Photoinduced micropatterning on biodegradable aliphatic polyester surfaces for anchoring dual brushes and its application in bacteria and cell patterning†
Received
12th July 2022
, Accepted 27th September 2022
First published on 27th September 2022
Abstract
In view of intrinsic challenges encountered in surface patterning on actual biomaterials such as the ones based on biodegradable polymers, we have demonstrated an innovative strategy to create micro-patterns on the surface of tartaric acid based aliphatic polyester P (poly(hexamethylene 2,3-O-isoprpylidentartarate)) without significant loss of its molecular weight. Around 10 wt% PAG (photoacid generator, 2-(4-methoxystyryl)-4,6-bis(trichloromethyl)-1,3,5-triazine) was purposefully encapsulated in a polyester matrix comprising of P and PLA (polylactide) at a ratio of 5
:
95. With the help of a photomask, selective areas of the matrix were exposed to UV radiation at 395 nm for 25 min to trigger the acid release from PAG entrapped unmasked areas for generating hydroxyl functionality that was later converted to an ATRP (atom transfer radical polymerization) initiating moiety on the irradiated domain of P. In subsequent steps, spatio-selective surface modification by surface initiated ATRP was carried out to generate an alternate pattern of polyPEGMA (poly(ethylene glycol)methyl ether methacrylate) and polyDMAPS (poly(3-dimethyl-(methacryloyloxyethyl)ammonium propane sulfonate)) brushes on the matrix. The patterned surface modified with dual brushes was found to be antifouling in nature (rejection of >97% of proteins). Strikingly, an alternate pattern of live bacterial cells (E. coli and S. aureus) was evident and a relatively high population of bacteria was found on the polyPEGMA brush modified domain. However, a complete reverse pattern was visible in the case of L929 mouse fibroblast cells, i.e., cells were found to predominantly adhere to and proliferate on the zwitterionic brush modified surface. An attempt was made to discuss a plausible mechanism of selective cell adhesion on the zwitterionic brush domain. This novel strategy employed on the biodegradable polymer surface provides an easy and straightforward way to micro-pattern various cells, bacteria, etc. on biodegradable substrates which hold great potential to function as biochips, diagnostics, bacteria/cell microarrays, etc.
Introduction
During the last decade, there has been increasing attention towards immobilization of biological macromolecules such as proteins, bacteria and cells onto patterned surfaces to develop protein/bacteria/cell microarrays.1–3 Spatio-selective interactions between the functionalized surface and biomolecules determine facilitate conjugation, adhesion, proliferation, inhibition, death and other responses.4–6 For example, cell adhesion on designated places of micropatterned surfaces can be employed in several biomedical applications such as tissue engineering, biomedical implantation, cell based diagnostics, etc.7,8 On the other hand, bacterial microarrays9,10 can be a promising platform to function as diagnostics for various diseases, as a tracker for genotoxins or heavy metals, and for gene expression assays including fundamental studies of biological processes.9–12 Various techniques such as soft lithography, microcontact printing using patterned stamps, laser ablation, photopolymerization through a mask, etc. are available in the literature to immobilize biomacromolecules in a precise manner on patterned surfaces.3,9,13 Recently, immobilization of biomolecules on polymer brush modified patterned surfaces has drawn significant attention due to their versatility in topography and functionality.4,14–17 Moreover, owing to the high density of functional motifs as compared to self-assembled monolayers, polymer brush modified surfaces with tailored chemical functionality and topographic features may attract/repel multiple layers of drugs/biomolecules,14,18,19 thereby augmenting the effect of immobilization. For the parallel reason, patterned surfaces may also behave as an ECM (extracellular matrix) mimicking the biological environment to promote cell growth in a specified fashion. Strategies to develop polymer brush based micropatterned surfaces mostly exploit inorganic or non-degradable polymers as the underlying substrate.20–24 There is increasing interest towards biodegradable polymers because of their eco-friendly and biocompatible nature.25,26 Also, biodegradable polymers are extensively used as a biomedical implant and scaffold for tissue engineering,18,27–29 the surfaces of which may require micropatterning for the said purposes. To the best of our knowledge, creation of micropatterned surfaces on biodegradable polymers via grafting of polymer brushes has not been reported to date. The main challenge lies in growing hydrophilic polymer brushes on a biodegradable polymeric substrate without altering the molecular weight of the underlying substrate which is susceptible to hydrolytic cleavage/degradation. Various researchers including us have reported the attachment of polymer brushes on biodegradable polymeric substrates such as PLA, PCl, etc.19,30via surface aminolysis which causes significant degradation of polymeric backbones as revealed from their reduced molecular weight after the aminolysis step. In contrast to this, the methodology used in this study and our previous publications as well18,19,31 doesn’t suffer from significant loss of molecular weight of the substrate post immobilization of the ATRP initiating moiety. Thus, grafting of polymer brushes in a designated domain to construct a micropatterned surface using spatio-selective chemistry, e.g., photochemistry, by directing the light through a suitable mask appears to be completely feasible on a biodegradable polymeric substrate with negligible loss of its molecular weight. Moreover, a versatile biodegradable platform modified with dual polymer brushes in designated areas for precisely adhering and repelling cells, bacteria, etc. in an alternate fashion is an unmet need. In order to avail this, a strategy based on photoinduced anchoring of dual polymer brushes in irradiated areas of a biodegradable polymeric surface has been adopted and discussed in this piece of work. Briefly, a photo masked film made of polymer P (a tartaric acid based aliphatic polyester P containing protected hydroxyl functionality) and PAG (photoacid generator) was exposed to UV radiation (λ: 345 nm) in a sequential way. PAG was specifically used to deprotect the hydroxyl group of polymer P upon UV irradiation which triggered acid formation from PAG to unmask hydroxyl groups. This path was followed to generate functional groups onto the surface of the biodegradable polymer without degrading its backbone.18 Following initiator immobilization in the UV exposed area by reacting the ATRP (atom transfer radical polymerization) initiating moiety with the unmasked hydroxyl group, dual hydrophilic brushes such as poly(ethyleneglycol)methyl ether methacrylate (polyPEGMA) and poly(3-dimethyl-(methacryloyloxyethyl) ammonium propane sulfonate) (polyDMAPS) were grafted in alternate regions of the exposed area using SIATRP (surface initiated ATRP) in 2 steps. Neutral polyPEGMA32 and zwitterionic polyDMAPS33 brushes were chosen to act as a human cell repellant and cell adherent, respectively. However, they were expected to behave oppositely for bacterial cells driven by stronger hydration of the zwitterionic brush over the neutral polyPEGMA brush.34 This may lead to the formation of a patterned surface consisting of cells or bacteria in an alternate fashion. Ultimately, the patterned surface modified with dual polymer brushes was demonstrated to enable adherence and growth of cells as well as bacteria in desired regions, thereby forming a microarray of cells or bacteria. Therefore, the scope of this innovative micropatterned surface comprised of dual polymer brushes of suitable functionality grafted on a biodegradable polymeric surface is wide and can be tuned for adherence of various types of biomolecules in a desired fashion. This may open up new avenues to create biochips, biosensors, bioassays, diagnostics, etc.35
Experimental part
Materials and methodology
1,6-Hexanediol, 2,2-dimthoxypropane, p-toluene sulfonic acid, tartaric acid and cyclohexane were purchased from Spectro Chem Pvt Ltd, India. 2-Bromoisobutyryl bromide (BIBB) was procured from Alfa Aesar. 3-Dimethyl-(methacryloyloxyethyl) ammonium propane sulfonate (DMAPS), poly(ethylene glycol)methyl ether methacrylate (PEGMA, Mn = 950), 2,2′ bipyridine (BPY, 99%), copper(I) bromide (CuBr, 99%), copper(II) bromide (CuBr2, 97%), fluorescein diacetate (FDA), propidium iodide, 2-(4-methoxystyryl)-4,6-bis(trichloromethyl)-1,3,5 triazine as a photoacid generator (PAG) and titanium tetraisopropoxide were received from Sigma Aldrich. Polylactic acid (PLA, Mn ∼ 80
000, 43032D) was procured from Nature works, USA. The solvents such as dichloromethane (DCM), chloroform and methanol (HPLC grade) were purchased from Fisher India. Prior to use, the solvents were purified and dried using standard procedures. Doubly distilled water was used for all the experiments. The antibacterial activity was assessed against the E. coli (Escherichia coli) DE3 gold strain which was purchased from Agilent Technologies, USA, and S. aureus (Staphylococcus aureus), MRSAATCC43300, was a gift from Department of Microbiology, All India Institute of Medical Sciences, Delhi, India. In addition, nutrient agar and luria broth were bought from Hi-Media Inc, India.
Light triggered deprotection of polymer P and immobilization of the ATRP initiator on the tartarate based polyester (P) surface
Synthesis of the monomer and polymer P is described in detail in the ESI.† Polyester P as shown in the reaction scheme (Fig. 1) was deprotected by illuminating UV light following the procedure given below. Around 500 mg (1.83 mmol) of polymer P was combined with 50 mg of PAG in the presence of chloroform and then dried completely to remove the solvent and dispersed in 10 mL of water to form a milky suspension (turbid) due to the insolubility of the polymer in water. Finally, a portable UV lamp (PerkinElmer Lambda 900 nm) (345 nm) was used to radiate the suspension kept in a vial for different time intervals such as 5, 10, 15, 20 and 25 min. The milky solution became transparent after 25 min of UV exposure. The UV exposed polymer sample (exposed for 25 min) was then evaporated to dryness by using a rotary evaporator and the yield was found to be around 90.4%. The resultant polymer was characterized by proton NMR and gel permeation chromatography (GPC, Waters GPC equipped with a 1515 isocratic HPLC pump and also a 2414 refractive index detector) to obtain its structural information and molecular weight, respectively. Following this, surface functionalization was carried out by radiating the film made of polymer P. Polymer P (500 mg) combined with PAG (50 mg) was dissolved (10 mg mL−1) in chloroform and spin coated on a glass substrate (1 cm × 1 cm) uniformly by using a spin coater (spin NXG-P1) at 200 rpm. In the subsequent step, the film was exposed to UV radiation for 25 min. It was then washed by water and stored in a vacuum desiccator until further use. A similar procedure was adopted for a film made of PLA and P at a ratio of 95
:
5 dissolved in chloroform (10 mg mL−1). The amount of PAG (50 mg) was kept the same for all the cases. All the UV exposed samples were dipped in 10 mL of anhydrous hexane (dried on molecular sieves). To this, 0.5 mL of 2-bromo isobutyral bromide (BIBB) and 0.2 mL of dry pyridine (dried on molecular sieves) were added in the presence of nitrogen. The reaction mixture was maintained at 0 °C for 2 h and then kept at room temperature for another 12 h. This resulted in the formation of P1 Br as shown in Fig. 1. The substrates were scrupulously washed with plenty of water and methanol combined in 1
:
2 v/v ratio and finally dried under vacuum and stored until further use. The molecular weight of the modified film was measured by dissolving it in THF and analysed by GPC. To prepare a patterned surface, a TEM grid was placed on the blend's surface and irradiated with UV light to functionalize the exposed surface. Following UV exposure, the masked sample was subjected to the initiator immobilization step in the same way as described above. After treatment, all the samples were dried under vacuum in a casting chamber for about 24 h at room temperature and stored in a desiccator until further use.
