Surface charge and dynamic mechanoelectrical stimuli improves adhesion, proliferation and differentiation of neuron-like cells
Received
9th September 2022
, Accepted 22nd November 2022
First published on 23rd November 2022
Abstract
Neuronal diseases and trauma are among the current major health-care problems. Patients frequently develop an irreversible state of neuronal disfunction that lacks treatment, strongly reducing life quality and expectancy. Novel strategies are thus necessary and tissue engineering research is struggling to provide alternatives to current treatments, making use of biomaterials capable to provide cell supports and active stimuli to develop permissive environments for neural regeneration. As neuronal cells are naturally found in electrical microenvironments, the electrically active materials can pave the way for new and effective neuroregenerative therapies. In this work the influence of piezoelectric poly(vinylidene fluoride) with different surface charges and dynamic mechanoelectrical stimuli on neuron-like cells adhesion, proliferation and differentiation was addressed. It is successfully demonstrated that both surface charge and electrically active dynamic microenvironments can be suitable to improve neuron-like cells adhesion, proliferation, and differentiation. These findings provide new knowledge to develop effective approaches for preclinical applications.
1. Introduction
Neural tissue dysfunction can result from many situations including trauma (e.g., spinal cord injuries and strokes), the development of neurodegenerative disorders (e.g., Parkinson's disease, Alzheimer's disease, and Multiple Sclerosis) or tumor resection (e.g., optic nerve meningioma and acoustic neuroma).1 All these injuries and disorders can lead to irreversible neural damage, affecting the lives of millions of people worldwide and result in premature death. Although the peripheral nervous system (PNS) has naturally pro-regenerative capacity against small injuries, the central nervous system (CNS) creates a nonpermissive growth environment within the damage site that prevents regeneration from occurring.2 Neural tissue engineering is focusing on overcoming this limitation using biomaterials – scaffolds – to recreate permissive microenvironments to allow for tissue regeneration. The selection of the specific biomaterials is critical, as it is intended to induce a pro-regenerative microenvironment capable of mimicking the native cell milieu with essential sets of biological, morphological, chemical, and physical cues.3 Excitatory cells such as neurons are naturally found in electrical and mechanotransducive microenvironments4,5 and may benefit from the usage of piezoelectric biomaterials.6 When subjected to a mechanical stimulus piezoelectric materials can generate transient surface charges,7 or in the presence of an external electrical field can respond with a mechanical deformation.8 These features make piezoelectric materials suitable for the delivery of electrical and mechanical stimuli to cells, especially those who have piezoelectricity as an intrinsic property,9 such as bone,10–12 tendon13 or skin,14,15 or those who are within or take part of electrical and/or mechanoelectrical environments, such as muscle16,17 and neurons.3,18,19 Electrical stimulation is a method that has been proposed for different neurological and psychiatric disorders, being increasingly investigated for new strategies in the scope of neural repair. It is already being used in clinical practices, but essentially to avoid disease progression, side-effects attenuation and rehabilitation, as the use of functional electrical stimulation,20 often relying on highly invasive procedures as the case of deep brain stimulation.21 Efforts are being carried out in the application of electroactive materials for less invasive approaches in neural repair.
