DOI:
10.1039/D3SD00109A
(Paper)
Sens. Diagn., 2023,
2, 1267-1276
A selective chemosensor via click chemistry for Cu2+ and Hg2+ ions in organic media†
Received
8th May 2023
, Accepted 30th June 2023
First published on 5th July 2023
Abstract
A simple chemosensor, 4-amino antipyrine linked bis-1,2,3-triazole, was synthesized via the “click” approach using the CuAAC method and characterized by FTIR, NMR, and ESI-MS spectroscopy. The synthesized chemosensor upon ion sensing study showed a selective and significant detection of Hg(II) and Cu(II) ions, with the LOD being 56 and 63 μM, respectively. Mercury and copper are toxic heavy metals. The quantitative and qualitative detection of these ions is paramount for human health and the environment. The effect of time and temperature on the binding capability of APT with Hg(II) and Cu(II) ions was also studied using UV-Vis spectroscopy. Additionally, DFT studies lay forward the structural optimization of the chemosensor by the (B3LYP)/6-311G(d,p) and B3LYP/LanL2DZ level of theory.
1. Introduction
In recent years, heavy metal exposure has increased due to anthropogenic and agricultural activity and modern industrialization, which negatively impact human health. Worldwide, millions of people are intoxicated by toxic metal contamination in water, air, and food, which are a matter of grave concern for humankind and the complete ecosystem of the environment. Among them, mercury and copper are toxic heavy metals that can cause severe health problems in humans and wildlife. Copper in surface waters occurs predominantly in Cu(II) cupric form. Mercury is a naturally occurring element found in air, water, and soil in various forms: elemental (or metallic) and inorganic (to which people may be exposed through their occupation), and organic (e.g., methylmercury, to which people may be exposed through their diet). Exposure to mercury, even in small amounts, may cause serious health problems. The toxicity and potential of mercury may bio-accumulate and be biomagnified in the food chain, leading to severe health problems such as neurological disorders, renal failure, and congenital disabilities.1 The permissible limit of Hg and Cu in human physiology according to the World Health Organisation (WHO) and Indian Standard Institutions (ISI) is (0.001 mg l−1 and 0.001 mg l−1) and (1.0 mg l−1 and 0.05 mg l−1), respectively.2 Beyond this limit, the aforementioned harmful effects may appear. Therefore, quantitative and qualitative detection of essential trace elements is vital to balance the smooth physiological functioning in biological systems. Developing reliable and sensitive sensors for detecting Hg and Cu ions is crucial.
Various analytical methods have been developed for detecting mercury and copper, including atomic absorption spectroscopy3 (AAS), inductively coupled plasma mass spectroscopy4 (ICPMS), and electrochemical sensing.5 However, these techniques require expensive instrumentation, large sample volume, and trained personnel, and are not always suitable for field applications. Therefore, there is a growing demand to develop a low-cost, portable, and reliable sensing platform for metal ion detection.
One promising approach for developing such a sensing platform is to use 1,2,3-triazole linked with a suitable fluorophore as a sensing molecule6–8 that allows researchers to develop recognition devices that have distinct advantages like low detection limit, naked eye visual changes, etc., over the other techniques mentioned above. 1,2,3-Triazole is a heterocyclic compound containing a five-membered ring with two carbon atoms and three nitrogen atoms that has been extensively used as a building block in synthetic organic chemistry. The biological importance of 1,4-disubstituted 1,2,3-triazoles includes antimicrobial,9–11 anticancer,12 antiviral activities,13etc. It has also been widely used in developing various analytes due to its unique electronic and structural properties, including its complexation ability with metal ions like mercury and copper. The synthesis of 1,2,3-triazole-based sensors involves the modification of the 1,2,3-triazole ring to introduce functional groups that can interact with metal ions.14 The improvement can be achieved through synthetic routes, including “click” chemistry,9,15–17 azide–alkyne cycloaddition,18,19 and Sonogashira coupling reactions.20–22 “Click” chemistry is a powerful tool for synthesizing 1,2,3-triazole-based sensors. In this method, azides and terminal alkynes react in the presence of a copper(I) catalyst to form 1,4-disubstituted-1,2,3-triazoles. The resulting 1,2,3-triazoles can further be functionalized with various reactive functional groups to enhance the sensing ability.
Antipyrine and its derivatives have provoked boundless interest, especially in medicine, due to the wide range of pharmacological activities and therapeutic applications, such as the antibacterial, antifungal, analgesic, antitumor, antipyretic, and anti-inflammatory activity of antipyrine.23–26 A few intriguing fluorescent sensors based on antipyrine have also been produced.27–30 Most of the effort has been concentrated on synthesis, structure characterization, and biological evaluation of antipyrine derivatives.31–35 Due to the abovementioned importance, we were interested in scheming and fabricating antipyrine-based materials with potential technological applications. Herein, we report the design and preparation of an antipyrine-based sensor (APT) that bears two triazole rings via the CuAAC pathway. Other sensor parts, except for the antipyrine core, serve as metal ion-binding sites. The sensing mechanisms and the analytical performance of the presented sensor for mercury and copper detection were also established via UV-vis spectroscopy.