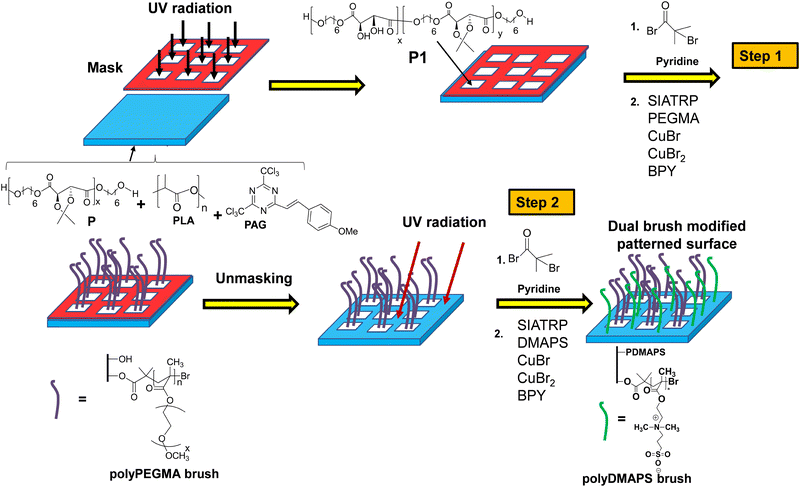 |
| Fig. 1 Schematic diagram illustrating the synthesis of a patterned surface modified with dual polymer brushes, i.e., polyDMAPS and polyPEGMA onto the tartarate based polyester using surface-initiated ATRP. | |
Surface initiated ATRP of polyPEGMA, polyDMAPS and patterned polymer brushes on initiator immobilized polyester surfaces
Poly(DMAPS) and polyPEGMA brushes were grafted on the polyester surface via SIATRP following the procedure described below. Around 2.6 g of DMAPS in 6 mL of distilled water was kept in a round bottomed Schlenk flask with continuous stirring at room temperature under the flow of nitrogen. Then, bipyridine, CuBr2 and CuBr were added in a molar feed ratio of [DMAPS]
:
[CuBr]
:
[CuBr2]
:
[bipyridine] = 100
:
1
:
0.2
:
1.2 to the Schlenk flask. Afterwards, the reaction mixture was made to undergo 3–4 freeze pump thaw cycles for the removal of oxygen. Finally, the solution was transferred with the help of a glass syringe to another round bottom flask containing a set of dry initiator immobilized polyester films coated on glass substrates under the nitrogen flow. After a certain period of time, these films were taken out from the flask and they were thoroughly washed with double distilled water before placing them in the vacuum desiccator for vacuum drying. Ultimately, clean and dry substrates were stored in the desiccator till further use. A similar procedure was adopted for polyPEGMA brush grafting and also for the patterned surface using the same condition and reagent ratio as mentioned above. For the patterned surface, after grafting of the polyPEGMA brush in the exposed area, the mask was removed and the same substrate was exposed to UV radiation for 25 min followed by initiator immobilization. After this, the polyDMAPS brush was allowed to grow onto a previously masked area, thereby forming a patterned surface composed of dual polymer brushes. For the patterned surface, ATRP was carried out for 24 h to graft both polyDMAPS and polyPEGMA brushes. Similar procedures were repeated for the P2 surface prepared by blending PLA with P (PLA/P = 95/5). So, P1 represents the modified neat polymer surface and P2 represents the modified blend surface.
Characterization
Proton NMR experiments were conducted using a Bruker DPX-400 spectrometer operating at 400 MHz. While doing the NMR measurements, trimethyl silane and deuterated solvents were used as an internal standard and solvent, respectively. Molecular weight was determined by gel permeation chromatography (GPC, Waters GPC equipped with a 1515 isocratic HPLC pump and also a 2414 refractive index detector). The water contact angle was measured using a drop shape analyzer (DSA 100 KRUSS). Contact angles were checked at least three times at different points of the samples and the average values were taken. To determine hydration percentage for brush modified surfaces, three surfaces such as polyPEGMA, polyDMAPS and the patterned surface comprising of both polyPEGMA and polyDMAPS in their respective domains were dipped in PBS buffer solution for 24 hours at 37 °C. The samples were taken out the next day and placed onto a filter paper to absorb excess water. The weight of the samples was precisely measured before and after soaking them in water. The difference in weight provides the amount of water absorbed and hydration percentage. The whole process was repeated for three samples taken from each category and an average value was reported for each sample. Topology of the surface for all the substrates was examined by AFM (atomic force microscopy, INNV – Base Innova SPM system, INSC-090) imaging. A 90 micron large area scanner was used for the Innova scanning probe microscope for capturing the image under the tapping mode under ambient conditions. The average roughness (Ra) was obtained from AFM images using Nanoscope software version 1.5. The chemical components of the surfaces were characterized by XPS (X-ray photoelectron spectroscopy) having an angle between the analyzer and the source at around 85 degrees. The XPS analysis data were obtained on an Omicron ESCA (electron spectroscope for chemical analysis) by exploiting a monochromatized Al X ray source (1487.3 eV electron). To obtain the infrared absorption spectra of the modified film, spectra were collected from the range of 4000 to 400 cm−1 using Fourier transform infrared spectroscopy (FTIR) in ATR (attenuated total reflectance) mode (PerkinElmer, Waltemath, MA). Bacterial growth on the surfaces was analyzed using a scanning electron microscope (Zeiss EVO50) at 20 kV. The images of live cells on the P2 blend surface were obtained by using a cell imaging multi-mode reader (Cytation 3, Biotek, USA). The experimental details of the antibacterial study and their anaylysis are given in the ESI.†
Protein adsorption test
The protein adsorption study was carried out by using FITC (fluorescein isothiocyanate) conjugated albumin following a dye interaction method.19,36 For investigating the protein adsorption on the polymer brush modified surface, it was dipped in a solution of FITC-albumin (0.1 mg mL−1 concentration prepared in PBS buffer) at room temperature. After 24 hours, the film was taken out and the substrate was thoroughly rinsed first with PBS and then with DI water for the removal of unbound protein. Subsequently, the surfaces were analyzed under a fluorescence microscope (Olympus microscope IX 73). Additionally, the total amount of protein present in the supernatant was also determined by the micro-BCA (bicinchoninic acid) protein assay following a literature reported protocol.37 Absorbance was measured at 562 nm using a microplate spectrophotometer (Epoch, BioTek, USA). The experiment was performed in triplicate. The total amount of protein adsorbed on the scaffold was estimated accordingly.
Cell adhesion study
For cell adhesion studies, all the samples such as the P2 surface, dual brush modified patterned surface, non-patterned individual brush grafted surface, etc. were sterilized by exposing to UV for 30 min and then placed in a 96 well plate. L929 mouse fibroblast cells at a density of 8 × 103 in DMEM media (Dulbecco's modified Eagle's medium) containing 10% FBS and 1% penicillin–streptomycin were seeded on the surfaces and incubated for 24 h at 37 °C with 5% CO2. After 24 h, the medium was removed and the wells were washed thrice with Hanks’ balanced salt solution (HBSS). The live staining of cells was performed by adding 1 μM Calcein-AM dye for 30 min at 37 °C. After 30 min, the dye was removed and the samples were washed thrice with HBSS. The live cells on the surface of polymers were visualized at 4× by using a multi-mode cell imager (Cytation 3, Biotek, USA).18,38
Results and discussion
Immobilization and characterization of polymer brushes on a micropatterned polyester surface using surface initiated ATRP
In order to create a micropatterned brush modified polymeric surface, we have explored a light responsive system where light can be selectively used to functionalize a designated area of a biodegradable polymeric surface with the help of a photomask. Subsequently, the light illuminated areas can further be exploited to spatioselectively grow polymer brushes with suitable functionality so that bacteria patterning and/or cell guiding arrays can be constructed on the polymeric substrate. In order to do so, we have selected an acid sensitive acetal protected biodegradable aliphatic polyester derived from tartaric acid that can be easily deprotected in the presence of photolabile acids such as PAG. PAG generates free acid upon UV irradiation and deprotects the hydroxyl group to create hydroxyl functionality onto the illuminated surface of the aliphatic polyester as shown in Fig. 1. At first, the tartaric acid-based aliphatic polyester P of molecular weight ∼14
500 (
n = 14
500,
w = 36
398, PDI (ĐM) = 2.93) was synthesized as per the published procedure18 and blended with PLA at a ratio of 5
:
95 (P
:
PLA) in chloroform. While blending with PLA, a certain amount of PAG (10% of P) was incorporated in the mixture to entrap the photo acid into the polymer matrix and finally a thin film (∼67 ± 2 μm thick) was prepared out of this blend on a glass substrate using a spin coater. Prior to the formation of the PAG caged polymeric film, we tested the ability of the photo acid in deprotecting the polymer P by dispersing the PAG entrapped polymer P matrix (not the blend) in water and irradiating UV light onto the solution for different time periods. The milky solution turned clear upon UV exposure for a certain time period indicating the successful deprotection of polymer P leading to dissolution of deprotected polymer P1 in water. A control experiment was carried out by irradiating polymer P dispersed in water without incorporating PAG in it and the solution remained milky. This clearly reflects the role of PAG in unmasking hydroxyl functionality which facilitated the dissolution of P1 in water. In order to optimize the irradiation time, the dispersed polymer was exposed to UV radiation for 5, 10, 15, 20 and 25 min for deprotection of the polymer P to introduce hydroxyl functionality. Direct excitation of entrapped PAG by UV light led to the photolytic-cleavage of the carbon chlorine bond of PAG to generate HCl which ultimately deprotected the isopropylidene groups of polymer P to unmask hydroxyl functionality leading to the formation of polymer P1. Based on the unchanged molecular weight, reduced contact angle (Fig. S1, ESI†) and enhanced water solubility of the deprotected polymer, 25 min was found to be the optimized time for UV irradiation beyond which the molecular weight of the polymer started to decline probably due to the main chain scission of the polyester in the presence of the released HCl. Till 25 min of UV irradiation, no change of molecular weight of the polyester was observed (Mn ∼ 14
