allergens determination,16 metal cation and anion detection,17etc. However, a functional molecular tool for viscosity detection as one kind of physical indicator is still lacking. Consequently, it is essential to develop an effective fluorescent sensor with a large Stokes shift for exploring viscosity fluctuations in a commercial liquid system.
In this work, we designed a highly sensitive fluorescent sensor DTPMP for viscosity detection. This sensor was constructed from a diethylamine salicylaldehyde derivate (donor, D) and phenyl carbon trifluoride (acceptor, A) through the Schiff base condensation reaction. With the existence of a Schiff base, the intramolecular conjugate system was expanded, and the hydroxyl group may further cause a distinct red-shifting phenomenon. A typical intramolecular charge transfer (ICT) platform was constructed, and it was hypothesized that DTPMP would be able to undergo intramolecular D–A twisting around the single bond between D and A; the conversion between the locally excited state and stabilized ICT state could hold great promise toward viscosity sensing.18 The twisted intramolecular charge transfer (TICT) mechanism may afford this. With increases in environmental viscosity, the sensor exhibited a 97-fold fluorescence increase at 532 nm, and a turn-on mode was found. The optical experimental test confirmed that the sensor could be used as a powerful tool for viscosity variation determination, due to its good adaptability, photostability, and excellent specificity in various analytes. A higher signal-to-noise ratio released signal toward thickening efficiency inspection further demonstrated the potential in viscosity checking. Accordingly, the ability of DTPMP to detect viscosity changes in the liquid deterioration process was investigated through the combination of the molecular tool with the fluorescence technique.
Experimental
Materials and methods
Dimethylsulfoxide (DMSO), ethyl acetate (EA), 4-(trifluoromethyl)aniline, 4-(diethylamino)-2-hydroxybenzaldehyde, sodium sulfate (Na2SO4), glycerol, acetonitrile, methanol (MeOH), tetrahydrofuran (THF), dichloromethane (DCM), petroleum ether, ethanol, and various metal salts, such as the potassium nitrate, potassium carbonate, sodium chloride, magnesium chloride, etc., were purchased from Aladdin Reagent (Shanghai) Co., Ltd. Common food additives including glucose (Glu), vitamin C (VC), D-mannitol, acesulfame, sorbitol, sodium benzoate (SB), beet molasses (BM), trisodium citrate dehydrate (TCD), sodium carboxymethyl cellulose (SCC), pectin (Pec), xanthan gum (XG) were obtained from Energy Chemical Technology (Shanghai) Co., Ltd. All the chemical reagents used in this work were of analytical grade. Triple distilled water was used in the experiments.
Nuclear magnetic resonance (NMR) spectra were obtained with a Bruker AVANCE III HD 400 NMR Spectrometer. Fluorescence spectra were measured using a Hitachi F-7000 fluorescence spectrophotometer. High-resolution mass spectra were obtained through an Agilent 7250 & JEOL-JMS-T100LP AccuTOF mass spectrometer. The viscosity was determined using a rotating viscometer (DV2T, Brookfield, AMETEK Corp., USA). Absorption spectra were recorded on a Hitachi U-3010 UV-vis spectrophotometer.
Synthetic procedures
The synthetic procedure of molecular sensor DTPMP.