When considering piezoelectric polymers, poly(vinylidene fluoride) (PVDF) and co-polymers stands out by their high piezoelectric coefficient (d33). Semicrystalline PVDF can be obtained into five crystalline phases, known as α, β, γ, ε, and δ, depending on the processing conditions, being the non-polar α-phase the most commonly obtained when processed from the melt and the polar β-phase the most electroactive one.22 Although β-PVDF possess piezoelectric properties, the dipole moments within the material are typically randomly oriented after processing. Thus, it is necessary to submit the samples to a polarization process through the application of an electric field that will align the dipole moments along the thickness direction. This process will generate positive and negative average surface charges on both sides of the sample, respectively, optimizing the piezoelectric response of the material.17 β-PVDF has been reported to promote neurite extension and to enhance proliferation and differentiation of neurons and stem cells under static conditions – that is without dynamic piezoelectric stimulation23,24 – and to induce neuron-like cells differentiation under dynamic conditions by ultrasound stimulation.3,25
The present work investigates both the influence of surface charge type and piezoelectric stimulation of β-PVDF films in the adhesion, proliferation and differentiation of neuron-like SH-SY5Y. Poled β-PVDF is characterized by a high d33 coefficients, up to 34 pC N−1 with a dielectric constant up to 12.22,26 The poling process does not affect the degree of crystallinity of β-PVDF films27 or the mean surface roughness.28,29 However, the poling state leads to increased materials surface energy, presenting lower water contact angles compared to non-poled samples30 (Fig. 1b). Here, SH-SY5Y cells were cultured on non-poled β-PVDF (PVDF NP) and on poled β-PVDF films with positive and negatively charged surfaces (PVDF+ and PVDF−, respectively). The effect of surface charge on cell response was evaluated under standard static conditions, and the piezoelectric effect of the poled samples was evaluated under dynamic culture conditions within a mechanical bioreactor responsible to deliver a mechanical stimulus to poled β-PVDF films to promote the generation of electrical surface variations in the films through the piezoelectric response (Fig. 1). PVDF NP was used to isolate the effects of surface charge and piezoelectric stimulation.
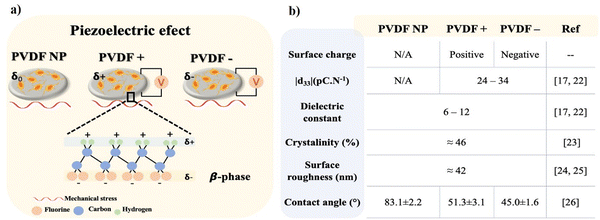 |
| Fig. 1 (a) Piezoelectric PVDF films in the β-phase with average none, positive and negative surface charge and (b) most relevant propertied of β-phase PVDF films. | |
2. Material and methods
β-Phase PVDF films with different surface charges (none ‘PVDF NP’, positive ‘PVDF+’ and negative ‘PVDF−’), with a thickness of 110 μm were obtained by solvent casting according to.16,31 The materials are characterized by a d33 response of |24| pC N−1 for poled films, measured by a d33-meter (model 8000, APC Int Ltd) and a dielectric constant of 12. All materials present a similar degree of crystallinity, surface roughness, and a hydrophilic behavior (Fig. 1b).
SH-SY5Y neuroblastoma cells were purchased from Sigma-Aldrich. For each adhesion, proliferation and differentiation experiments, six replicates of the different PVDF films were cut into discs and sterilized under ultraviolet (UV) light for 30 min on each side before being rinsed twice with phosphate buffer saline (PBS) 1×. Discs with 13 mm diameter were cut and placed on 24-well culture plates for adhesion and differentiation experiments, and 7 mm diameter discs were cut and placed on 48-well culture plates to for proliferation.
2.1 Cell culture
SH-SY5Y neuroblastoma cells were grown in Dulbecco's Modified Eagle's Medium/Nutrient Mixture F-12 Ham (DMEM/Ham's F-12 (1
:
1), Merck), supplemented with 10% of fetal bovine serum (FBS, Biochrom) and 1% of penicillin/streptomycin (P/S, Biochrom). Cells were seeded in 75 cm2 culture flasks at 37 °C under humidified air and a 5% CO2 atmosphere. Grown medium was changed every other day and when cells reached around 60–70% confluence were detached with 0.05% trypsin-EDTA (Biochrom).
Cell suspensions were prepared to seed on top of each film a drop of 150 μL containing 1 × 104 cells for adhesion, a drop of 35 μL containing 1.5 × 104 cells for proliferation, and a drop of 150 μL with 2.5 × 104 cells for differentiation experiments. Plates were incubated for 40 min for cells to adhere and then the wells were supplemented with growth medium. For all assays, dynamic cultures plates were placed in a custom-made mechanical bioreactor with a vertical vibration module,32 previously placed in an CO2 incubator (Panasonic), with a 1 Hz mechanical stimulation, leading to voltage amplitude variations between −20 μV and 100 μV at the surface of the PVDF poled films.