500), though the contact angle was found to decline with irradiation time indicating surface hydrophilicity due to deprotected hydroxyl groups. However, a drastic change in molecular weight was observed when the sample was exposed to UV radiation for 30 min, i.e., the molecular weight of P1 went down from ∼14
500 Da to ∼7500 Da (
n = 7500,
w = 26
398, PDI (ĐM) = 2.10) indicating the degradation of the polyester by the released HCl. The amount of HCl released from PAG after 25 min was estimated to be ∼167 ppm as determined from both acid base titration and chloride ion concentrations as well (see the ESI† for details, Fig. S2f). Since around 290 ppm of HCl (0.08 mmol) was found to be released from the same amount of PAG (0.11 mmol) upon UV illumination for 1 hour, ∼58% (167 ppm) HCl was observed to be released after 25 min, and that was sufficient to deprotect the polymer P for subsequent functionalization. Proton NMR analysis before and after UV radiation (Fig. S2, ESI†) further confirmed the removal of around 33% isopropylidene groups (as estimated from NMR, Fig. S2e, ESI†) from polymer P (Fig. 1) upon UV irradiation and all the peaks appearing in the NMR spectrum (was also taken in D2O to confirm the presence of OH) for deprotected polymer P1 correlated well with the literature.18,39 After optimizing the radiation time for the dispersed sample, a spin coated film made of polymer P containing 10% PAG entrapped in it was prepared on a glass substrate and exposed to UV radiation for 25 min. Then, the surface of the film was characterized by ATR-IR and contact angle measurements (Fig. 2a and Fig. S1, ESI†). The appearance of a broad peak centered at 3476 cm−1 corresponding to O–H stretching in the ATR-IR (Fig. 2a) spectrum of polymer P1 further confirmed the unmasking of hydroxyl functionality upon UV irradiation.40,41 All the other signature peaks for polymer P1 agreed well with the reported literature.18,19 In the subsequent step, unmasked surface hydroxyl groups (Fig. 1) were functionalized with 2-bromoisobutyral bromide to immobilize the ATRP initiating moiety onto the surface of the P1 film for growing neutral brushes such as polyPEGMA and polyzwitterionic brushes via SIATRP.42,43
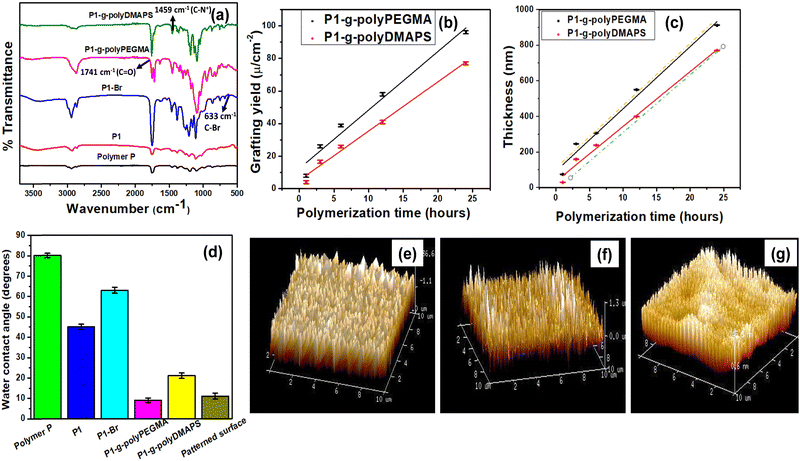 |
| Fig. 2 (a) Representative ATR-FTIR spectra of pure polymer P, P1, P1–Br, and homo polymer brush (polyPEGMA and polyDMAPS) modified surfaces. (b) Variation of the ‘Grafting yield' of polyDMAPS and polyPEGMA with respect to polymerization time grafted on the P1–Br surface. (c) Thickness of the polyDMAPS and polyPEGMA brush determined from ‘Grafting yield’ values (solid line) and AFM images (dotted line) with respect to polymerization time. (d) Variation of the water contact angle after each step of surface modifications of pure polymer P, P1, P1–Br, P1-g-polyDMAPS, P1-g-polyPEGMA and dual brush modified patterned surfaces (polymerization time: 24 h). (e) AFM topography image obtained from the P1-g-polyDMAPS (polymerization time: 24 h) surface. (f) AFM topography image obtained from the P1-g-polyPEGMA (polymerization time: 24 h) surface. (g) AFM topography image obtained from the dual brush modified patterned surface (polymerization time: 24 h). Scale bar for all AFM images = 10 microns. | |
A negligible change in molecular weight was observed for the P1 film (
n = 13
990,
w = 35
388, PDI (ĐM) = 2.71) and P1–Br (
n ∼ 13
245,
w = 33
990, PDI (ĐM) = 2.65) after deprotecting hydroxyl functionality and immobilizing the ATRP initiating moiety for 12 h, respectively, as compared to the pure polyester P (
n ∼ 14
500, PDI (ĐM) = 2.93) indicating minimal degradation of the polyester backbone during functionalization steps. After every modification step of the P1 film, the surfaces were characterized by NMR, contact angle, ATR-IR, Raman, EDX and XPS to confirm the modifications. Expectedly, the contact angle was found to decline after unmasking the hydroxyl groups; however, after the bromo-functionalization step, a concomitant increase in contact angle resulted due to the surface coverage with the isopropyl group (hydrophobic) originating from the alkyl bromide conjugation step (Fig. 2d).
The appearance of a feeble peak at 633 cm−1 in the ATR-IR spectrum of P1–Br corresponding to C–Br stretching further confirmed the immobilization of the ATRP initiator onto the surface of the polyester (Fig. 2a).19,22,40,44 In addition, XPS analysis was also carried out to confirm the same, but on a blend surface (blending of PLA with P at a weight ratio of 95
:
5 keeping 10 wt% PAG w.r.t. P, referred to as P2), instead of the pure P1 film as running XPS on the sticky P1 film was not possible. C 1s, N 1s, O 1s, S 2p and Br 3d core spectra were obtained from the P2–Br surface (after modification of P2 with 2-bromoisobutyral bromide in the same manner as described above) and deconvoluted into respective constituents as depicted in Fig. 3.
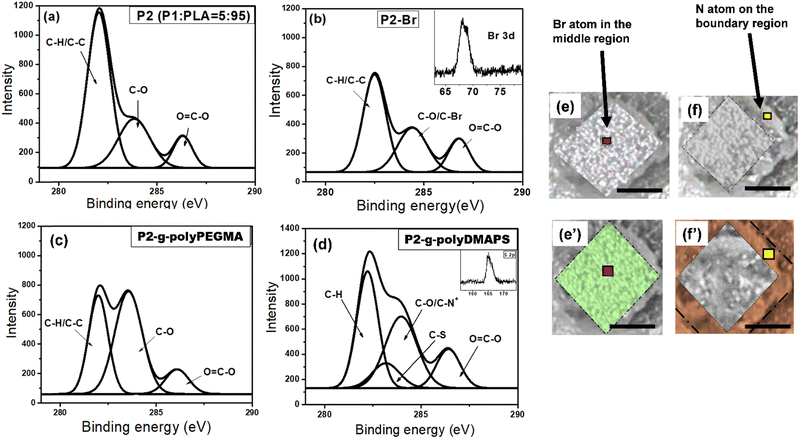 |
| Fig. 3 Deconvoluted C 1s core-level spectra for various surfaces: (a) P2 (P/PLA = 5/95), (b) P2–Br after the first step of bromo functionalization (Br 3d spectrum shown as the inset and its corresponding XPS image shown in panels (e) and (e′) with a pink pointer), (c) P2-g-polyPEGMA brush (polymerization time: 24 hours) after the first ATRP, and (d) P2-g-polyDMAPS brush (polymerization time: 24 hours) after the second ATRP (S 2p spectrum shown as the inset). (e and e′) Representative XPS images mapped for the Br atom (the region labeled with green) after the first step of bromo functionalization and (f and f′) representative XPS images mapped for the N atom from the polyDMAPS brush after the second ATRP step (scale bar: 50 microns). | |
The core level spectra for C 1s and Br 3d acquired from the P2–Br surface further supported the immobilization of the bromo functionalized ATRP initiator onto the P2 surface which was initially devoid of the peak related to bromo functionality18 (Fig. 3b). Subsequently, polymer brush growth such as that of polyPEGMA and polyDMAPS via SIATRP afforded the P2 surface modified with a homogeneously distributed dense polymer brush with good surface coverage as evident from AFM images (Fig. 2e and f) acquired from the brush modified surfaces. A distinctly different surface morphology covered with bristles of polymer chains with rougher surface (average surface roughness: 101 nm for polyPEGMA and 290 nm for polyDMAPS) is clearly visible in Fig. 2e and f for brush modified surfaces as compared to the AFM image acquired from the initiator immobilized surface (average surface roughness: 30 nm). Moreover, a continuous reduction of contact angle with the progress of polymerization for both PEGMA and DMAPS further indicates a good surface coverage with hydrophilic polymer brushes (Fig. 2d),45–47 The ATR-FTIR spectrum obtained from the zwitterionic polyDMAPS brush modified surface corroborates well with its characteristic peaks. For example, the occurrence of a band at 1459 cm−1 corresponding to C–N+ bending vibrations, a set of strong bands in the range of 1056 cm−1 to 1278 cm−1 attributed to S–O stretching and a moderately powerful band at around 679 cm−1 for an umbrella kind of resonating movement of the oxygen atoms (bending vibrations) are distinctly evident from Fig. 2a, further confirming the successful grafting of polyDMAPS brushes onto the polyester surface.48 In addition, the distinctive band of carbonyl groups (ν(C
O) at 1749 cm−1) from the ester moiety of polyDMAPS brushes was found to be overlapping with the ester group (ν(C
O)) of the polyester support.49 In the case of the polyPEGMA brush modified surface, a peak at 1741 cm−1 corresponding to C
O stretching and a set of characteristic C–O peaks for wagging, twisting, stretching and rocking modes found at 1451 cm−1, 1299 cm−1, 1270 cm−1 and 1069 cm−1, respectively, clearly support the presence of polyPEGMA brushes on the polyester surface.50 Apart from these, brush thickness and grafting yield of the tethered brush were also determined from AFM images (using Nano scope Analysis Software) and by weighing the surfaces after modification, respectively.45 The data shown in Fig. 2b and c suggest a linear brush growth on the polyester surface irrespective of the type of monomer, as desired from a controlled polymerization technique like ATRP.51 Expectedly, thicknesses determined from AFM as well as FESEM images correlate well with each other and also conform well with the thicknesses calculated from grafting yield values, further confirming the uniform and controlled chain growth on the polyester surface (Fig. 2c).18,19 Nevertheless, the polyPEGMA brush follows a faster growth kinetics as compared to the polyDMAPS brush probably due to less interference of the PEGMA monomer with the copper complex (used during ATRP) than that of DMAPS.52,53 Ultimately, 24 h of polymerization was the condition to grow the brush with highest thickness for both the brushes (as beyond 24 h, no change of thickness was observed), and was chosen to make the pattern surface (discussed below) covered with two different brushes in their respective domain.