4-(Trifluoromethyl)aniline (161.0 mg, 1.0 mM) was dissolved in ethanol, and the 4-(diethylamino)-2-hydroxybenzaldehyde (241.4 mg, 1.25 mM) was dissolved in ethanol as well, and both solution were mixed. The mixture was stirred at room temperature for half an hour under an N2 atmosphere. Then the reaction system was refluxed at 78 °C, and the reaction was monitored by thin-layer chromatography (TLC). When the reaction was finished, the solvent was removed under reduced pressure and extracted in the DCM/de-ionized water, the crude product was purified through silica-gel column chromatography using DCM/petroleum ether (v/v = 1/3), and the molecular sensor DTPMP as a bright tangerine powder was obtained (302.5 mg, 90%). 1H NMR (400 MHz, CDCl3) δ 13.38 (s, 1H), 8.40 (s, 1H), 7.61 (d, J = 8.4 Hz, 2H), 7.28 (d, J = 8.3 Hz, 1H), 7.16 (d, J = 8.8 Hz, 1H), 6.26 (dd, J = 8.8, 2.4 Hz, 1H), 6.19 (d, J = 2.4 Hz, 1H), 3.41 (q, J = 7.1 Hz, 4H), 1.21 (t, J = 7.1 Hz, 6H). 13C NMR (101 MHz, CDCl3) δ 164.07, 161.89, 152.37, 152.21, 134.22, 128.40, 126.46, 123.00, 121.06, 108.99, 104.17, 97.72, 44.66, 12.69. MS (ESI): m/z 337.15287 [M + H]+, calcd for C18H19F3N2O 336.14495.
Optical property investigation
The molecular sensor DTPMP was dissolved in DMSO to prepare the stock solution with the concentration of 1 mM and stored in the dark at a temperature of 5 °C. During the test procedure, the concentrations of DTPMP were controlled at 10 μM. The viscosity determination procedure was as follows: the sensor was added into a 3 mL solution consisting of various volume percentages of distilled water and glycerol (from 0% to 99%, including 1% DMSO), and fluorescence spectroscopy and UV-vis absorption measurements were carried out in this mixture system. The corresponding viscosity values were recorded and measured using a viscometer.
Solvents with various polarities, such as methanol, DMSO, DMF, etc., were selected to test the emission properties of molecular sensor DTPMP in the complex solvent environment. DTPMP was added to the solvents and shaken before the test. In the specificity test, solutions with various potential interfering analytes (including NaCl, MgCl2, glucose, VC, etc.) were prepared with distilled water, and DTPMP was added in each interfering test. Before the test, the solutions were shaken uniformly. In the temperature effect experiment, DTPMP was added to glycerol containing 1% DMSO at different temperatures, such as the normal body temperature (37 °C), fresh-keeping temperature (∼5 °C), and common room temperature (25 °C). In all measurements, the mixed solutions were transferred to the quartz cell. The excitation wavelength was set as 420 nm.
Common food thickeners including sodium carboxymethyl cellulose, pectin, and xanthan gum with different mass concentrations (from 1 g kg−1 to 5 g kg−1) were added into the distilled water with ultrasonic dispersion, and the corresponding thickening solutions were prepared. Before the test, bubbles in the solution were removed, and the stock solution of sensor DTPMP was diluted as 10 μM. The excitation wavelength was 420 nm, and emission spectra were recorded from 450 nm to 780 nm (Scheme 1).
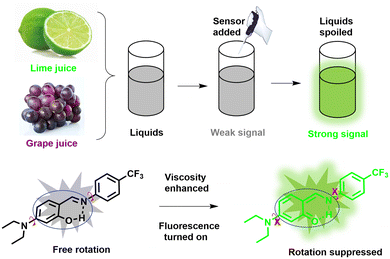 |
| Scheme 1 Viscosity sensing mechanism of the molecular sensor DTPMP toward the liquid deterioration tracking process. | |
Viscosity checking process
For liquid deterioration process tracking, two kinds of commercial liquids purchased from the local supermarket, including lime juice and grape juice, were stored at different storage temperatures (ambient temperature & fresh-maintenance temperature) and exposed to air for one week. The fluorescence emission spectra were recorded at different time intervals (on day 0, day 2, day 5, and day 7). The relationship between the fluorescence intensity enhancement and viscosity variation was established as the following equation: (Fn − F0)/F0 ∼ (ηn − η0)/η0, in which ηn and η0 were defined as the viscosity of liquids at day 0 and day n (0 < n < 8), and F0 and Fn were the fluorescence intensity of the beverages at day 0 and day n (0 < n < 8).
The Förster–Hoffmann equation
According to previous studies,19,20 the relationship between the fluorescence intensity of the molecular sensor DTPMP and the viscosity can be determined by the following Förster–Hoffmann equation:
where η represents the viscosity, I represents the fluorescence intensity of the molecular sensor DTPMP at 532 nm, C is a constant, and x represents the sensitivity of the molecular sensor toward viscosity.