2. Results and discussion
As reported, the symmetrical bis-alkyne 1 having an antipyrine ring was prepared using a propargylation reaction. The organic-azide 2 was obtained from the azidation of the chloroacetyl derivative of antipyrine. The proposed probe APT named 2,2′-((((1,5-dimethyl-3-oxo-2-phenyl-2,3-dihydro-1H-pyrazol-4-yl) azanediyl) bis(methylene)) bis(1H-1,2,3-triazole-4,1-diyl)) bis(N-(1,5-dimethyl-3-oxo-2-phenyl-2,3-dihydro-1H-pyrazol-4-yl) acetamide) was synthesized via the CuAAC “click” reaction of the terminal bis-alkyne with an azide, as shown in Scheme 1. The symmetrical bis-alkyne (1), organic azide (2), and probe APT were characterized successfully (detailed in the ESI† file).
 |
| Scheme 1 Synthesis of the 1,4-disubstituted bis-1,2,3-triazole based probe APTvia CuAAC. | |
2.1 Spectroscopic analysis
2.1.1 FTIR spectroscopy.
The FTIR spectroscopy analysis of the terminal bis-alkyne (1), organic azide (2), and resulting product, 1,2,3-triazole ring via click chemistry, showed a high level of agreement with the expected results. The alkyne (1) exhibited characteristic peaks at stretching frequencies of 3269 and 2113 cm−1, corresponding to the
C–H and C
C bonds, respectively. Similarly, the presence of the –N3 group in the organic azide (2) was confirmed by a peak at 2096 cm−1 in its IR spectra. Upon the fusion of alkyne and azide groups, resulting in the formation of the 1,2,3-triazole ring, the peaks at 3269, 2113, and 2096 cm−1 in the spectra of the product APT disappeared, indicating the formation of a new compound. Additionally, the emergence of a new peak at 3183 cm−1 confirmed the presence of –C
C–H structure in the triazole ring.
2.1.2 NMR and mass spectrometry.
Confirmation of the successful synthesis of the symmetrical bis-alkyne (1) and its 1,2,3-triazole i.e., APT was obtained via1H and 13C NMR spectra. The 1H NMR spectrum of compound 1 showed a triplet at δ = 2.23 ppm, attributed to the alkynyl protons (
C–H) of the bis-alkyne, which was absent in the spectrum of APT. However, the alkynyl proton appeared in the aromatic region of the 1H NMR spectrum of APT. Furthermore, the –CH2–N– protons of the bis-alkyne (1) appeared in the vicinity of the aromatic 1,2,3-triazole moiety in APT, initially detected by the downfield shift of the peak at δ = 3.96 ppm in the bis-alkyne (1). The peaks at δ = 72.26 ppm and δ = 80.74 ppm corresponding to the C
C bonds in the 13C NMR spectrum of the bis-alkyne (1) were absent in the 1,2,3-triazole APT, confirming the successful formation of the chemosensor APT. The confirmation was further supported by the observed molar mass at m/z = 852.38.
2.2 UV-vis analysis
For UV-vis investigations, after optimization of the solution concentration, the concentration of the APT solution was adjusted to 0.03 mM for sensing purposes. The absorption maxima of the probe were measured to be at 283 nm. Solutions of metal ions having 0.5 mM concentration of Ba(II), Zn(II), Cu(II), Hg(II), Ca(II), Mg(II), Cd(II), Pb(II), Ni(II), Co(II), Mn(II) and Cr(III) were prepared in DMSO and then evaluated for sensing experiments using the synthesized probe APT. Only the solutions of metal ions Hg(II) and Cu(II) were shown to effectively induce substantial changes in the absorption spectra as shown in Fig. 1, while the relative change in the absorption intensity is graphically represented in Fig. 2.
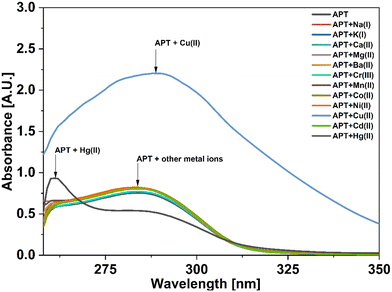 |
| Fig. 1 UV-vis absorption spectra of APT with the addition of various metal ions. | |
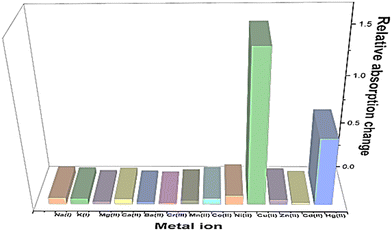 |
| Fig. 2 Relative absorption changes of APT with various metal ions in DMSO. | |
2.3 Chemo-sensing potential of APT
UV-vis spectral analysis was used to investigate the metal ion recognition potential of the probe APT with Hg(II) and Cu(II) ions. This was accomplished by titration of 0.03 mM APT solution with 15 equiv. of 0.5 mM Hg(II) and Cu(II) solution in discrete instances. The probe concentration was maintained at 0.03 mM throughout the analysis, while the concentration of metal ions was systematically raised from 0 to 15 equiv. On the incremental addition of Hg(II) ions to APT solution, the absorption intensity of the latter exhibited a progressive hypochromic shift at 283 nm accompanied by a significant hyperchromic shift leading to the emergence of a sharp peak at 261 nm, with an isosbestic point occurring at 271 nm and hence, verifying the interaction of Hg(II) with the probe APT. The absorbance maxima shift (An/Ao) vs. metal ion molar concentration for the peak at 261 nm with 15 equiv. of Hg(II) ions added in a sequential manner as shown in the inset of Fig. 3, wherein An = maximum absorbance, when Hg(II) ions were added incrementally, and Ao = maximum absorbance of the probe. Furthermore, by analyzing the correlation map (Fig. S14†), it was calculated that the LoD (limit of detection) and LoQ (limit of quantification) for Hg(II) is 56 μM and 186 μM, respectively. Also, the stoichiometric ratio between the probe and the metal ion(s) was verified by means of Job plot analysis, wherein different solutions containing APT and the metal ion were prepared by holding the total molar concentration constant while altering the molar ratio of APT (or metal ion) from 0.1 to 0.9. Fig. S16† shows the relationship between the molar concentration of the metal ions and the relative absorbance change; a threshold at the 0.7 molar ratio suggests a 2
:
1 (M
:
L) stoichiometry, which are collectively presented in Table 1.