In order to fabricate a micropatterned surface, the solution of blend P2 (PLA
:
P = 95
:
5) with 10% PAG content (10% w.r.t. P) was uniformly spin coated on a glass substrate which was partially covered with a photomask (TEM grid) in the subsequent step. Then the masked surface was exposed to UV radiation for 25 min to incorporate hydroxyl functionality on uncovered areas which underwent subsequent functionalization with 2-bromoisobutyral bromide to immobilize the ATRP initiating moiety for the time period of 12 h.18 Following this, hydrophilic polyPEGMA brushes were selectively allowed to grow from initiator immobilized areas only as evidenced from the reduced contact angle of 8 degrees for the polyPEGMA brush grafted on unmasked areas (Fig. 2d). In the next step, the whole surface was unmasked and further exposed to UV radiation followed by initiator immobilization in the same way as described above. At this stage, zwitterionic polyDMAPS brushes were tethered onto the previously masked but newly exposed area to create a well-defined patterned surface having neutral and zwitterionic brushes in their respective domains. Both brushes were allowed to grow for 24 h beyond which no change of thickness or grafting yield was observed.54 It is worth mentioning that the chance of formation of block copolymer brushes of polyPEGMA-b-polyDMAPS during polyDMAPS brush formation is slim, since UV irradiation in the second step may terminate surface radicals responsible for ATRP initiation.54 Desirably, no signature peaks of polyDMAPS in the polyPEGMA region were seen in the confocal Raman spectra (Fig. 4a) acquired from the site specific region of the dual brush modified surface, thereby confirming the same (discussed later).
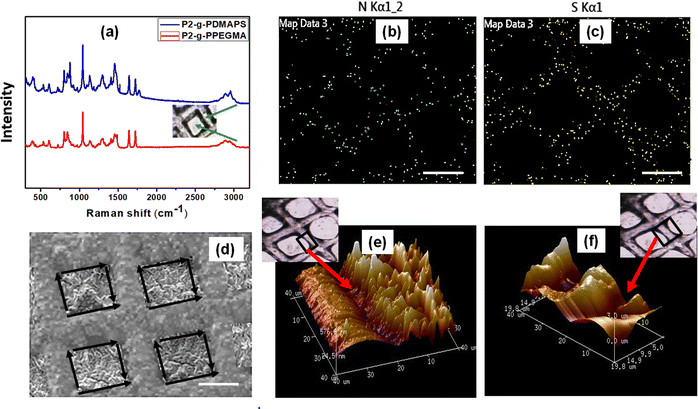 |
| Fig. 4 (a) Representative Raman spectra of polyPEGMA and polyDMAPS brush modified P2 surfaces, (b) and (c) EDX mapping for N and S atoms acquired from the polyDMAPS brush modified region, (d) SEM image for the patterned surface modified with dual polymer brushes (arrows represent the interface of dual brushes) and (e) and (f) AFM topography image obtained from the polyPEGMA brush modified surface after the 1st ATRP acquired from various regions shown by red arrows in optical images. Scale bar = 10 microns. | |
XPS analysis in selective areas along with imaging of patterned surfaces was carried out to confirm brush growth in selective domains. Deconvolution of C 1s, N 1s, S 2p and O 1s spectra to respective components acquired from the selective region was carried out and is displayed in Fig. 3. For the polyPEGMA brush modified area, the existence of three peaks with binding energy (BE) at 282.7 eV for the C–H/C–C bond, 283.3 eV for the C–O bond and 287.0 eV for the O–C
O bond from the C 1s spectrum with increased intensity as compared to the P2–Br surface correlates well with its structure having a long ethylene glycol unit.18,19 On the other hand, the core level spectra for C 1s generated from the polyDMAPS brush modified region can be curve fitted to four peak components with BEs at around 283.8 eV, 282.7 eV, 283.3 eV and 287.0 eV corresponding to C–H, C–S, C–O/C–N+ and O
C–O, respectively. Evidently, the C–S and C–N+ peak components originating from the polyDMAPS brush modified region further indicate the selective immobilization of both brushes in their respective domain.53 The [Br/C] ratio after the initial bromo functionalization step (step-1 in Fig. 1), as estimated from the Br 3d and C 1s spectra acquired from P2–Br surface of the unmasked region (Fig. 3), was found to be around 16.77 × 10−3. The same was found out after the second bromo functionalization step and the ratio (15.87 × 10−3) remains almost the same as observed in the first step (Fig. 3 and Fig. S3a, ESI†). From the [Br/C] ratio, initiator density can be determined to be approximately 3.94 chains per nm2 for both the cases (Fig. 3 and Fig. S3a, ESI†).55 Expectedly, no Br was detected from the brush modified surface after the first polymerization step. All the information derived from XPS spectra agreed well with the published literature.56–58 It is noteworthy that the polymer brushes grafted onto the blend surface were at least 400 nm thick which was significantly higher than the penetration depth (7.5 nm) of X-rays used during XPS; hence the compositions derived from the peak constituents certainly originated from the brush modified region only, and not from the underlying polyester surface. EDX analysis (Fig. S3b and c, ESI†) and elemental mapping (Fig. 4b and c) were also accomplished to further confirm the findings from XPS. The elemental mapping of nitrogen and sulphur heteroatoms obtained from the micropatterned (Fig. 4b and c) brush modified surface clearly signifies the dominant presence of the polyDMAPS brush in the spatio-selective domain only,59 further confirming the successful immobilization of brushes in selective areas. Both XPS (Br and N atom mapping, Fig. 3) and EDX mapping (N and S atom mapping, Fig. 4b and c) were carried out post bromofunctionalization and post grafting of the polyDMAPS brush to map atoms such as Br, N and S, from their respective domains. The images from XPS and EDX mapping evidence the presence of specific elements (Br, N, and S) in the desired region of the micropatterned surface, thereby strengthening the conclusion60 obtained from previous results.
Spatio-selective Raman spectra obtained from specific regions of the patterned surface as observed by an optical microscope (Fig. 4a) further confirm the successful grafting of both brushes in their respective locations. All the characteristic peaks along with their assignment are summarized in Table S1 (ESI†) and agreed well with the literature.61,62
To investigate the surface morphology of the brush modified patterned surface, SEM and AFM (in tapping mode) images (Fig. 2g and 4d–f) were captured. Images of the P2 surface homogeneously modified with individual brushes and also the patterned surface comprised of dual brushes were captured by AFM. Patterned surfaces modified with both single brush and dual brushes were found to be homogeneously modified with brushes showing a high surface roughness (Ra) of ∼90 nm for polyPEGMA, ∼ 234 nm for polyDMAPS and ∼ 456 nm for the dual brush system63,64 (Fig. 2g and 4e,f) as compared to the unmodified surface (Ra ∼ 20 nm). Presumably, aggregated bristles of entangled zwitterionic chains and polyPEGMA chains segregated in their respective domains might make the surface rougher as compared to the unmodified one. A similar observation has also been reported in many studies.18,61,65 Nevertheless, well controlled polymerization yielded good surface coverage by both brushes as revealed from both AFM images and reduced contact angle as well (Fig. 2g and Fig. S1, ESI†).66,67 Brush thicknesses of the patterned surface determined from both SEM and AFM images tally well with each other and also with the thickness estimated from grafting yields as reported by us in our previous study18 (Fig. 2). Interestingly, dry and collapsed polymer brush accumulation was evident on the brush modified, patterned P2 surface as revealed from SEM and AFM images as well (Fig. 2g and 4d–f). A high contrast observed in the high magnification SEM image (Fig. 4d) acquired from the patterned dual polymer brush clearly evidences the presence of two distinct domains originating from the different morphology of neutral and zwitterionic polymer chains with two different densities and chemical distributions which further confirms the results obtained from EDX mapping (Fig. 4b and c). These results provide visual evidence for the successful creation of a well-defined micropatterned surface on a biodegradable polymeric surface with precisely tuned surface chemistry and size.68 A similar observation was reported by other researchers too on a styrene–ethylene–butylene–styrene SEBS substrate.69
Surface charge analysis
In order to understand the interaction of biomolecules such as proteins, cells, etc., it was important to determine surface charges of the brush modified surfaces. Instead of the patterned surface, surface charges for two distinct P2 surfaces homogeneously modified with polyDMAPS and polyPEGMA brushes along with the unmodified P2 surface (control sample) were measured. A shift in surface potential (ζ) at around pH 7.4 from −45 mV to −2 mV was observed upon modification of P2 with the zwitterionic polyDMAPS brush. A negative surface potential on the P2 surface probably originated from the carboxylate functionality that prevailed at the end chain of polyesters.70 However, due to variation in the relative strength of acid and base components of the zwitterionic brush, the polyDMAPS brush modified surface may show negative surface potential instead of exhibiting charge neutrality.71 On the other hand, a slight positive surface potential (around +5 mV) was found for polyPEGMA brush modified surfaces owing to its neutral nature and also probably due to association of metal ions72 (probably residual copper from ATRP) with a long ethylene glycol unit (Mn of PEGMA = 950) of polyPEGMA chains73 (Fig. S4, ESI†). Interestingly, the patterned surface composed of polyDMAPS and polyPEGMA brushes at their respective domains was found to remain in a neutral state since surface charge was close to zero (+1 mV). This may be attributed to association of neighbouring chains of dual brushes bearing slight opposite charges making the surface overall neutral.71,74,75 Generally, it may be expected that, almost neutral surface charges for the patterned surface may further enable the surface to act as an antiadhesive agent towards bacteria possessing charged surfaces such as Gram-positive and Gram negative bacteria.71 It may also inhibit non-specific adsorption of proteins as discussed below.