Results and discussion
Molecular sensor design and synthesis
To control its photoluminescence behaviour in various liquid systems, ingenious design of a powerful optical sensor for viscosity monitoring is highly desirable. We expect that the designed sensor should exhibit stronger fluorescence in higher-viscosity media while emitting weaker fluorescence in lower-viscosity media. In this case, the viscosity enhancement in liquids can be captured. Another problem in designing sensors is avoiding auto-fluorescence. Thus, a larger Stokes shift is necessary.21 Herein, the molecular sensor DTPMP was readily prepared via the Schiff base dehydration condensation reaction in a one-step facile way, as shown in Fig. 1a. The chemical structure and detailed relative molecular mass information are shown in Fig. S1–S3 (ESI†). Commonly, viscosity probes are constructed based on intramolecular rotation and contain both donors and acceptors. As we know, salicylaldehyde is a widely used fluorophore due to its excellent practical advantages, and with the incorporation of a diethylamine group, a typical donor group can be established.22 And in terms of the acceptor group, phenyl carbon trifluoride has attracted much attention in recent years due to its excellent photophysical properties, outstanding food compatibility, and low toxicity to liquid systems. Based on this, a smart fluorescent sensor DTPMP for sensing viscosity was constructed by combining a salicylaldehyde derivate and phenyl carbon trifluoride. We hypothesized that the introduction of a hydroxyl group could improve electron-donating capacity, and a typical excited-state intramolecular proton transfer (ESIPT) effect existed between the hydroxyl and Schiff base groups.23,24 Thus, the conjugated system can be extended, and the fluorescence performance of the fluorophore can be improved. We envisioned that the sensor DTPMP showed an obvious turn-on signal in high-viscosity media, since the rotation was restricted in the high viscous microenvironment, and the fluorescence was released via the radiative transition way.25 While in a low-viscosity microenvironment, an opposite phenomenon occurred. As a consequence, it is anticipated that molecular sensor DTPMP may be able to discriminate viscosity changes in liquids.
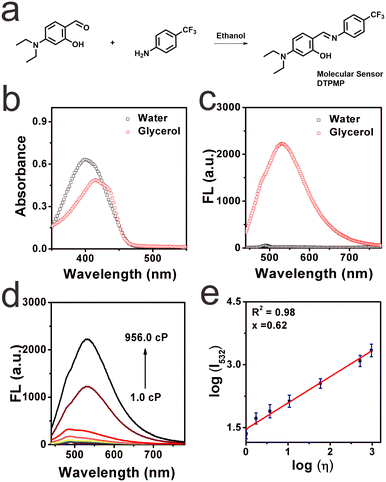 |
| Fig. 1 (a) Synthesis route for the molecular sensor DTPMP. (b) Absorption spectra of the sensor DTPMP in water and glycerol. (c) Fluorescence spectra of the sensor DTPMP in water and glycerol. (d) Fluorescence emissive spectra of the sensor DTPMP in a water–glycerol mixture with the fraction of glycerol (fg) from 0% to 99%. (e) A linear relationship between log I532 and log η. λex = 420 nm, and the concentration of DTPMP is 10 μM. | |
Optical properties toward viscosity
After the successful preparation of the probe, its optical properties in the glycerol and water were studied first, respectively. As shown in Fig. 1b, the main absorption peak of sensor DTPMP appeared at 401 nm in pure water; however, the absorption in glycerol redshifted by 14 nm, at which the absorption bathochromically shifted toward 415 nm. This may be attributed to the fact that DTPMP can adopt a more planar configuration to expand its binding degree in viscous media compared with non-viscous environments. Then, the fluorescence spectra were recorded as well, as displayed in Fig. 1c. Obviously, DTPMP showed almost no fluorescence in pure water with an excitation of 420 nm. By contrast, a strong fluorescence signal was found in the high-viscosity glycerol solution. Furthermore, fluorescence responsiveness in different viscosity media was investigated in detail, as shown in Fig. 1d, the fluorescence intensity of sensor DTPMP was gradually enhanced upon increasing the content of glycerol (from 1.0 cP to 956.0 cP), and in 99% glycerol, the fluorescence intensity dramatically reached the maximum and increased by 97-fold. We found a linear relationship with the viscosity (log