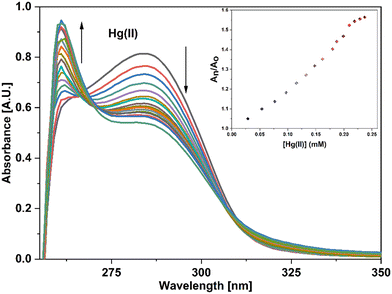 |
| Fig. 3 Progressive changes in the absorption spectrum of APT on incremental addition of 15 equiv. of Hg(II) ions; the inset shows the relationship between the relative absorbance of APT (An/Ao) and the molar concentration of the ion (mM). | |
Table 1 LoD, LoQ, and stoichiometric binding of APT with Cu(II) and Hg(II) ions
Probe |
Metal ion |
LoD (μM) |
LoQ (μM) |
Association constant (Ka) |
Stoichiometry (M : L) |
APT
|
Hg(II) |
56 |
186 |
7.34 × 103 M−1 |
2 : 1 |
APT
|
Cu(II) |
63 |
212 |
4.43 × 104 M−1 |
2 : 1 |
In the case of Cu(II) ions, a progressive addition of Cu(II) ion solution to the 0.03 mM solution of APT exhibited a hyperchromic shift at 283 nm, with a slight bathochromic shift of about 3–4 nm, thereby establishing the sensing potential of APT for Cu(II) ions also. The absorbance maxima shift (An/Ao) vs. metal ion molar concentration for the peak at 283 nm with 15 equiv. of Cu(II) ions added in a sequential manner as shown in the inset of Fig. 4, wherein An = maximum absorbance, when Cu(II) ions were added incrementally, and Ao = maximum absorbance of the probe. Furthermore, by analyzing the correlation map (Fig. S15†), it was calculated that the LoD and LoQ for Cu(II) are 63 μM and 212 μM, respectively, with a binding ratio of 2
:
1 (M
:
L), which are also collectively presented in the above mentioned Table 1.
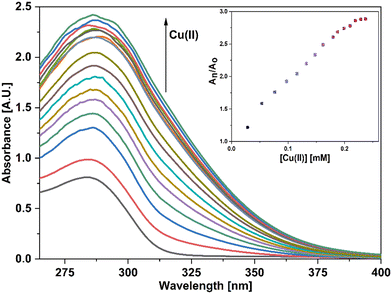 |
| Fig. 4 Progressive changes in the absorption spectrum of APT on incremental addition of 15 equiv. of Cu(II) ions; the inset shows the relationship between the relative absorbance of APT (An/Ao) and the molar concentration of the metal ion (mM). | |
Furthermore, the Benesi–Hildebrand (B–H) equation (I) was used to calculate the association constant (Ka) for the 2
:
1 metal–APT complexation with respect to Hg(II) and Cu(II) ions, and the associated B–H graphs are shown in Fig. S17 and S18.† The Ka values for the APT–metal complexation were determined to be 7.34 × 103 M−1 for Hg(II) and 4.43 × 104 M−1 for Cu(II) as presented in the above mentioned Table 1.
2.3.1 Competitive metal ion studies.
Competitive ion titration of a 0.03 mM probe solution in DMSO with a solution containing an equimolar concentration of multiple metal ions demonstrated the probe's practical usefulness to preferentially detect Cu(II) independent of the presence of other metal ions. Based on the absorption spectrum (Fig. 5) obtained after the titrations, it seemed that the sensor probe's capacity to identify Cu(II) was unaffected by the presence of other metal ions. However, some significant diminishing in the intensity of absorbance was observed. Additionally, it was also determined from the spectrum that the probe had a binding preference for Cu(II) over other metal ions.
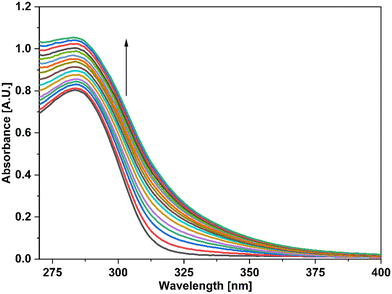 |
| Fig. 5 UV-vis spectra of the probe APT signifying the recognition of Cu(II) among various metal ions present in equimolar concentration. | |
In addition, UV-vis spectroscopy was also employed to examine the probe's propensity for preferentially sensing either Hg(II) or Cu(II) in the presence of both metal ions. The absorption spectrum obtained when the probe solution was titrated with an equimolar solution of Hg(II) and Cu(II) ions was identical to that obtained for Cu(II), as shown in Fig. 4, demonstrating the probe's stronger selectivity for Cu(II) over Hg(II) (Fig. 6).
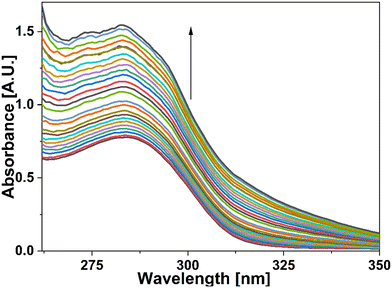 |
| Fig. 6 Absorption spectrum of APT demonstrating the selective recognition of Cu(II) over Hg(II) from an equimolar concentration solution of both metal ions. | |
2.3.2 Time-dependent analysis.
After analyzing the influence of time on the binding of APT with both metal ions, i.e., Hg(II) and Cu(II), for twenty minutes, the spectral data (Fig. S19 and 20†) revealed that the absorption intensity of the APT solution with added Hg(II) as well as Cu(II) ions was not dependent on the time at any point throughout the study. In addition, the aforementioned metal ion solutions, when added to the probe solution, displayed immediate changes in absorption, which indicated that the probe APT is an effective “no-wait” sensor for Hg(II) and Cu(II).