Analysis of protein adsorption on a patterned surface
Strongly hydrated, flexible, soft polymer brushes typically show resistance towards non-specific protein adsorption. This is essential for them to act as anti-fouling, anti-adherent as well as antibacterial surfaces, since hydrated polymer brushes may create an enthalpic barrier against protein/bacteria fouling.19 A low water contact angle (<7° for polyPEGMA, 21° for polyDMAPS and 10° for dual brush modified patterned surface) for brush modified surfaces reveals their hydrophilic and thus hydration capability. In order to demonstrate the protein resistance behavior of brush modified surfaces, three different substrates, namely a patterned surface composed of polyDMAPS and polyPEGMA brushes at designated places, P2–Br surface and P2 surface (control), were incubated for 24 h with a fluorescent-tagged protein, i.e., FITC-conjugated albumin solutions (0.1 mg mL−1 in PBS solution), and imaged under a fluorescence microscope post removal of the surfaces from protein solutions followed by scrupulous washing to remove non-adherent proteins. The fluorescent micrographs depicted in Fig. 5 displayed no detectable proteins onto the brush modified patterned surface, whereas significant green fluorescence was observed on both neat P2 and P2–Br surfaces suggesting a strong protein resistive characteristic of brush modified surfaces owing to their strong hydrophilicity. In addition, quantitative estimation suggests a minimal adsorption of protein on the brush modified patterned (2.56 μg cm−2) as well as non-patterned surface (polyDMAPS: 3.25 μg cm−2 and polyPEGMA: 2.89 μg cm−2), further confirming their non-fouling nature (Fig. 5). Similar results were also obtained from micro-BCA protein assay as displayed in Fig. S5 (ESI†), where the total amount of non-adsorbed protein present in the supernatant was determined. As per literature reports, both polyDMAPS and polyPEGMA brushes possess antifouling properties; thus our findings are in good agreement with the available literature.3,37,76,77
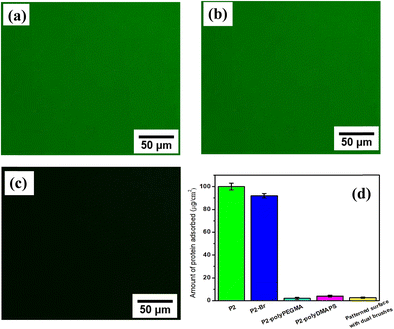 |
| Fig. 5 Fluorescent micrographs of (a) polymer blend P2, (b) P2–Br and (c) dual brush modified patterned surface (surface polymerization time was kept at 24 h). (d) Quantitative analysis of protein adsorption on various surfaces. All data points are shown as average + standard deviation (error bar). | |
Antibacterial evaluation
Fascinated by the antifouling characteristics of brush modified patterned surfaces, the antibacterial activity of brush modified patterned as well as non-patterned homogeneous surfaces along with P2 (control) was assessed against both Gram positive and Gram negative bacteria such as E. coli and S. aureus by following the spread plate method18 and the results are summarized in Fig. 6 and Fig. S6, S7 (ESI†).
 |
| Fig. 6 Comparison of bacterial growth on polymer P2, P2-g-polyDMAPS (polymerization time: 24 h) and P2-g-polyDMAPS (24 h) and patterned surfaces modified with dual brushes against (a) E. coli (5 samples) and (b) S. aureus (5 samples) bacteria exposed for 24 h. All data points are shown as average ± standard deviation represented by an error bar (p ≤ 0.05). | |
The antiadherent property of the zwitterionic polyDMAPS brush modified surface towards bacterial cells was found to be significantly higher (7% and 5.2% adherent bacteria for E. coli and S. aureus, respectively) as compared to the polyPEGMA modified one (21% and 19.6% adherent bacteria for E. coli and S. aureus, respectively). This may be attributed to the hydrated zwitterionic head group present in the polyDMAPS brush as revealed from its higher water uptake data (19.9%) as compared to the polyPEGMA brush (11.4%).78–81 As expected, no anti-adherent/antibacterial activity was observed for the P2 and P1 surface suggesting the role of hydrophilic brushes in repelling bacterial cells.
Strikingly, least bacterial fractions (4.6% for E. coli and 3.5% for S. aureus) were found on patterned surfaces, making them the most promising substrate for antibacterial applications. This may be attributed to the nanotextured surfaces originating from the presence of neighboring dual brushes which may enhance the surface roughness (Ra = 456 nm), thereby further reducing the bacterial adhesion in comparison with the non-patterned homogeneously modified brush surfaces (Fig. 6 and 7). A similar observation, i.e., reduced bacterial adhesion on the patterned surface, was reported by other researchers too.82 Nevertheless, the degree of bacterial attachment was relatively less for polymer brush modified surfaces,64 particularly for the polyDMAPS brush modified surface. This may be attributed to the presence of long hydrophilic flexible zwitterionic chains that can easily access and bind polarized water molecules through electrostatically induced solvation via positive and negative charges of zwitterions.83 This may generate a strong hydration sheath surrounding the flexible zwitterionic chains that can drastically reduce the bacterial adhesion (Fig. 7) as compared to neutral brushes such as polyPEGMA.84 The trend agrees well with the literature.84 Presumably, the polarized water molecules can be easily intruded to the zwitterionic chains and strongly bound via electrostatically induced solvation which is generally stronger than the H-bonded solvation prevailing in neutral polyPEGMA brushes.80 This was further supported by increased water uptake by polyDMAPS brushes as mentioned above. Hence, enhanced interfacial hydration forces may resist the bacterial adhesion in comparison with polyPEGMA brushes. However, dense colonies of bacteria with intact cell walls were found on the neat P2 surface clearly indicating the role of the brushes in reducing bacterial adhesion.
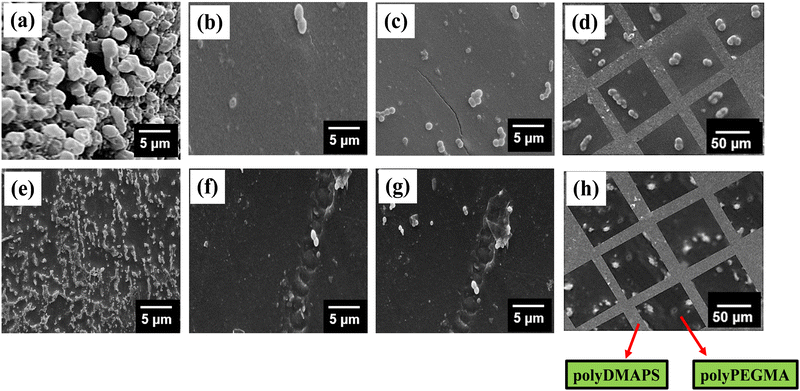 |
| Fig. 7 SEM images of E. coli and S. aureus obtained on the (a and e) P2 surface, (b and f) P2-g-polyDMAPS surface (24 h), (c and g) P2-g-polyPEGMA surface (24 h), and (d and h) patterned surface modified with dual brushes. Information in parentheses represents polymerization time. | |
Along with SEM images of adherent bacteria, fluorescence images were also captured for observing the adherent live and dead bacterial cells (stained with fluorescein diacetate (FDA) and propidium iodide for detecting live and dead cells, respectively) on various surfaces. As can be seen from Fig. 8, the neat P2 surface clearly facilitates the bacterial growth, whereas the brush modified surfaces including the patterned one (discussed below) show relatively less concentration of adherent live bacteria depending on the brush nature. Nevertheless, it is worth mentioning that only live bacteria (represented by the appearance of green dye only, but no red dye corresponding to dead bacteria) were visible on the surfaces suggesting their bacteriostatic nature instead of bactericidal as reported in the literature too.79
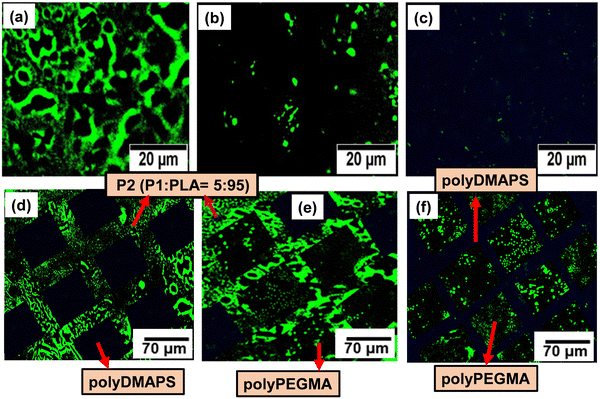 |
| Fig. 8 Fluorescence microscopic images for FDA-stained live cells of E. coli adhered to the (a) P2 surface, (b) P2-g-polyPEGMA surface, (c) P2-g-polyDMAPS surface, (d) patterned P2 surface modified with the single polyDMAPS brush, (e) patterned P2 surface modified with the single polyPEGMA brush and (f) patterned surface modified with dual brushes. | |
Based on the SEM and fluorescence images obtained from non-patterned, homogeneous brush modified surfaces (Fig. 7 and 8), it can be inferred that the patterned surface composed of dual brushes may show a difference in the density of bacterial attachment in individual domains of dual brushes. Expectedly, singly patterned polyDMAPS brush modified surfaces show absolutely no bacterial cell attachment (Fig. 7 and 8) on the zwitterionic brush domain due to its excellent resistance to bacterial adhesion, thereby forming an alternate pattern of bacteria, since they were well grown on the unmodified P2-surface. However, polyPEGMA brush modified singly patterned surfaces display reduced bacterial adhesion on the polyPEGMA domain as compared to the unmodified P2 surface (Fig. 8 and Fig. S8, ESI†). Interestingly, when both brushes were attached in their respective domain of the patterned surface, a distinct pattern of bacteria was evident as the degree of bacterial attachment was considerably higher on the polyPEGMA surface than on the polyDMAPS surface. It is noteworthy that the polyDMAPS brush domain completely resisted bacterial adhesion in the patterned surface, whereas a low fraction of bacteria was evident on the polyDMAPS brush modified homogeneous surface (non-patterned). A complete inhibition of bacterial adhesion from the patterned surface could be attributed to the micro/nano-texturing of surfaces. Overall, it can be stated that with the judicious selection of brush functionality, an alternate pattern of bacteria can be easily generated on the dual brush modified patterned surfaces.85 However, the reverse pattern could not be generated where zwitterionic polyDMAPS was initially grafted as it has a tendency to form a block copolymer with the polyPEGMA brush while running second ATRP as revealed from ATR-IR (Fig. S8, ESI†). This may be attributed to the interaction of zwitterionic chains (preferably the cationic site) with the carboxyl moiety of the bromo initiator while carrying out the second step of initiator immobilization.86,87 This may lead to the grafting of polyPEGMA chains on the zwitterionic chains in addition to the homo polyPEGMA brush grafted at its designated area. As a result, the whole surface was found to be covered with the polyPEGMA brush instead of forming a reverse pattern.