η) (R2 = 98%), which was derived from the Förster–Hoffmann equation (Fig. 1e), which revealed that DTPMP was able to quantitatively determine the viscosity. Moreover, the viscosity sensitivity coefficient x of sensor DTPMP was 0.62. And the detection limit of this sensor for viscosity determination was investigated as well, which was found to be 1.220 cP, as seen in Fig. S4 (ESI†). Subsequently, the results demonstrated that the molecular sensor DTPMP can efficiently respond to changes in viscosity. To support this conclusion, we tested the fluorescence response capability toward viscosity when the high-viscosity glycerol was stored at different temperatures, since the viscosity can be usually affected by temperature.26 As in Fig. S5 (ESI†), it was found that the fluorescence intensity decreased when the glycerol test system was stored at a lower temperature. Meanwhile, the fluorescence signal became stronger when the glycerol was stored at a lower temperature. This phenomenon may be attributed to the restriction of rotation in highly viscous media to some degree. Furthermore, the capacity of viscosity determination in various common liquids has been investigated, as shown in Fig. S6 (ESI†). Eight kinds of commercial liquids were tested herein, including water, grape juice, lime juice, red pomelo juice, milk, jasmine juice, watermelon juice and edible oil. Different fluorescence intensities were displayed, which may be attributed to the viscosity variations in these liquids. And detailed fluorescence intensities are shown in Table S2 (ESI†). The viscosities of these liquids were investigated using a viscometer, and the results were inconsistent with the fluorescence intensities, as collected in Table S3 (ESI†). Based on the results, we designed a sensor DTPMP for further studies.
And the viscosity changes due to the thickeners were all investigated well since food thickeners are often contained in commercial liquids, which were used to enhance the consistency, homogeneity and stability of commercial beverages.27 Thus, three kinds of food thickeners (including sodium carboxymethyl cellulose, pectin and xanthan gum) with various amounts of 1 g kg−1 to 5 g kg−1 were dissolved in pure water. As shown in Fig. 2a and b, it can be found that the fluorescence signal was gradually enhanced with the increased addition of thickeners, and an approximately linear relationship between the mass and the concentrations of the thickener and the fluorescence intensity were observed. From these linear relationships, we found xanthan gum had the highest thickening efficiency, while sodium carboxymethyl cellulose showed the lowest. The thickening efficiency can be helpful in controlling the viscosity during the production process of liquids. Overall, the optical studies confirmed the potential in viscosity determination as a molecular tool.
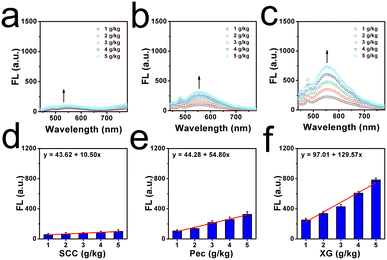 |
| Fig. 2 Fluorescence spectra of the sensor DTPMP in the presence of various mass amounts of (a) sodium carboxymethyl cellulose (SCC), (b) pectin (Pec), and (c) xanthan gum (XG). Fluorescence intensity of the sensor DTPMP and fitting line with the existence of various mass amounts of (d) SCC, (e) Pec, and (f) XG. | |
Adaptability, selectivity, and photostability investigation
High selectivity toward viscosity is crucial for its potential application in the liquid system. The effect of solvent polarity was first checked by investigating the fluorescence signals of DTPMP in various common solvents (including water, acetonitrile, DMSO, methanol, THF, toluene, ethyl acetate, and glycerol) with various polarities, as shown in Fig. 3a and b. It showed quite weak fluorescence in other solvents except for the high viscosity glycerol solution. And in the absorption spectra, the maximum absorption peak in the high-viscosity glycerol redshifted compared to the other solvents. This phenomenon may be ascribed to a more parallel stacking structure, which led to the conjugation enlargement in high viscous environments.28 The detailed photo-physical data of the molecular sensor DTPMP in different solvents are collected in Table S4 (ESI†). Moreover, the solvatochromism of the sensor DTPMP was measured in six kinds of representative solvents varying in polarity, and a typical red shift of both normalized fluorescence and absorption spectra was found (as shown in Fig. S7, ESI†), which may be attributed to the stabilization of the ICT occurring between the terminal donor and acceptor groups. And a dual emission phenomenon was found in the emission spectra, which may be caused by the typical TICT processes. These results indicated that the solvent had a very weak effect on the fluorescence emission of sensor DTPMP.