2.3.3 Temperature-dependent analysis.
The impact of temperature on the probe's binding efficiency was also measured across a wide range of conditions. A positive link between the absorbance of Hg(II)/Cu(II)-bound APT and temperature was established as a result of the observation that the absorption spectrum of APT displayed a mild rise in the absorption intensity on a steady increase in the temperature from 20 °C to 50 °C. Fig. S21 and S22† represent the spectra of Hg(II)-bound APT and Cu(II)-bound APT at 2 °C intervals from room temperature up to 50 °C.
3. Computational studies
Density functional theory has become an important tool for computational studies, which acts as a potential instrument for providing structural understanding, especially in organic molecules with extended conjugation, and interpreting several physiochemical properties such as the energy gap (ΔELUMO–HOMO). The stability and geometrical optimization of the synthesized probe APT were also explored using DFT calculations. The geometrically optimized structures of the alkyne (1), azide (2), and APT along with complexes such as APT.Cu and APT.Hg were obtained by the DFT basis set B3LYP/6-311G(d,p) and B3LYP/LanL2DZ level of theory using the Gaussian 09 package36,37 as illustrated in Fig. S23–S25.† Additionally, the frontier molecular orbital diagrams with an energy difference of these optimized molecules (1, 2, APT, and APT.Cu) are represented in Fig. 7. However, the frontier molecular orbital diagram with an energy difference of the APT.Hg optimized molecule is given in Fig. S27.† As clearly depicted in Fig. 7, the band-gap energy of 4-amino antipyrine based 1,2,3-triazole, i.e., APT considerably decreases to 4.34 eV as compared to its immediate predecessors, alkyne (4.60 eV) and azide (4.85 eV), which further decreased to 3.55 eV once it formed a complex with Cu, as shown in Table 2. Therefore, the order of the band-gap energy of 1, 2, APT, and APT.Cu is: 2 (4.85 eV) > 1 (4.60 eV) > APT (4.34 eV) > APT.Cu (3.55 eV). The lowest band-gap energy of the APT.Cu complex is due to the probable availability of the metal orbital for accommodating the electron as an acceptor. Surprisingly, the band-gap energy of the APT.Hg complex is further decreased to 0.28 eV due to the projection of heavy metal Hg, which can more easily accommodate the electrons to better act as an acceptor.
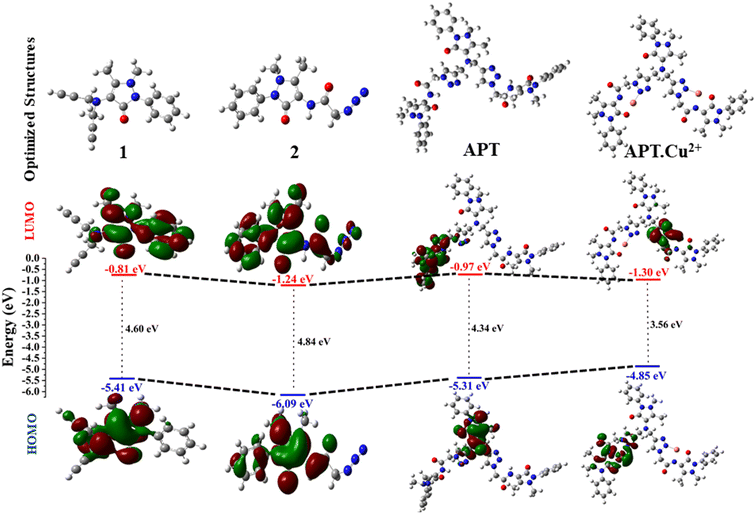 |
| Fig. 7 Optimized structures (1, 2, APT, and APT.Cu) with the (B3LYP)/6-311G (d,p) and B3LYP/LanL2DZ level of theory via the Gaussian 09 package along with their contour plots. | |
Table 2 Calculated FMOs and energy gap in eV at the B3LYP/6-311G(d,p) and B3LYP/LanL2DZ level of theory
S. no. |
Compound |
HOMO |
LUMO |
ΔELUMO–HOMO |
1. |
1
|
−5.41 |
−0.81 |
4.60 |
2. |
2
|
−6.09 |
−1.24 |
4.85 |
3. |
APT
|
−5.31 |
−0.97 |
4.34 |
4. |
APT.Cu
|
−4.85 |
−1.30 |
3.55 |
5. |
APT.Hg
|
−4.07 |
−3.79 |
0.28 |
4. Probable binding mode
The host–guest relationship38 between triazole of the probe APT with Hg(II) and Cu(II) selectively in the presence of other metal ions refers to the specific interaction and recognition between these entities, primarily based on the HSAB principle.39 According to this principle, which classifies Hg(II) and Cu(II) as weak acids,40 have a higher affinity for APT as the ligand cavity is compatible b/w these two, compared to other metal ions. The 1,2,3-triazole moiety of APT can bind to the electron-deficient metal ions via different lone pair bearing N and O atoms. Since the Job's plot of APT corresponds to a 2
:
1 stoichiometry of metal–ligand, and the 1,2,3-triazole moiety's N atoms and the ligand's O atoms can be attributed to being involved in the metal ion binding, the other valency is satisfied by the solvent molecule. Therefore, based on these instances, a proposed mode of interaction between APT and metal ions has been portrayed in Fig. 8.
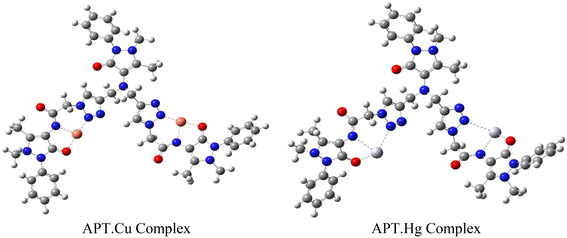 |
| Fig. 8 Proposed binding of the probe APT with the electron-deficient metal ions. | |
Overall, the host–guest relationship between the probe APT and Hg(II) and Cu(II) ions in the presence of other metal ions is based on the hard–soft acid–base principle, which provides a framework for understanding and predicting the preferential binding interactions between metal ions and ligands based on their electronic properties.