Cell adhesion on a patterned surface
Surface patterning with biomolecules aids in understanding various biological phenomena such as cell to cell interactions and their adhesion property. L929 cells were chosen as model cells to demonstrate their selective adhesion on the desired region of the brush modified patterned surface.38,88 Before performing the cell adhesion experiments on the patterned surface, cell adhesion on each of the polyDMAPS and polyPEGMA brush modified surfaces (non-patterned ones) along with the glass surface as a control (Fig. S9d, ESI†) was assessed to investigate the cytotoxicity of the substrates (Fig. 9a and b). Interestingly, the cells were found to be viable and able to adhere and proliferate in an elliptical shape on the polyzwitterionic (polyDMAPS) brush modified surface (Fig. 9a and Fig. S9, ESI†); however, only a few cells were observed to be present on the polyPEGMA brush modified surface (Fig. 9b) owing to its high hydrophilicity as revealed from its least contact angle (Fig. 2d). Similarly, for the brush modified patterned surface, cells were found to adhere and align along the region of the polyDMAPS brush instead of the polyPEGMA brush domain (Fig. 9c). A drastic difference in cell population was observed between the area of polyPEGMA and polyDMAPS brushes in the form of a square pattern as shown in Fig. 9c and d (51 cells per unit area for the patterned surface). Though both the brushes exhibited excellent non-fouling property, i.e., >99% inhibition of non-specific protein adsorption, dominant cell adhesion on the polyDMAPS brush modified surface (91 cells per unit area) instead of the polyPEGMA brush modified surface (5 cells per unit area) might have originated for the following reasons: (1) enhanced surface roughness observed for the polyDMAPS brush (Ra ∼ 290 nm) modified surface was indicative of good surface coverage with long bristles of zwitterionic chains entangled with each other due to interchain assembly of zwitterions, thereby forming a non-uniform rough surface as compared to the polyPEGMA one (Ra ∼ 101 nm). As per literature reports, the surfaces with higher roughness may facilitate cell adhesion as compared to smooth ones.89 (2) It is well known that a balance of hydrophobic and hydrophilic domains distributed throughout the surface may greatly influence the viability of cells,90,91 thus providing a positive environment for cells to adhere and grow. For a similar reason, the relatively less wetting and rougher polyDMAPS brush modified surface (contact angle: 21 degrees) may facilitate cell adhesion on zwitterionic brushes as compared to the super hydrophilic polyPEGMA brush (contact angle of ∼8 degrees). (3) Lower grafting density (41 μg cm−2) of the polyDMAPS brush may drive the brushes to fall into a collapsed mushroom regime which is considered to be a potent surface for cell adhesion as opposed to the brush regime with high grafting density as was the case for the polyPEGMA brush (77.23 μg cm−2).91 Moreover, water hydration on mushroom type zwitterionic brushes driven by electrostatic force was expected to be more as compared to neutral polyPEGMA brushes solvated by H-bonding only.89 Higher hydration observed for the polyDMAPS brush modified surface (amount of water absorbed: 15.67 μg cm−2, 19.9%) as compared to the polyPEGMA brush modified surface (amount of water absorbed: 11.40 μg cm−2, 11.4%) may further be correlated with the formation of mushroom type zwitterionic brushes in the case of the former. Furthermore, slightly higher hydration obtained for the patterned surface (amount of water absorbed: 24.33 μg cm−2, 21.6%) may be predominantly attributed to the zwitterionic domain facilitating cell adhesion. Contrary to this, a higher contact angle observed for the polyDMAPS brush modified surface may originate from its high surface roughness as rough surfaces tend to increase the water contact angle.92 Nevertheless, it is worth mentioning that the presence of a relatively compacted brush layer with good surface coverage was evident from the low hydration values irrespective of the type of brushes, thereby resisting the protein adsorption as mentioned before. It is noteworthy that a good surface coverage with the thick polyDMAPS brush would be essential as too low grafting density may not be sufficient to anchor cells and facilitate their proliferation, since a few cells were found to adhere on the surface when the polymerization time to grow the polyDMAPS brush was kept at 12 h or less (Fig. S9, ESI†). Similarly, Braden et al. found very few cells on the sulfobetaine containing polymer brush modified surface when the brush length was a few nm.93 Similar findings related to enhanced cell adhesion on thicker brushes were reported by others too.94 (5) As reported in our previous study95 (Table S4 of ref. 95, ESI†), the polyPEGMA brush modified surface exhibited lower hardness and reduced modulus as compared to the polyDMAPS brush modified surface. This may further facilitate cell adhesion on a relatively hard surface, i.e., the polyDMAPS region. As per literature reports, cells can adhere and proliferate via intracellular signaling which will be suppressed on a softer surface94,96 keeping them in a round shape (as observed for the softer polyPEGMA region) instead of a spread out elliptical shape as evident from the polyDMAPS brush modified hard domain (Fig. 9c and d). Collectively, cell adhesion on the polyDMAPS brush modified surface may then be primarily attributed to low softness and high surface roughness along with flexible, electroneutral hydrated zwitterionic chains as observed by others too.91 (6) A last but not least reason could be the sulfobetaine functional group which bears a resemblance to the chemical structure of 2-aminoethanesulfonic acid or taurine,97,98 a biomolecule abundantly present in the animal body. Therefore, the polyDMAPS brush modified surface may become enriched with biomimetic taurine groups which eventually facilitate cell adhesion and proliferation via zwitterionic and cell membrane interactions, though the surface was resistant to protein adsorption. Similar observations have been reported by other researchers too.89,93,99
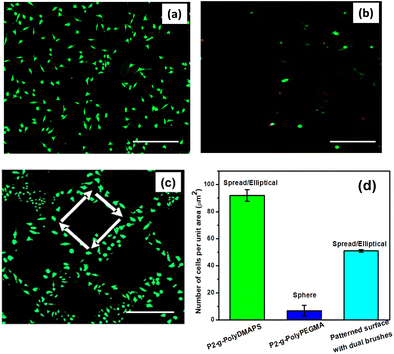 |
| Fig. 9 Fluorescence microscopic images for L929 cells (stained with Calcein-AM) grown on various surfaces: (a) P2-g-polyDMAPS (24 h), (b) P2-g-polyPEGMA (24 h) and (c) patterned surface modified with dual brushes (24 h). Scale bar: 50 μm (from a–c); information in parentheses represent polymerization time; arrows added to show the patterned region. (d) Number of adherent L929 cells on the brush modified patterned and non-patterned surfaces. Predominant morphology of the cells corresponding to each surface is written at the top of each bar in panel (d). | |
Considering all the factors stated above, a plausible illustration of the cell adhesion mechanism on the polyDMAPS brush modified domain is schematically presented in Fig. 10. Overall, it can be stated that a balance of interfacial amphiphilicity along with chain flexibility of biomimetic groups, low softness and high roughness may be collectively attributed to the spatio-selective cell adhesion on polyDMAPS brush domains of the patterned surface.
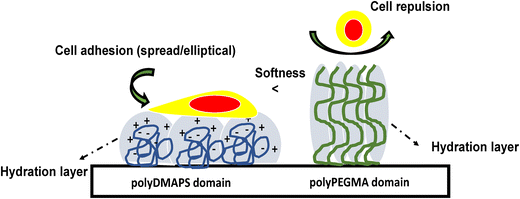 |
| Fig. 10 Plausible illustration of L929 cell adhesion and cell repulsion behavior on a selective region of brush modified patterned surfaces; pictorial representation of predominant cell adhesion on the polyDMAPS region and cell repulsion from the polyPEGMA region of the patterned surface. | |
Conclusion
Micropatterned dual brush coating on the surface of a tartaric acid based biodegradable aliphatic polyester was successfully accomplished to demonstrate spatio-selective arrangement of bacteria as well as fibroblast cells for constructing microarrays of bacteria/cells. The micropatterned surface was originally created on an aliphatic polyester surface (prepared by blending tartaric acid-based polyester and poly lactic acid (PLA) in the ratio 5
:
95) with the help of a photomask and PAG that was used to release acid upon UV radiation at 395 nm for 25 min to deprotect hydroxyl functionality present in the polyester. Ultimately, the hydroxyl groups from the irradiated unmasked area were used to anchor the ATRP initiating moiety for growing 902 ± 30 nm thick polyPEGMA brushes from that specific domain. Similarly, a 770 ± 45 nm thick zwitterionic brush such as the polyDMAPS brush was allowed to grow in the previously masked region by uncovering it in the second step and following the similar steps. Both the brushes were grown via ATRP and found to follow linear kinetics up to 24 h as expected. The dual brush modified micropatterned surface was thoroughly characterized by XPS, ATR-IR, RAMAN, contact angle, optical microscopy, AFM and SEM. The brush modified patterned surface was observed to be antifouling in nature (rejection of >97% proteins). Ultimately, an alternate pattern of adherent bacterial cells (E. coli and S. aureus) was found to form on the polyPEGMA brush modified domain, since almost complete rejection of bacteria from zwitterionic brush domains was evident. However, a reverse pattern of fibroblast cells was visible, where cells were found to adhere and align along the region of the zwitterionic brush instead of the polyPEGMA brush. A plausible mechanism of predominant L929 cell adhesion on the zwitterionic brush modified domain was schematically presented and discussed. This novel biodegradable micropatterned platform modified with dual brushes of suitable functionality offers promising applications in the biomedical area such as construction of bacteria/cell arrays, biochips, diagnostics, etc.
Conflicts of interest
There are no conflicts to declare.
References
- P. Costa, J. E. Gautrot and J. T. Connelly, Directing cell migration using micropatterned and dynamically adhesive polymer brushes, Acta Biomater., 2014, 10(6), 2415–2422 CrossRef CAS PubMed.
- C. de las Heras Alarcón,
et al., Bioadhesion at micro-patterned stimuli-responsive polymer brushes, J. Mater. Chem. B, 2005, 15(21), 2089–2094 RSC.
- M. Verma,
et al., Protein Patterning on Microtextured Polymeric Nanobrush Templates Obtained by Nanosecond Fiber Laser, Macromol. Biosci., 2022, 2100454 CrossRef CAS PubMed.
- S. Rahmani,
et al., Chemically orthogonal three-patch microparticles., Angew. Chem., 2014, 126(9), 2364–2370 CrossRef.
- I. Mirza and S. Saha, Biocompatible anisotropic polymeric particles: synthesis, characterization, and biomedical applications, ACS Appl. Bio Mater., 2020, 3(12), 8241–8270 CrossRef CAS PubMed.
- H. Takahashi and T. Okano, Thermally-triggered fabrication of cell sheets for tissue engineering and regenerative medicine, Adv. Drug Delivery Rev., 2019, 138, 276–292 CrossRef CAS PubMed.