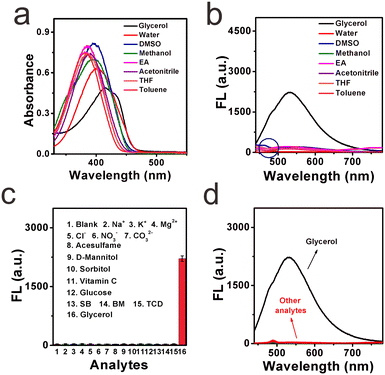 |
| Fig. 3 (a) Fluorescence emissive spectra of the sensor DTPMP in various common solvents with different polarities. (b) Absorption spectra of the sensor DTPMP in various common solvents with different polarities. (c) Selectivity of the sensor DTPMP (10 μM) toward various liquid-related analytes, including blank, Na+, K+, Mg2+, Cl−, NO3−, CO32−, acesulfame, D-mannitol, sorbitol, VC, glucose, SB, BM, TCD, and glycerol. (d) Fluorescence spectra of the sensor DTPMP in the presence of various analytes in the distilled water and the glycerol. | |
Hereafter, to demonstrate that sensor DTPMP can selectively measure viscosity normally in a complex liquid system, its properties in the presence of liquid-relevant molecules, such as various metallic cations, anions, and food additives, were further analysed, and the spectra show that no obvious fluorescence signals appeared, the fluorescence only changed significantly in the high-viscosity glycerol, as shown in Fig. 3c and d. In this case, the results demonstrate that the response of sensor DTPMP to viscosity will not be obstructed by other analytes and can be used to homogeneously monitor the viscosity changes in complex liquid environments. To avoid auto-fluorescence of the liquid itself, the Stokes shift was investigated in pure water and glycerol, respectively. As displayed in Fig. 4a and b, the Stokes shift in the pure water was found to be 142.5 nm, and data was 116.8 nm in the high-viscosity glycerol. A larger Stokes shift can be helpful to enhance the signal-to-noise ratio.29 Moreover, the data herein were found best among those of the reported studies as listed in Table S1 (ESI†). The selectivity can be confirmed and was suitable to be used in the complex microenvironment.
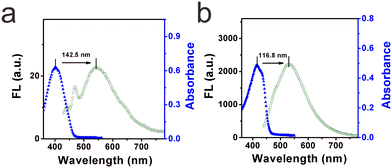 |
| Fig. 4 (a) Stokes shift of the molecular sensor DTPMP in low viscosity water (containing 1% DMSO). (b) Stokes shift of the molecular sensor DTPMP in high viscosity glycerol (containing 1% DMSO). | |
To demonstrate that the sensor can steadily measure viscosity changes in liquids, especially in the wide pH range of various liquid systems, we explored its operation within a certain pH range. The effect of pH on the fluorescence intensity was negligible with this range of 3.0–12.0, as shown in Fig. S8 (ESI†). These results indicate that the sensor DTPMP can monitor changes in viscosity without the influence of the pH value. Afterwards, the photostability of sensor DTPMP under continuous irradiation for 60 minutes in common liquids (including commercial beverages) was determined as well. As shown in Fig. S9 (ESI†), negligible differences in the fluorescence signal in each liquid were observed. The universality in various commercial liquids can be found. Taken all together, the sensor DTPMP showed excellent adaptability, selectivity, and photostability in a complex liquid system.