5. Experimental
5.1 Materials and methods
All chemicals were purchased from commercially available sources and used without further purification. 4-Amino antipyrine was purchased from (Loba Chemie), propargyl bromide from (Sigma Aldrich), potassium carbonate from (Loba Chemie), chloroacetyl chloride from (Loba Chemie), and sodium azide from (Loba Chemie). Chlorides of various metal ions such as Ba(II), Zn(II), Cu(II), Hg(II), Ca(II), Mg(II), Cd(II), Pb(II), Ni(II), Co(II), Mn(II) and Cr(III) were purchased from Loba Chemie. Solvents were bought from S.D. Fine, Finar, and Loba Chemie. 1H-NMR and 13C-NMR spectra were recorded using a Jeol ECZ 400S instrument with TMS (tetramethylsilane) as a standard internal reference. ESI-MS spectra were recorded using a XEVO G2-XS QTOF spectrometer. FTIR data was obtained from a Shimadzu IRAffinity-1S spectrophotometer. X-ray diffraction data was collected by a Bruker APEX-II CCD diffractometer. A SHIMADZU UV-1900 spectrometer was used for metal ion sensing studies.
5.2 Synthesis and characterization of 4-(di(prop-2-yn-1-yl) amino)-1,5- dimethyl-2-phenyl-1,2-dihydro-3H-pyrazol-3-one, 1
Bis-alkyne was prepared by following a known method.27 For synthesis, 4-amino antipyrine was taken in a round bottom flask and dissolved in 8–10 mL of DMF. K2CO3 (2.5 equiv.) was added, and the reaction mixture was stirred for 30 min. After that, propargyl bromide (2.2 equiv.) was added dropwise with constant stirring, and the reaction was allowed to go continuously for another 18 h at room temperature. The reaction progress was monitored with TLC. After completion of the reaction, the workup was facilitated in ice-cold water to get precipitates. The solid that appeared was filtered and washed with plenty of cold water to remove DMF. Further, residues were recrystallized in acetonitrile to obtain the desired product as a brown solid.
Yield: 95%, color: brown solid, m.p. 123–125 °C. FTIR (νmax cm−1): 3269, 3205, 2995, 2933, 2837, 2113, 1651, 1498, 1288 cm−1; 1H NMR (400 MHz, CDCl3) δ 7.48–7.26 (m, 5H), 3.96 (d, J = 4 Hz, 4H), 3.05 (s, 3H), 2.30 (s, 3H), 2.23 (t, J = 4 Hz, 2H); 13C NMR (101 MHz, CDCl3) δ 163.40 (C), 153.83 (C), 135.14 (C), 129.19 (CH), 126.45 (CH), 123.69 (CH), 118.69 (C), 80.74 (C), 77.16 (CH), 72.26 (CH), 42.84 (CH2), 36.44 (CH3), 10.83 (CH3).
5.3 Synthesis and characterization of 2-azido-N-(1,5-dimethyl-3-oxo-2-phenyl-2,3-dihydro-1H-pyrazol-4-yl)acetamide, 2
First, the chloroacetyl chloride derivative of 4-amino antipyrine was synthesized by following the reported procedure41 with some modifications. 1 mmol of 4-amino antipyrine was dissolved in 10 mL DCM in a 100 mL round-bottom flask; 1.5 equiv. of K2CO3 was added, and the reaction mixture was allowed to stir in an ice bath. A solution of chloroacetyl chloride (1.2 equiv.) was added dropwise to the reaction mixture, and after addition, the ice bath was removed, and the reaction mixture was allowed to reflux for 10–12 h. On completion of the reaction, as monitored by TLC, the workup was done by filtration followed by evaporation of the filtrate on a rotary evaporator. The solid crude product was washed with plenty of cold water and, after complete drying, used as such for the azidation reaction. The so-obtained solid was dissolved in a minimum amount of acetonitrile. Then, 1.5 equiv. of sodium azide was added to this reaction mixture. On completion of the reaction, the mixture was filtered, and then the filtrate was evaporated on a rotary evaporator. The solid that appeared was washed with cold water and dried under a vacuum to obtain a pale yellow solid.
Yield: 90%, color: pale yellow solid, m.p. 158–160 °C. FTIR (νmax cm−1): 3238, 3207, 3024, 2958, 2096, 1693, 1490, 1307 cm−1. 1H NMR (400 MHz, CDCl3) δ 9.36 (s, H), 7.32–7.49 (m, 5H), 3.88 (s, 2H), 3.11 (s, 3H), 2.22 (s, 3H); 13C NMR (101 MHz, CDCl3) δ 167.42 (C), 162.13 (C), 150.51 (C), 134.53 (C), 129.86 (CH), 128.07 (CH), 125.35 (CH), 107.72 (C), 52.36 (CH2), 36.09 (CH3), 12.61 (CH3). ESI-MS [M + H]+: m/z cal. for C43H45N15O5H+ is 287.125, found 287.147. Finally, the solid crystalline material was developed into the single crystal of compound 2 (CCDC no. 2254070), as shown in Fig. 9, upon recrystallization with the solution of ethanol and n-hexane. The complete details of the crystal and its structure refinement are given in Table 3. However, the bond length and angles of the obtained single crystal of compound 2 are detailed in Tables 4 and 5, respectively.