- S. Sarkar,
et al., Development and characterization of a porous micro-patterned scaffold for vascular tissue engineering applications, Biomaterials, 2006, 27(27), 4775–4782 CrossRef CAS PubMed.
- M. Pardo-Figuerez,
et al., Controlled arrangement of neuronal cells on surfaces functionalized with micropatterned polymer brushes, ACS Omega, 2018, 3(10), 12383–12391 CrossRef CAS PubMed.
- M. Palacios-Cuesta,
et al., Patterning of individual Staphylococcus aureus bacteria onto photogenerated polymeric surface structures, Polym. Chem., 2015, 6(14), 2677–2684 RSC.
- C.-H. Choi,
et al., Preparation of bacteria microarray using selective patterning of polyelectrolyte multilayer and poly(ethylene glycol)–poly(lactide) diblock copolymer, Macromol. Res., 2010, 18(3), 254–259 CrossRef CAS.
- N. Thirumalapura,
et al., Lipopolysaccharide microarrays for the detection of antibodies, J. Immunol. Methods, 2005, 298(1–2), 73–81 CrossRef CAS PubMed.
- N. Thirumalapura,
et al., Bacterial cell microarrays for the detection and characterization of antibodies against surface antigens, J. Immunol. Methods, 2006, 309(1–2), 48–54 CrossRef CAS.
- S. Raina,
et al., Microbial quorum sensing: a tool or a target for antimicrobial therapy?, Biotechnol. Appl. Biochem., 2009, 54(2), 65–84 CrossRef CAS PubMed.
- S. Saha, Fabrication of topologically anisotropic microparticles and their surface modification with pH responsive polymer brush, Mater. Sci. Eng., C, 2019, 104, 109894 CrossRef PubMed.
- P. Jain,
et al., Completely aqueous procedure for the growth of polymer brushes on polymeric substrates, Langmuir, 2007, 23(23), 11360–11365 CrossRef CAS PubMed.
- S. S. Pradhan and S. Saha, Advances in design and applications of polymer brush modified anisotropic particles, Adv. Colloid Interface Sci., 2021, 102580 Search PubMed.
- Ifra, A. Singh and S. Saha, High Adsorption of α-Glucosidase on Polymer Brush-Modified Anisotropic Particles Acquired by Electrospraying—A Combined Experimental and Simulation Study, ACS Appl. Bio Mater., 2021, 4(10), 7431–7444 CrossRef CAS.
- S. Dhingra,
et al., Infection resistant polymer brush coating on the surface of biodegradable polyester, Mater. Sci. Eng., C, 2021, 118, 111465 CrossRef CAS.
- M. Verma,
et al., Antibacterial response of polylactide surfaces modified with hydrophilic polymer brushes, Iran. Polym. J., 2019, 28(6), 493–504 CrossRef CAS.
- A. M. Brzozowska,
et al., Biomimicking micropatterned surfaces and their effect on marine biofouling, Langmuir, 2014, 30(30), 9165–9175 CrossRef CAS.
- C. M. Nelson,
et al., Degradation of micropatterned surfaces by cell-dependent and-independent processes, Langmuir, 2003, 19(5), 1493–1499 CrossRef CAS.
- S. Saha, M. L. Bruening and G. L. Baker, Surface-initiated polymerization of azidopropyl methacrylate and its film elaboration via click chemistry, Macromolecules, 2012, 45(22), 9063–9069 CrossRef CAS.
- S. Saha, M. L. Bruening and G. L. Baker, Facile Synthesis of Thick Films of Poly(methyl methacrylate), Poly(styrene), and Poly(vinyl pyridine) from Au Surfaces, ACS Appl. Mater. Interfaces, 2011, 3(8), 3042–3048 CrossRef CAS.
- Ifra, A. Thattaru Thodikayil and S. Saha, Compositionally Anisotropic Colloidal Surfactant Decorated with Dual Metallic Nanoparticles as a Pickering Emulsion Stabilizer and Their Application in Catalysis, ACS Appl. Mater. Interfaces, 2022 DOI:10.1021/acsami.2c03255.
-
T. Aiswarya and S. Saha, Biocompatible Anisotropic Designer Particles, Advances in Sustainable Polymers, 2020, Springer, pp. 217–236 Search PubMed.
-
S. Saha, et al., Bio-plastics and biofuel: is it the way in future development for end users?, Plastics to Energy, 2019, Elsevier, pp. 365–376 Search PubMed.
- H. Patel, M. Bonde and G. Srinivasan, Biodegradable polymer scaffold for tissue engineering. Trends Biomater, Artif. Organs, 2011, 25(1), 20–29 Search PubMed.
- C. M. Agrawal and R. B. Ray, Biodegradable polymeric scaffolds for musculoskeletal tissue engineering, J. Biomed. Mater. Res., 2001, 55(2), 141–150 CrossRef CAS.
- A. T. Thodikayil, S. Sharma and S. Saha, Engineering Carbohydrate-Based Particles for Biomedical Applications: Strategies to Construct and Modify, ACS Appl. Bio Mater., 2021, 4(4), 2907–2940 CrossRef CAS.
- M. Klein Gunnewiek,
et al., Thin polymer brush decouples biomaterial's micro-/nanotopology and stem cell adhesion, Langmuir, 2013, 29(45), 13843–13852 CrossRef CAS.
- S. Dhingra,
et al., Cytocompatible, soft and thick brush-modified scaffolds with prolonged antibacterial effect to mitigate wound infections, Biomater. Sci., 2022, 10(14), 3856–3877 RSC.
- A. M. Telford,
et al., Micropatterning of polymer brushes: grafting from dewetting polymer films for biological applications, Biomacromolecules, 2012, 13(9), 2989–2996 CrossRef CAS PubMed.
-
Y. K. Feng, et al., Grafting sulfoammonium zwitterionic brushes onto polycarbonateurethane surface to improve hemocompatibility, Advanced Materials Research, Trans Tech Publ, 2011 Search PubMed.
- N. Kaga,
et al., Effect of micro/nano-patterned surfaces on cell adhesion of Ca9-22 cells, e-J. Surf. Sci. Nanotechnol., 2017, 15, 1–6 CrossRef CAS.
- M. E. Welch and C. K. Ober, Responsive and patterned polymer brushes, J. Polym. Sci., Part B: Polym. Phys., 2013, 51(20), 1457–1472 CrossRef.
- K. M. Woo,
et al., Suppression of apoptosis by enhanced protein adsorption on polymer/hydroxyapatite composite scaffolds, Biomaterials, 2007, 28(16), 2622–2630 CrossRef CAS PubMed.
- A. Banerjee, V. Koul and J. Bhattacharyya, Fabrication of in situ layered hydrogel scaffold for the co-delivery of PGDF-BB/chlorhexidine to regulate proinflammatory cytokines, growth factors, and MMP-9 in a diabetic skin defect albino rat model, Biomacromolecules, 2021, 22(5), 1885–1900 CrossRef CAS.
- S. Changotade,
et al., Preliminary in vitro assessment of stem cell compatibility with cross-linked poly(ε-caprolactone urethane) scaffolds designed through high internal phase emulsions, Stem Cells Int., 2015, 2015 DOI:10.1155/2015/283796.
- P. Larkin, General outline and strategies for IR and Raman spectral interpretation, Infrared Raman Spectrosc., 2011, 117 DOI:10.1016/B978-0-12-386984-5.10007-2.
-
P. Larkin, Infrared and Raman spectroscopy: principles and spectral interpretation, Elsevier, 2017 Search PubMed.
- T. H. Park,
et al., Photoswitchable particles for on-demand degradation and triggered release, Small, 2013, 9(18), 3051–3057 CrossRef CAS PubMed.
- J. Baggerman, M. M. Smulders and H. Zuilhof, Romantic surfaces: a systematic overview of stable, biospecific, and antifouling zwitterionic surfaces, Langmuir, 2019, 35(5), 1072–1084 CrossRef CAS PubMed.
- M. D. Rowe, B. A. Hammer and S. G. Boyes, Synthesis of surface-initiated stimuli-responsive diblock copolymer brushes utilizing a combination of ATRP and RAFT polymerization techniques, Macromolecules, 2008, 41(12), 4147–4157 CrossRef CAS.
-
S. Saha, M. L. Bruening and G. L. Baker, POLY 409-Effect of substrate in surface ATRP, ABSTRACTS OF PAPERS OF THE AMERICAN CHEMICAL SOCIETY, AMER. CHEMICAL SOC. 1155 16TH ST, NW, WASHINGTON, DC 20036 USA, 2008.
- H. Jiang,
et al., Improvement of hemocompatibility of polycaprolactone film surfaces with zwitterionic polymer brushes, Langmuir, 2011, 27(18), 11575–11581 CrossRef CAS PubMed.
- C. Leng,
et al., Probing the surface hydration of nonfouling zwitterionic and PEG materials in contact with proteins, ACS Appl. Mater. Interfaces, 2015, 7(30), 16881–16888 CrossRef CAS.
- J. Zhao,
et al., Improved biocompatibility and antifouling property of polypropylene non-woven fabric membrane by surface grafting zwitterionic polymer, J. Membr. Sci., 2011, 369(1–2), 5–12 CrossRef CAS.
- J. Yin and J. Zhou, Novel polyethersulfone hybrid ultrafiltration membrane prepared with SiO2-g-(PDMAEMA-co-PDMAPS) and its antifouling performances in oil-in-water emulsion application, Desalination, 2015, 365, 46–56 CrossRef CAS.
- J. Yang,
et al., Functionalization of polycarbonate surfaces by grafting PEG and zwitterionic polymers with a multicomb structure, Macromol. Biosci., 2013, 13(12), 1681–1688 CrossRef CAS PubMed.
- S. Tugulu and H. A. Klok, Surface modification of polydimethylsiloxane substrates with nonfouling poly(poly(ethylene glycol) methacrylate) brushes, Macromol. Symp., 2009 DOI:10.1002/masy.200950516.
- Y. He,
et al., Anti-biofilm surfaces from mixed dopamine-modified polymer brushes: synergistic role of cationic and zwitterionic chains to resist staphyloccocus aureus, Biomater. Sci., 2019, 7(12), 5369–5382 RSC.
- G. Emilsson,
et al., Strongly stretched protein resistant poly(ethylene glycol) brushes prepared by grafting-to, ACS Appl. Mater. Interfaces, 2015, 7(14), 7505–7515 CrossRef CAS.
- C.-W. Chu,
et al., Zwitterionic polymer brush grafting on anodic aluminum oxide membranes by surface-initiated atom transfer radical polymerization, Polym. Chem., 2017, 8(15), 2309–2316 RSC.