Deterioration process tracking
Inspired by the above positive results, the molecular sensor for assessing viscosity fluctuation in liquids was investigated, since fresh liquids are perishable during long-term storage and transportation.30 Two kinds of commercial liquids, named lime juice and grape juice, were selected. These liquids were stored at ambient temperature (25 °C) and lower temperature (6 °C) for around 8 days, respectively. As shown in Fig. 5a, it can be found that both liquids displayed a clear and transparent appearance in the first couple of days. After 5 days of storage, floating objects were observed in the lime juice, and a yellower appearance was found in the grape juice. By contrast, when the liquids were stored under lower temperatures, the liquids remained relatively clear and displayed a normal appearance even after day 5, as shown in Fig. 5b. This deterioration process was analyzed by fluorescence spectroscopy. The fluorescence intensities of lime juice and grape juice increased obviously when these liquids were stored at ambient temperature, and the increase range was found to be 13.8% and 14.9%, respectively. However, the increase range of fluorescence intensities was only 6.8% and 8.8% when the storage environment was at fresh-maintenance temperature. This phenomenon indicated that lower temperatures can extend the storage time, and the deterioration progress can be decelerated. The abovementioned further confirmed that the molecular sensor DTPMP can monitor the viscosity fluctuations during the deterioration process.
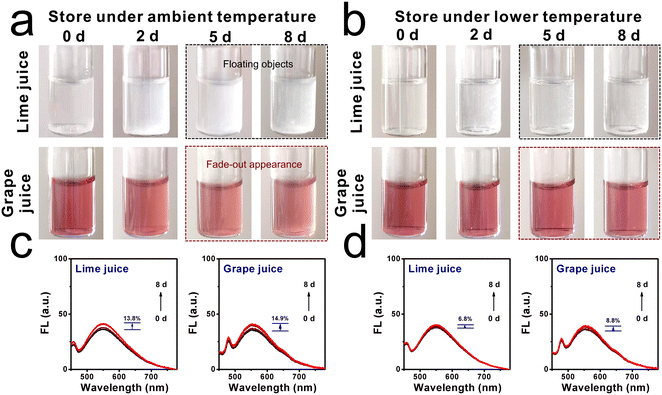 |
| Fig. 5 Digital images of the lime juice and grape juice stored at (a) ambient temperature (25 °C) and (b) lower temperature (5 °C) for varying time (from 0 to 8 days) and the corresponding fluorescence spectra of (c) lime juice and (d) grape juice at different time intervals. Concentration of DTPMP = 10 μM, λex = 420 nm. | |
Moreover, the viscosities of each liquid were determined using a viscometer from a quantitative perspective. As displayed in Fig. 6a and b, the corresponding viscosities of lime juice and grape juice increased by 22.7% and 18.3%, respectively. Meanwhile, the viscosities increased only 14.2% and 8.6% when stored under lower temperatures. The results were consistent with the fluorescence investigation results. Notably, a fitting linear relationship can be established between the viscosity increment percentage and percentage (ηn − η0)/η0 × 100% and the fluorescence intensity increment percentage (Fn − F0)/F0 × 100%, as shown in Fig. 6c. The results showed that sensor DTPMP can precisely evaluate the viscosity level in the liquid deterioration process through the fluorescence technique.