To investigate the influence of PVDF surface charge and the dynamic piezoelectric stimulation on the cell adhesion process, cells were incubated for 3 h before analysis (Fig. 2a).
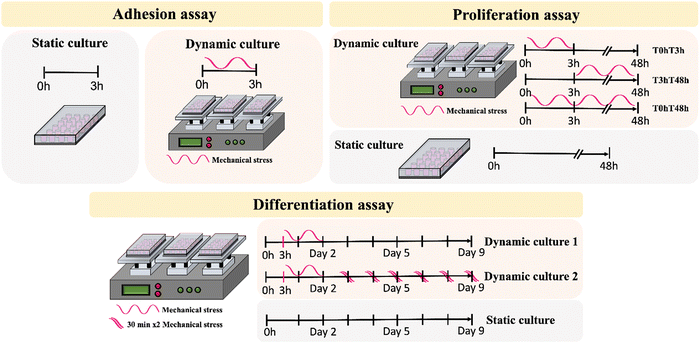 |
| Fig. 2 Schematic representation of cell adhesion, proliferation, and differentiation assay under static and dynamic conditions. | |
For SH-SY5Y proliferation, the piezoelectric effect of the samples with different surface charge was evaluated under mechanical stimulus at different time ranges (Fig. 2b). Different timepoints were selected to assess the effect of the given stimulus on cell adhesion (0 h to 3 h – defined as T0hT3h), after cell adhesion (3 h to 48 h – defined as T3hT48h) and in a continuous stimulus throughout the assay (0 h to 48 h – defined as T0hT48h). Cultures under static conditions were performed in parallel for functional comparison.
Differentiation assays were also carried out under static and dynamic culture conditions. Dynamic conditions were determined following proliferation results, to select the best stimuli for the first 48 h, before initiating differentiation. With this, the selected time interval for the initial stimuli was T3hT48h, after which the growth medium was replaced by differentiation medium, containing 1% P/S, 1% FBS and 10 μM retinoic acid (RA). Two dynamic culture conditions were applied: dynamic1 – cells were exposed to an initial T3hT48h stimuli and remained in static conditions until the end of the assay; and dynamic2 – cells were exposed to a T3hT48h initial stimulus followed by 2 × 30 min stimuli per day, until the end of the assay (Fig. 2c). Medium was replaced every other day until sample collection.
2.2 Cell culture characterization
2.2.1 Immunofluorescence imaging.
Before staining, culture media was removed and cells were fixed with 4% formaldehyde for 10 min at 37 °C. For adhesion assay, cells seeded on the substrates were permeabilized with 0.1% Triton X-100 in PBS 1× and stained with anti-vinculin–FITC antibody (Sigma-Aldrich) in a 1
:
50 PBS 1× solution for 3 h. For proliferation assessment, cellular cytoskeleton was stained for 45 min with tetramethylrhodamine (TRITC, Sigma-Aldrich) at a 1
:
200 ratio in PBS 1×. To evaluate differentiation, cells were blocked in blocking buffer, consisting of 1% bovine serum albumin (BSA), 5% goat serum, 0,2% Triton X-100 in PBS 1×, during 1 h at RT. After blocking, cells were incubated with Anti-Tubulin β-III, clone Tu-20, Alexa Fluor®488 conjugated antibody (Milipore), at a 1
:
250 concentration in PBS 1×, for 2 h at RT. All stainings were performed at room temperature (RT) in the dark and were followed by nucleus staining with 4′,6-diamidino-2-phenylindole (DAPI, Sigma-Aldrich) (1
:
1000× in PBS 1×) for 5 min. Samples were washed two times with PBS 1× and once with distilled water between steps, mounted on glass slides with Fluoroshield (Sigma-Aldrich) and visualized with an Olympus Bx51 Fluorescence Microscope.