- C. G. Wang,
et al., Facile fabrication of concentrated polymer brushes with complex patterning by photocontrolled organocatalyzed living radical polymerization, Angew. Chem., Int. Ed., 2018, 57(41), 13504–13508 CrossRef CAS PubMed.
- F. Xu,
et al., Functionalized polylactide film surfaces via surface-initiated ATRP, Macromolecules, 2011, 44(7), 2371–2377 CrossRef CAS.
- A. Al-Ani,
et al., Tuning the density of poly(ethylene glycol) chains to control mammalian cell and bacterial attachment, Polymers, 2017, 9(8), 343 CrossRef.
- N. Morimoto,
et al., Membrane translocation and organelle-selective delivery steered by polymeric zwitterionic nanospheres, Biomacromolecules, 2016, 17(4), 1523–1535 CrossRef CAS.
- H. Sánchez-Morán,
et al., Understanding design rules for optimizing the interface between immobilized enzymes and random copolymer brushes, ACS Appl. Mater. Interfaces, 2021, 13(23), 26694–26703 CrossRef.
- Y. Hou,
et al., Atomically dispersed nickel–nitrogen–sulfur species anchored on porous carbon nanosheets for efficient water oxidation, Nat. Commun., 2019, 10(1), 1–9 CrossRef CAS.
- A. J. Barlow,
et al., Chemically specific identification of carbon in XPS imaging using Multivariate Auger Feature Imaging (MAFI), Carbon, 2016, 107, 190–197 CrossRef CAS.
- W.-H. Hsu,
et al., Thermosensitive double network of zwitterionic polymers for controlled mechanical strength of hydrogels, RSC Adv., 2019, 9(42), 24241–24247 RSC.
- A. Z. Samuel and S. Umapathy, Energy funneling and macromolecular conformational dynamics: a 2D Raman correlation study of PEG melting, Polym. J., 2014, 46(6), 330–336 CrossRef CAS.
- E. Kutnyanszky and G. J. Vancso, Nanomechanical properties of polymer brushes by colloidal AFM probes, Eur. Polym. J., 2012, 48(1), 8–15 CrossRef CAS.
- X.-Y. Zhang,
et al., Antimicrobial peptide-conjugated hierarchical antifouling polymer brushes for functionalized catheter surfaces, Biomacromolecules, 2019, 20(11), 4171–4179 CrossRef CAS.
- X. Liu,
et al., Construction of multiple generation nitriloacetates from poly(PEGMA) brushes on planar silicon surface for enhancement of protein loading, Phys. Status Solidi A, 2011, 208(6), 1462–1470 CrossRef CAS.
- X. Sui,
et al., Characterization and molecular engineering of surface-grafted polymer brushes across the length scales by atomic force microscopy, J. Mater. Chem., 2010, 20(24), 4981–4993 RSC.
- X. Wang,
et al., Length scale heterogeneity in lateral gradients of poly(N-isopropylacrylamide) polymer brushes prepared by surface-initiated atom transfer radical polymerization coupled with in-plane electrochemical potential gradients, Langmuir, 2006, 22(2), 817–823 CrossRef CAS.
- C.-G. Wang, H. W. Yong and A. Goto, Effective Synthesis of Patterned Polymer Brushes with Tailored Multiple Graft Densities, ACS Appl. Mater. Interfaces, 2019, 11(15), 14478–14484 CrossRef CAS.
- J. Hou,
et al., Facile Fabrication of Hierarchically Thermoresponsive Binary Polymer Pattern for Controlled Cell Adhesion, Macromol. Rapid Commun., 2018, 39(6), 1700572 CrossRef PubMed.
- L. Guo,
et al., Zeta potential and surface physico-chemical properties of atmospheric air-plasma-treated polyester fabrics, Text. Res. J., 2009, 79(15), 1371–1377 CrossRef CAS.
- S. Guo,
et al., Surface charge control for zwitterionic polymer brushes: tailoring surface properties to antifouling applications, J. Colloid Interface Sci., 2015, 452, 43–53 CrossRef CAS.
- R. Mroczka and A. Słodkowska, The properties of the polyethylene glycol complex PEG (Na+)(Cu+) on the copper electrodeposited layer by Time-of-Flight Secondary-Ion Mass Spectrometry. The new insights, Electrochim. Acta, 2020, 339, 135931 CrossRef CAS.
- S. Liufu, H. Xiao and Y. Li, Investigation of PEG adsorption on the surface of zinc oxide nanoparticles, Powder Technol., 2004, 145(1), 20–24 CrossRef CAS.
- K. Ishihara, T. Kitagawa and Y. Inoue, Initial cell adhesion on well-defined surface by polymer brush layers with varying chemical structures, ACS Biomater. Sci. Eng., 2015, 1(2), 103–109 CrossRef CAS.
- A. Steinbach,
et al., Selective adsorption of functionalized nanoparticles to patterned polymer brush surfaces and its probing with an optical trap, ChemPhysChem, 2013, 14(15), 3523–3531 CrossRef CAS PubMed.
- P. Liu,
et al., Correction: Anti-biofouling ability and cytocompatibility of the zwitterionic brushes-modified cellulose membrane, J. Mater. Chem. B, 2016, 4(37), 6279 RSC.
- D. Lou,
et al., Antifouling Membranes Prepared from Polyethersulfone Grafted with Poly(ethylene glycol) Methacrylate by Radiation-Induced Copolymerization in Homogeneous Solution, ACS Omega, 2020, 5(42), 27094–27102 CrossRef CAS PubMed.
- Y. Zhang,
et al., Self-adaptive antibacterial surfaces with bacterium-triggered antifouling-bactericidal switching properties, Biomater. Sci., 2020, 8(3), 997–1006 RSC.
- Z.-W. Tang,
et al., Antiadhesive zwitterionic poly-(sulphobetaine methacrylate) brush coating functionalized with triclosan for high-efficiency antibacterial performance, Prog. Org. Coat., 2016, 97, 277–287 CrossRef CAS.
- M. Kurowska,
et al., A simultaneously antimicrobial, protein-repellent, and cell-compatible polyzwitterion network, Biomacromolecules, 2017, 18(4), 1373–1386 CrossRef CAS PubMed.
- S. Dhingra, S. Sharma and S. Saha, Infection Resistant Surface Coatings by Polymer Brushes: Strategies to Construct and Applications, ACS Appl. Bio Mater., 2022, 5(4), 1364–1390 CrossRef CAS PubMed.
- M. Ayazi, N. G. Ebrahimi and E. J. Nodoushan, Bacterial adhesion reduction on the surface with a simulated pattern: An insight into extrand model, Int. J. Adhes. Adhes., 2019, 88, 66–73 CrossRef CAS.
- V. G. Shaifali Dhingra, V. Saini, K. Rana, J. Bhattacharyya, T. Loho, S. Ray, A. Bajaj and S. Saha Cytocompatible, Soft and Thick Brush Modified Scaffolds with Prolonged Antibacterial Effect to Mitigate Wound Infections, Biomater. Sci., 2022, 10, 3856–3877 RSC.
- M.-C. Sin, Y.-M. Sun and Y. Chang, Zwitterionic-based stainless steel with well-defined polysulfobetaine brushes for general bioadhesive control, ACS Appl. Mater. Interfaces, 2014, 6(2), 861–873 CrossRef CAS PubMed.
- M. V. Graham,
et al., Development of antifouling surfaces to reduce bacterial attachment, Soft Matter, 2013, 9(27), 6235–6244 RSC.
- C. A. Bunton,
et al., Effect of changes in surfactant structure on micellarly catalyzed spontaneous decarboxylations and phosphate ester hydrolysis, J. Org. Chem., 1975, 40(9), 1321–1327 CrossRef CAS.
- V. L. Frescura,
et al., Effects of sulfobetaine-sodium dodecanoate micelles on deacylation and indicator equilibrium, J. Phys. Chem., 1995, 99(29), 11494–11500 CrossRef CAS.
- A. Joshi, T. Kaur and N. Singh, Exploiting Substrate Cues for Co-Culturing Cells in a Micropattern, Langmuir, 2021, 37(16), 4933–4942 CrossRef CAS PubMed.
- X. Chen,
et al., Multilayer choline phosphate molecule modified surface with enhanced cell adhesion but resistance to protein adsorption, Langmuir, 2017, 33(33), 8295–8301 CrossRef CAS PubMed.
- A. Higuchi,
et al., Preservation of hematopoietic stem and progenitor cells from umbilical cord blood stored in a surface derivatized with polymer nanosegments, Biomacromolecules, 2008, 9(2), 634–639 CrossRef CAS PubMed.
- Y. Chang,
et al., Bioadhesive control of plasma proteins and blood cells from umbilical cord blood onto the interface grafted with zwitterionic polymer brushes, Langmuir, 2012, 28(9), 4309–4317 CrossRef CAS PubMed.
- C.-J. Wu,
et al., Superhydrophilicity and spontaneous spreading on zwitterionic surfaces: carboxybetaine and sulfobetaine, RSC Adv., 2016, 6(30), 24827–24834 RSC.
- B. L. Leigh,
et al., Photopolymerizable zwitterionic polymer patterns control cell adhesion and guide neural growth, Biomacromolecules, 2017, 18(8), 2389–2401 CrossRef CAS PubMed.
- Z. Afzali,
et al., Cell Adhesion and Migration on Thickness Gradient Bilayer Polymer Brush Surfaces: Effects of Properties of Polymeric Materials of the Underlayer, ACS Appl. Mater. Interfaces, 2022, 14(2), 2605–2617 CrossRef CAS PubMed.
- S. Dhingra,
et al., Cytocompatible, Soft and Thick Brush Modified Scaffolds with Prolonged Antibacterial Effect to Mitigate Wound Infections, Biomater. Sci., 2022 10.1039/D2BM00245K.
- Y. Inoue, Y. Onodera and K. Ishihara, Initial cell adhesion onto a phospholipid polymer brush surface modified with a terminal cell adhesion peptide, ACS Appl. Mater. Interfaces, 2018, 10(17), 15250–15257 CrossRef CAS PubMed.
- Z. Zhang,
et al., Superlow fouling sulfobetaine and carboxybetaine polymers on glass slides, Langmuir, 2006, 22(24), 10072–10077 CrossRef CAS PubMed.
- R. Huxtable, Physiological actions of taurine, Physiol. Rev., 1992, 72(1), 101–163 CrossRef CAS PubMed.
- J. C. Kim,
et al., Biocompatible characteristics of sulfobetaine-containing brush polymers, Macromol. Res., 2012, 20(7), 746–753 CrossRef CAS.
|
This journal is © The Royal Society of Chemistry 2023 |
Click here to see how this site uses Cookies. View our privacy policy here.