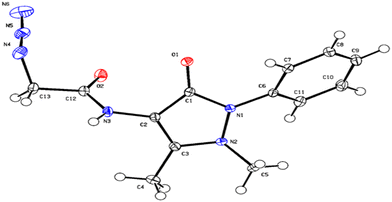 |
| Fig. 9 ORTEP diagram of compound 2 (CCDC no. 2254070). | |
Table 3 Crystal data and structure refinement for compound 2
Compound |
2
|
Identification code |
mo_SB_0m_a |
Molecular structure |
|
Crystal structure (CCDC: 2254070) |
|
Crystal image |
|
Empirical formula |
|
Formula weight |
286.30 |
Temperature/K |
100(2) |
Crystal system |
‘Monoclinic’ |
Space group |
‘P21/n’ |
a/Å |
13.3072(7) |
b/Å |
6.9369(3) |
c/Å |
15.2738(9) |
α/° |
90 |
β/° |
106.507(2) |
γ/° |
90 |
Volume/Å3 |
1351.82(12) |
Z
|
4 |
ρ
calc g cm−3 |
1.407 |
μ/mm−1 |
0.101 |
Radiation |
MoKα (λ = 0.71073) |
2Θ range for data collection/° |
4.794 to 68.222 |
Reflections collected |
92 555 |
Independent reflections |
5503 [Rint = 0.0871, Rsigma = 0.0458] |
Data/restraints/parameters |
5503/0/192 |
Final R indexes [I ≥ 2σ(I)] |
R
1 = 0.0447, wR2 = 0.1027 |
Final R indexes [all data] |
R
1 = 0.0751, wR2 = 0.1143 |
Largest diff. peak/hole/e Å−3 |
0.41/−0.32 |
Table 4 Bond lengths (Å) of compound 2
Atom 1 |
Atom 2 |
Length/Å |
Atom 1 |
Atom 2 |
Length/Å |
O1 |
C1 |
1.2404(11) |
N5 |
N6 |
1.1327(14) |
O2 |
C12 |
1.2248(11) |
C6 |
C7 |
1.3937(13) |
N3 |
C2 |
1.4113(11) |
C6 |
C11 |
1.3957(13) |
N3 |
C12 |
1.3510(12) |
C2 |
C1 |
1.4391(13) |
N1 |
N2 |
1.4065(11) |
C2 |
C3 |
1.3576(13) |
N1 |
C6 |
1.4153(11) |
C7 |
C8 |
1.3910(13) |
N1 |
C1 |
1.3851(11) |
C11 |
C10 |
1.3914(14) |
N2 |
C3 |
1.3854(11) |
C12 |
C13 |
1.5211(13) |
N2 |
C5 |
1.4730(12) |
C3 |
C4 |
1.4883(13) |
N4 |
N5 |
1.2349(13) |
C8 |
C9 |
1.3906(15) |
N4 |
C13 |
1.4721(14) |
C10 |
C9 |
1.3886(15) |
Table 5 Bond angles (°) of compound 2
Atom 1 |
Atom 2 |
Atom 3 |
Angle/° |
Atom 1 |
Atom 2 |
Atom 3 |
Angle/° |
C12 |
N3 |
C2 |
120.88(8) |
C8 |
C7 |
C6 |
119.33(9) |
N2 |
N1 |
C6 |
121.10(7) |
O1 |
C1 |
N1 |
124.01(8) |
C1 |
N1 |
N2 |
110.55(7) |
O1 |
C1 |
C2 |
131.40(8) |
C1 |
N1 |
C6 |
126.34(8) |
N1 |
C1 |
C2 |
104.55(8) |
N1 |
N2 |
C5 |
115.33(7) |
C10 |
C11 |
C6 |
119.75(9) |
C3 |
N2 |
N1 |
106.01(7) |
O2 |
C12 |
N3 |
124.18(8) |
C3 |
N2 |
C5 |
120.45(8) |
O2 |
C12 |
C13 |
121.03(8) |
N5 |
N4 |
C13 |
117.18(8) |
N3 |
C12 |
C13 |
114.79(8) |
N6 |
N5 |
N4 |
169.59(11) |
N2 |
C3 |
C4 |
121.63(8) |
C7 |
C6 |
N1 |
118.96(8) |
C2 |
C3 |
N2 |
109.69(8) |
C7 |
C6 |
C11 |
120.42(8) |
C2 |
C3 |
C4 |
128.68(8) |
C11 |
C6 |
N1 |
120.61(8) |
C9 |
C8 |
C7 |
120.36(9) |
N3 |
C2 |
C1 |
124.36(8) |
C9 |
C10 |
C11 |
119.91(9) |
C3 |
C2 |
N3 |
126.64(8) |
N4 |
C13 |
C12 |
110.70(8) |
C3 |
C2 |
C1 |
109.00(8) |
C10 |
C9 |
C8 |
120.19(9) |
5.4 Synthesis and characterization of the probe, APT
The synthesis of the 1,2,3-triazole derivative was performed by following the standard reported procedure. 1 mmol of alkyne 1 was dissolved in 8 mL EtOH in a 50 mL round bottom flask. To this, 2.2 mmol of organic azide 2 was added by dissolving in a minimum amount of EtOH. To the above reaction mixture, copper-sulfate and sodium-ascorbate in 10 and 20 mol%, respectively were added by dissolving each in 1 mL water. The reaction was completed in less than 30 minutes as confirmed by TLC monitoring. After that, the reaction was quenched with ice-cold water to obtain an off-white solid which was washed with 50–100 mL cold water and dried under a vacuum.