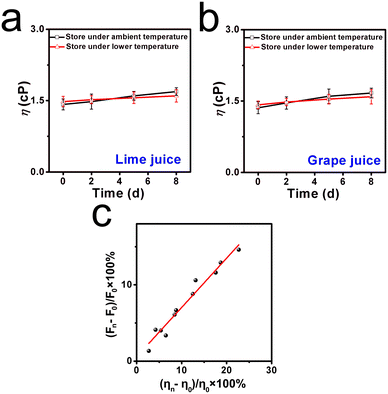 |
| Fig. 6 Viscosity values of the (a) lime juice and (b) grape juice, which were stored at room temperature or lower temperature within 8 days. (c) Fitting linear relationship among the fluorescence increment percentage and viscosity enhanced degree. | |
Conclusions
In summary, herein, we report one kind of salicylaldehyde-based molecular sensor DTPMP using a one-step facile preparation way. This sensor was constructed from a salicylaldehyde derivate and carbon trifluoride phenyl, identified as a donor–π–acceptor (D–π–A) structure, and a typical TICT mechanism was found. From the results of the experiments, DTPMP displayed an obvious turn-on response and a large Stokes shift (about 142 nm), together with the enhancement of viscosity. Moreover, the fluorescence signal of DTPMP was enhanced 97-fold from pure water to 99% glycerol. And the viscosity fluctuations caused by the addition of thickeners can be evaluated by this sensor through the fluorescence method. Moreover, spectroscopic data manifested that the sensor DTPMP possessed high photostability, selectivity, and sensitivity in various media, and its universality in complex microenvironments was confirmed. Prominently, DTPMP can measure the viscosity variations in non-invasive, in situ, and visualized procedures, and a linear relationship was found between the fluorescence increment percentage and viscosity enhanced degree. We believed that our study can effectively intersect molecular science toward liquid safety inspection, which may facilitate the perfection of functional molecular platforms.
Author contributions
Lingfeng Xu: conceptualization, writing – original draft, methodology, and funding acquisition. Yuefeng Wang & Chuan Jiang: formal analysis and investigation. Yanrong Huang: validation and visualization. Genhe He: resources and software. Limin Liu: writing – review & editing.
Conflicts of interest
There are no conflicts to declare.
Acknowledgements
This study was supported by the Natural Science Foundation of Jiangxi Province (20212BAB214031), the Doctoral Research Foundation of Jinggangshan University (JZB2006), the Innovation and Entrepreneurship Training Program for College Students of Jiangxi Province (202210419011), and the Science and Technology Program of Jiangxi Provincial Education Bureau (GJJ211032), Innovation and Entrepreneurship Training Program for College Students of Jinggangshan University (JDX2022150).
References
- X. Luo, Y. Han, X. Chen, W. Tang, T. Yue and Z. Li, Trends Food Sci. Technol., 2020, 95, 149–161 CrossRef CAS.
- Y. Han, W. Yang, X. Luo, X. He, H. Zhao, W. Tang, T. Yue and Z. Li, Crit. Rev. Food Sci. Nutr., 2022, 62, 244–260 CrossRef CAS.
- Z. Yang, Y. He, J.-H. Lee, N. Park, M. Suh, W.-S. Chae, J. Cao, X. Peng, H. Jung, C. Kang and J. S. Kim, J. Am. Chem. Soc., 2013, 135, 9181–9185 CrossRef CAS PubMed.
- Y. Baek, S. J. Park, X. Zhou, G. Kim, H. M. Kim and J. Yoon, Biosens. Bioelectron., 2016, 86, 885–891 CrossRef CAS PubMed.
- Z. Wang, Y. Zhang, Y. Liang, M. Li, Z. Meng, Y. Yang, X. Xu and S. Wang, J. Agric. Food Chem., 2022, 70, 669–679 CrossRef CAS.
- P. Arora, A. Sindhu, N. Dilbaghi and A. Chaudhury, Biosens. Bioelectron., 2011, 28, 1–12 CrossRef CAS PubMed.
- E. Lee, B. Kim and S. Choi, Sens. Actuators, A, 2020, 313, 112176 CrossRef CAS.
- J. Nsor-Atindana, H. Douglas Goff, W. Liu, M. Chen and F. Zhong, Carbohydr. Polym., 2018, 200, 436–445 CrossRef CAS.
- F. Morreale, R. Garzón and C. M. Rosell, Food Hydrocolloids, 2018, 77, 629–635 CrossRef CAS.
- X. Yang, D. Zhang, Y. Ye and Y. Zhao, Coord. Chem. Rev., 2022, 453, 214336 CrossRef CAS.