2.2.2 DNA quantification.
To measure DNA content of SH-SY5Y proliferating cells, a fluorescence-based method was used – CyQUANT® Cell Proliferation Assay Kit (Thermo-fisher scientific), following manufacturer's instructions. After 48 h of cell culture, medium was removed from each well and washed with PBS 1×. Cells were lysed using 0.1% Triton X-100 (in PBS 1×) and stored at −70 °C. After thawing, cell lysates were placed in a 96-well plate containing a green fluorescent dye (CyQuant® GR dye) that exhibits strong fluorescent enhancement when bound to cellular nucleic acids. Fluorescence signals (excitation 480 nm, emission 520 nm) were directly measured using to a fluorescence microplate reader (Biotech Synergy HT, Winooski, VT). A standard curve was created according to the manufacturer's protocol ranging 0 to 800 ng mL−1 DNA. Statistical analysis was carried out with two-way ANOVA, using Graphpad Prism 9, with p values <0.05 considered to be statistically significant.
2.2.3 Cells quantification.
For the measurements of cells aspect ratio, neurite length and counting tubulin expressing cells, the Fiji software from ImageJ was used. For all quantifications, about 200 cells were analyzed for each condition. All results are presented as mean ± standard error of mean (SEM) and statistical analysis was evaluated with two-way ANOVA, using Graphpad Prism 9, with p values <0.05 considered to be statistically significant.
2.2.4 Phase-contrast optical microscopy.
During differentiation assays, cells were visualized by phase-contrast microscopy at day 2, before exchange of the growth medium by differentiation medium, and at day 9 (end of the assay), to observe cell morphology and neurite extension before and after differentiation. A DMI 3000B Leica Inverted Microscope was used for analysis.
3. Results
3.1 Cell adhesion
Cell-scaffold adhesion is a complex dynamic series of events that involves physical interactions, chemical binding and biological signaling processes that modulate cell proliferation and differentiation.33 To assess cell-material interactions on the different samples, the cellular sensing machinery, i.e. focal adhesion points, were investigated through the visualization of the distribution and recruitment of vinculin after 3 h of contact with PVDF samples, under static and dynamic conditions (Fig. 3a). Vinculin is a ubiquitously cytoskeletal protein expressed during the focal adhesion complex formation. This dynamic complex is responsible for the recruitment of signaling proteins involved in the cellular adhesion process and is required for cellular response to environmental stimuli.34,35Fig. 3b depicts a schematic illustration of cell morphology transformation during the adhesion process.
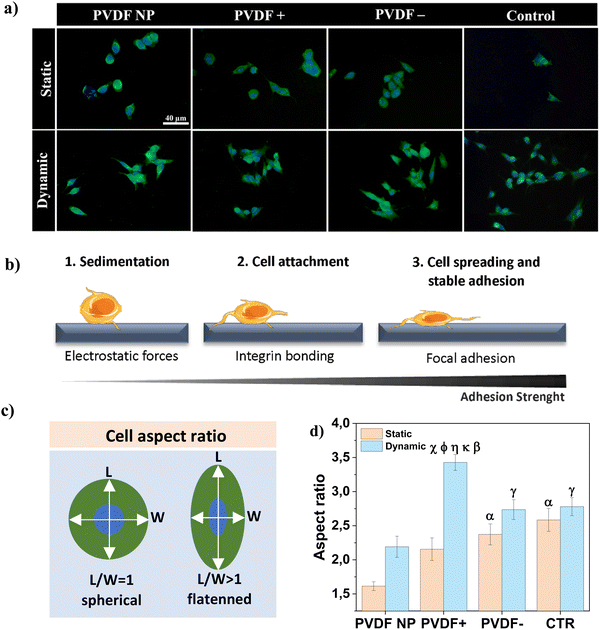 |
| Fig. 3 (a) Representative immunofluorescence images of vinculin expressing SH-SY5Y cells after 3 h of contact with the substrates under static and dynamic conditions. Vinculin stained with anti-vinculin–FITC antibody (green) and nucleus stained with DAPI (blue). Scale bar (40 μm) is valid for all images; (b) schematic representation of cell morphology evolution and adhesion strength variation during cellular adhesion process; (c) schematic representation of cell aspect ratio determination (length versus width); and (d) average cell aspect ratio, with results presented as mean ± SEM. Statistical analysis with two-way ANOVA: αp < 0.05 (static PVDF NP), χp < 0.0001 (static PVDF+), βp < 0.0001 (static CTR). ϕp < 0.0001 (dynamic PVDF NP), γp < 0.05 (dynamic PVDF NP), ηp < 0.05 (dynamic PVDF−), κp < 0.05 (dynamic CTR). | |