Yield: 89%, color: off-white solid, m.p. 240–241 °C. FTIR (νmax cm−1): 3265, 3188, 3030, 2922, 2854, 1701, 1309, 759 cm−1. 1H NMR (400 MHz, CDCl3) δ 9.63 (s, 2H), 7.92 (s, 2H), 7.27–7.50 (m, 15H), 5.28 (s, 4H), 4.27 (s, 4H), 3.04 (s, 6H), 2.80 (s, 3H), 2.11 (s, 6H), 1.75 (s, 3H); 13C NMR (101 MHz, CDCl3) δ 165.06 (C), 161.41 (C), 151.94 (C), 134.88 (C), 129.13 (C), 128.91 (CH), 126.39 (CH), 125.76 (CH), 125.15 (CH), 123.64 (CH), 123.18 (C), 106.57 (C), 51.43 (CH2), 48.27 (CH2), 35.90 (CH3), 11.23 (CH3), 9.75 (CH3). ESI-MS [M + H]+: m/z cal. for C43H45N15O5H+ is 852.3802, found 852.3802.
5.5 Spectrophotometric analysis
The synthesized probe APT was analyzed for sensing behavior using UV-visible spectroscopy. Metal ion selectivity was determined with various metal ions such as Ba(II), Zn(II), Cu(II), Hg(II), Ca(II), Mg(II), Cd(II), Pb(II), Ni(II), Co(II), Mn(II) and Cr(III). The sensitivity of the probe APT towards Cu(II) and Hg(II) ions was also examined through absorption spectroscopy. Time and temperature-dependent studies, along with competing metal ion titrations were performed for the synthesized probe. The association constant Ka was determined from the B–H plot with the formula Ka = 1/slope, where the slope is determined by plotting 1/conc. of analyte vs. 1/change in absorbance. The limit of detection (LoD) was calculated as 3σ/k, and the limit of quantification (LoQ) as 10σ/k, where the standard deviation of the maximum intensity of the probe is denoted by σ. k is the slope of the maximum absorption wavelength intensity as a function of ion concentration.8
Conclusion
Heavy metals are essential to life in small amounts, but excessive exposure can be toxic. Mercury and copper are two heavy metals that can harm the brain, heart, lungs, kidneys, and immune system when their levels exceed the body's tolerance. Children are particularly vulnerable to mercury exposure as it can impair their neurological development. It's important to be aware of the sources of heavy metals and take steps to minimize exposure. As a result, new affordable methods for detecting such ions are thus required during the time. Considering this, a new chemosensor was synthesized from 4-aminoantipyrine with a “quick-click” approach for the selective detection of Hg(II) and Cu(II) ions with an association constant of 7.34 × 103 M−1 and 4.43 × 104 M−1, respectively. This probe exhibited significant changes in its absorption spectra upon the addition of metal ions, allowing for the detection of these metal ions. Additionally, DFT studies were conducted to understand the spatial arrangement of various groups present in the probe through an energy-minimized structure. In order to enhance the capacity of the chemosensor APT and derivatives to recognize metal ions in real-world samples, we are currently developing additional testing techniques for upcoming investigations.
Author contributions
Sachin Kumar: methodology, investigation, validation, writing manuscript. Bajrang Lal: methodology, validation. Ram Kumar Tittal: conceptualization, methodology, resources, supervision, writing: review and editing. Gurleen Singh: investigation, validation. Jandeep Singh: conceptualization, resources, writing: review and editing. Vikas D. Ghule: investigation, validation. Renu Sharma: supervision. Jagjivan K. Sabane: investigation, validation.
Conflicts of interest
The authors declare no conflicts of interest.
Acknowledgements
One of the authors, BL acknowledge CSIR-JRF (No.09/1050(0014)/2019-EMR-I) for fellowship and financial assistance. The authors express their gratitude to the National Institute of Technology, Kurukshetra and Lovely Professional University for providing the support and resources that allowed them to complete the present research work.
References
- M. Balali-Mood, K. Naseri, Z. Tahergorabi, M. R. Khazdair and M. Sadeghi, Front. Pharmacol., 2021, 12, 1–19 CrossRef PubMed.
- M. Kumar and A. Puri, Indian J. Occup. Environ. Med., 2012, 16, 40–44 CrossRef.
- J. W. Robinson, Anal. Chem., 1960, 32, 17A–29A CrossRef CAS.
- S. C. Wilschefski and M. R. Baxter, Clin. Biochem. Rev., 2019, 40, 115–133 Search PubMed.
- J. Baranwal, B. Barse, G. Gatto, G. Broncova and A. Kumar, Chemosensors, 2022, 10, 363 CrossRef CAS.
- M. Loya, N. Pramanik, P. Pahari and A. K. Atta, J. Photochem. Photobiol., A, 2022, 433, 114173 CrossRef CAS.
- A. K. Atta, S. I. Hazarika, M. Loya and S. Giri, J. Photochem. Photobiol., A, 2022, 425, 113723 CrossRef CAS.
- S. Kumar, S. Gadiyaram, R. K. Tittal, V. D. Ghule and R. Sharma, J. Mol. Struct., 2023, 1290, 135940 CrossRef CAS.
- N. Nehra, R. K. Tittal, D. Ghule Vikas, Naveen and K. Lal, J. Mol. Struct., 2021, 1245, 131013 CrossRef CAS.
- Naveen, R. K. Tittal, V. D. Ghule, P. Yadav, K. Lal and A. Kumar, Steroids, 2020, 161, 108675 CrossRef CAS.
- N. Nehra, R. K. Tittal and V. D. Ghule, ACS Omega, 2021, 6, 27089–27100 CrossRef CAS PubMed.
- P. Yadav, K. Lal, A. Kumar, S. K. Guru, S. Jaglan and S. Bhushan, Eur. J. Med. Chem., 2017, 126, 944–953 CrossRef CAS PubMed.
- Y.-W. He, C.-Z. Dong, J.-Y. Zhao, L.-L. Ma, Y.-H. Li and H. A. Aisa, Eur. J. Med. Chem., 2014, 76, 245–255 CrossRef CAS.