- C. Ma, W. Sun, L. Xu, Y. Qian, J. Dai, G. Zhong, Y. Hou, J. Liu and B. Shen, J. Mater. Chem. B, 2020, 8, 9642–9651 RSC.
- N. Duan, S. Yang, H. Tian and B. Sun, Food Chem., 2021, 358, 129839 CrossRef CAS PubMed.
- J. Hou, J. Du, Y. Hou, P. Shi, Y. Liu, Y. Duan and T. Han, Spectrochim. Acta, Part A, 2018, 205, 1–11 CrossRef CAS PubMed.
- Z. Zou, Q. Yan, S. Ai, P. Qi, H. Yang, Y. Zhang, Z. Qing, L. Zhang, F. Feng and R. Yang, Anal. Chem., 2019, 91, 8574–8581 CrossRef CAS PubMed.
- Y. Sun, X. Liang, J. Fan and X. Yang, J. Photochem. Photobiol., A, 2013, 253, 81–87 CrossRef CAS.
- A. Pal, M. Karmakar, S. R. Bhatta and A. Thakur, Coord. Chem. Rev., 2021, 448, 214167 CrossRef CAS.
- J. Cui, H. Nie, S. Zang, S. Su, M. Gao, J. Jing and X. Zhang, Sens. Actuators, B, 2021, 331, 129432 CrossRef CAS.
- S. Li, P. Wang, W. Feng, Y. Xiang, K. Dou and Z. Liu, Chem. Commun., 2020, 56, 1050–1053 RSC.
- F. Liu, Z. Yuan, X. Sui, C. Wang, M. Xu, W. Li and Y. Chen, Chem. Commun., 2020, 56, 8301–8304 RSC.
- I. E. Steinmark, P.-H. Chung, R. M. Ziolek, B. Cornell, P. Smith, J. A. Levitt, C. Tregidgo, C. Molteni, G. Yahioglu, C. D. Lorenz and K. Suhling, Small, 2020, 16, 1907139 CrossRef CAS.
- S. Chen, S. Zheng, S. Jiang, H. Guo and F. Yang, Anal. Bioanal. Chem., 2021, 413, 1955–1966 CrossRef CAS PubMed.
- J. Yin, X. Kong and W. Lin, Anal. Chem., 2021, 93, 2072–2081 CrossRef CAS PubMed.
- Z. Song, D. Mao, S. H. P. Sung, R. T. K. Kwok, J. W. Y. Lam, D. Kong, D. Ding and B. Z. Tang, Adv. Mater., 2016, 28, 7249–7256 CrossRef CAS.
- J. E. Kwon and S. Y. Park, Adv. Mater., 2011, 23, 3615–3642 CrossRef CAS.
- M. Koenig, G. Bottari, G. Brancato, V. Barone, D. M. Guldi and T. Torres, Chem. Sci., 2013, 4, 2502–2511 RSC.
- A. Vyšniauskas, D. Ding, M. Qurashi, I. Boczarow, M. Balaz, H. L. Anderson and M. K. Kuimova, Chemistry, 2017, 23, 11001–11010 CrossRef PubMed.
- A. Moret-Tatay, J. Rodríguez-García, E. Martí-Bonmatí, I. Hernando and M. J. Hernández, Food Hydrocolloids, 2015, 51, 318–326 CrossRef CAS.
- R. Guo, J. Yin, Y. Ma, Q. Wang and W. Lin, J. Mater. Chem. B, 2018, 6, 2894–2900 RSC.
- T. King, M. Cole, J. M. Farber, G. Eisenbrand, D. Zabaras, E. M. Fox and J. P. Hill, Trends Food Sci. Technol., 2017, 68, 160–175 CrossRef CAS.
- L. Fan, Y. Pan, W. Li, Y. Xu, Y. Duan, R. Li, Y. Lv, H. Chen and Z. Yuan, Anal. Chim. Acta, 2021, 1149, 338203 CrossRef CAS.
|
This journal is © The Royal Society of Chemistry 2023 |
Click here to see how this site uses Cookies. View our privacy policy here.