Under static conditions, cells cultured on PVDF NP presented a spherical shape and vinculin was expressed tightly around the nucleus (Fig. 3a), suggesting that cells were in earlier stages of adherence with a weak adhesion strength (Fig. 3b1).36 For PVDF+ and PVDF− substrates, some cells retained the spherical form whereas others started to elongate (Fig. 3b2), with vinculin expressed in border regions around the edges of the cell membrane. For dynamic conditions, cells displayed a flattened morphology, which is typical of more advanced stages of adherence (Fig. 3b3), with vinculin expressed in larger areas following cell elongation. Cells cultured on a regular polystyrene well-plate (control), express more vinculin around the nucleus, when compared with poled PVDF samples, for both static and dynamic conditions. However, their shape is not as spherical as on PVDF NP. To validate these results/observations, the cellular aspect ratio was determined, as presented in Fig. 3c, to quantify cellular elongation variation as shown in Fig. 3d.
Regarding the effect of surface charge, cells cultured under static conditions on charged PVDF films (PVDF+ and PVDF−) displayed significantly higher flattening/elongation compared to cells cultured on PVDF NP. It has been reported that electrostatic interactions between cells and charged surfaces can facilitate cell-surface sedimentation, accelerating the adhesion process by lowering the energy barrier for cell adhesion.33,37 This energy barrier was theoretically proposed by Bell et al. and arises from unspecific forces competing with attraction forces between cell surface receptors and ECM ligands.38 Hong et al. experimentally supported this energy barrier concept,33 and our work supports this concept as well. Regarding dynamic conditions, where the mechanical stimulation was applied to induce a dynamic surface charge variation through the piezoelectric response, cells cultured on PVDF− presented significantly higher elongation compared to PVDF NP, and PVDF+ compared to all other substrates. As supported by the vinculin staining results, electrical stimuli given by poled samples, in particular by PVDF+, appeared to induce cellular elongation, which suggests higher adhesion and advanced stages of adherence, compared to static conditions. Cells cultured in the absence of surface charges, both on PVDF NP and on the control, do not present significant differences between static and dynamic conditions, which indicates no direct influence on cells adhesion of the mechanical stimulus alone, without being accompanied by surface charge variation.
3.2 Cell proliferation
SH-SY5Y cells were seeded on PVDF NP, PVDF+, PVDF− and control and exposed to static and dynamic culture conditions during 48 h to assess the effect of the materials surface charge and the different time of stimuli on cellular proliferation. Cells were exposed to bioreactor stimulus in the first 3 hours (T0hT3h), after that (T3hT48h), and throughout the assay (T0hT48h). These times are selected in order to evaluate the effect of the stimuli on: initial cell attachment; cell spreading and adhesion; and in all the events, respectively. DNA content was measured to determine cellular proliferation after 48 h on culture (Fig. 4).