- P. Kaur, B. Lal, N. Kaur, G. Singh, A. Singh, G. Kaur and J. Singh, J. Photochem. Photobiol., A, 2019, 382, 111847 CrossRef CAS.
- G. Singh, R. Singh, N. George, G. Singh, P. Satija, G. Kaur, H. Singh and J. Singh, J. Mol. Struct., 2023, 1277, 134823 CrossRef CAS.
- K. Sharma, R. K. Tittal, K. Lal, R. S. Mathpati and V. D. Ghule, New J. Chem., 2023, 47, 9077 RSC.
- P. Saini, Sushma, G. Singh, G. Kaur, J. Singh and H. Singh, Inorg. Chem. Commun., 2022, 141, 109524 CrossRef CAS.
- J. Sultana and D. Sarma, Catal. Rev.: Sci. Eng., 2020, 62, 96–117 CrossRef CAS.
- J. R. Johansson, T. Beke-Somfai, A. Said Stålsmeden and N. Kann, Chem. Rev., 2016, 116, 14726–14768 CrossRef CAS PubMed.
- B. Godlewski, D. Baran, M. de Robichon, A. Ferry, S. Ostrowski and M. Malinowski, Org. Chem. Front., 2022, 9, 2396–2404 RSC.
- E. M. da Silva, D. Y. de Albuquerque, J. Zukerman-Schpector and R. S. Schwab, Asian J. Org. Chem., 2021, 10, 1153–1160 CrossRef CAS.
- I. Kanwal, A. Mujahid, N. Rasool, K. Rizwan, A. Malik, G. Ahmad, S. A. A. Shah, U. Rashid and N. M. Nasir, Catalysts, 2020, 10, 443 CrossRef CAS.
- M. Hartleb, Biopharm. Drug Dispos., 1991, 12, 559–570 CrossRef CAS PubMed.
- M. Adithya Krishnan, S. Saranyaparvathi, C. Raksha, B. Vrinda, C. G. Girish, N. V. Kulkarni and B. I. Kharisov, Russ. J. Coord. Chem., 2022, 48, 696–724 CrossRef CAS.
- R. Çakmak, E. Başaran, M. Boğa, Ö. Erdoğan, E. Çınar and Ö. Çevik, Russ. J. Bioorg. Chem., 2022, 48, 334–344 CrossRef.
- C. J. Dhanaraj and S. S. Salin Raj, Inorg. Chem. Commun., 2020, 119, 108087 CrossRef CAS.
- G. Singh, Suman, Diksha, Pawan, Mohit, Sushma, Priyanka, A. Saini and P. Satija, J. Mol. Struct., 2022, 1250, 131766 CrossRef CAS.
- G. T. Selvan, M. Kumaresan, R. Sivaraj, I. V. M. V. Enoch and P. M. Selvakumar, Sens. Actuators, B, 2016, 229, 181–189 CrossRef CAS.
- V. K. Gupta, A. K. Singh and N. Mergu, Electrochim. Acta, 2014, 117, 405–412 CrossRef CAS.
- J. Dessingou, K. Tabbasum, A. Mitra, V. K. Hinge and C. P. Rao, J. Org. Chem., 2012, 77, 1406–1413 CrossRef CAS PubMed.
- D. Premnath, P. Mosae Selvakumar, P. Ravichandiran, G. Tamil Selvan, M. Indiraleka and J. Jannet Vennila, Spectrochim. Acta, Part A, 2016, 153, 118–123 CrossRef CAS PubMed.
- P. M. Selvakumar, E. Suresh and P. S. Subramanian, Polyhedron, 2007, 26, 749–756 CrossRef CAS.
- N. Uramaru, H. Shigematsu, A. Toda, R. Eyanagi, S. Kitamura and S. Ohta, J. Med. Chem., 2010, 53, 8727–8733 CrossRef CAS PubMed.
- R. Teran, R. Guevara, J. Mora, L. Dobronski, O. Barreiro-Costa, T. Beske, J. Pérez-Barrera, R. Araya-Maturana, P. Rojas-Silva, A. Poveda and J. Heredia-Moya, Molecules, 2019, 24, 1–21 CrossRef PubMed.
- H. M. Y. Al-Labban, H. Mohammed Sadiq and A. A. J. Aljanaby, J. Phys.: Conf. Ser., 2019, 1294, 052007 CrossRef CAS.
-
M. J. Frisch, G. Trucks, H. B. Schlegel, G. E. Scuseria, M. A. Robb, J. R. Cheeseman, G. Scalmani, V. Barone, B. Mennucci, G. A. Petersson, H. Nakatsuji, M. Caricato, X. Li, H. P. Hratchian, A. F. Izmaylov, J. Bloino, G. Zheng, J. L. Sonnenberg, M. Hada, M. Ehara and K. Toyota, Gaussian 09, Revision A.02, Gaussian, Inc., Wallingford, CT, 2009, 227–238 Search PubMed.
- G. Singh, R. Singh, N. George, G. Singh, Sushma, G. Kaur, G. Kaur, H. Singh and J. Singh, J. Photochem. Photobiol., A, 2023, 441, 114741 CrossRef CAS.
- F. Ahmed and H. Xiong, Dyes Pigm., 2021, 185, 108905 CrossRef CAS.
- R. G. Pearson, J. Am. Chem. Soc., 1963, 85, 3533–3539 CrossRef CAS.
- R. G. Pearson, J. Am. Chem. Soc., 1963, 85, 3533–3539 CrossRef CAS.
- S. Murtaza, A. A. Altaf, M. Hamayun, K. Iftikhar, M. N. Tahir, J. Tariq and K. Faiz, Eur. J. Chem., 2019, 10, 358–366 CrossRef CAS.
|
This journal is © The Royal Society of Chemistry 2023 |
Click here to see how this site uses Cookies. View our privacy policy here.