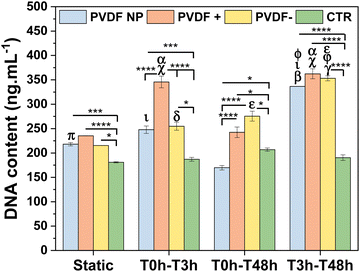 |
| Fig. 4 DNA content of SH-SY5Y cells in contact with PVDF NP, PVDF+ and PVDF−, determined by cell proliferation assay, under static and dynamic conditions. Statistical analysis with two-way ANOVA test: *p < 0.05, ***p < 0.0005, ****p < 0.0001, βp < 0.0001 (static PVDF NP), χp < 0.0001 (static PVDF+), δp < 0.05 (static PVDF−), εp < 0.0001 (static PVDF−), ϕp < 0.0001 (T0hT3h PVDF NP), γp < 0.0001 (T0hT3h PVDF−), πp < 0.05 (T0hT48h PVDF NP), ιp < 0.0001 (T0hT48h PVDF NP), αp < 0.0001 (T0hT48h PVDF+), φp < 0.0001 (T0hT48h PVDF−). | |
The results showed that cells proliferated similarly on all PVDF scaffolds under static conditions, with PVDF+ showing slightly higher values of cell proliferation, although non-significant except when compared to control. PVDF− and PVDF NP also present significant higher proliferation values when compared to control, indicating that cells proliferation was enhanced when cultured on PVDF surfaces. When scaffolds were exposed to bioreactor stimulus during the first 3 h (T0hT3h), cell amounts increase, with poled samples significantly enhancing cell proliferation compared to static conditions. In fact, PVDF+ films significantly improved cellular proliferation not only when compared to static culture, but also when compared to all other substrates under the same T0hT3h stimulus, confirming the effect of positive surface charges. T0hT48h stimulation, on the other hand, did not appear to have a positive effect on SH-SY5Y proliferation, except for PVDF− where cells proliferate more than without any stimulus, but still less than with T3hT48h stimulation. In fact, when PVDF scaffolds were subjected to mechanical stimulation between 3 h to 48 h (T3hT48h), cell proliferation was significantly increased on all substrates. This finding suggests that when the stimulus was applied after the firsts 3 h, that is after the adhesion initiation, the initial surface charge effect was being masked by the influence of the dynamic surface charge variation stimulus provided by the piezoelectric scaffolds. This was applicable for PVDF NP, that under dynamic stimulus can influence cellular behavior. Even though PVDF NP has a low macroscopic electroactive response, cells presented higher proliferation in T3hT48h dynamic conditions than in static culture, similar to poled surfaces. This is explained by the inherent piezoelectricity of PVDF NP and the electroactive response at the microscale, given by the β-phase conformation. Although PVDF NP is not poled and thus dipoles are not uniformly oriented in one direction, PVDF NP is characterized by piezoelectric domains that can be sensed by cells at the focal adhesion level, influencing cellular proliferation.22 These results suggest that, when the stimulus was applied from 3 h to 48 h, cell proliferation was improved by the dynamic surface charge variation (electrical stimulus) influence. It is important to highlight that cells cultured on conventional well-plate surfaces have the same behavior for all conditions, with no significant variation among them, indicating that the mechanical stimulus given by the bioreactor alone does not have any influence on cells proliferation, proving that all effects related to enhanced cell response are due to the electrical potential variation induced on the PVDF surfaces.
Cells morphology was determined by immunofluorescence microscopy and representative micrographs are shown in Fig. 5a, with respective measured neurite lengths in Fig. 5b.
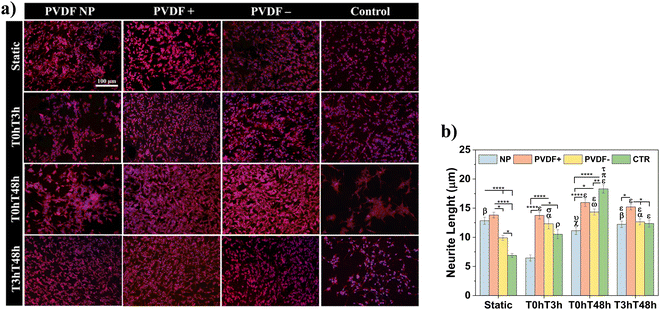 |
| Fig. 5 (a) Representative micrographs of proliferative SH-SY5Y cells. Cell cytoskeleton was stained with TRITC (red) and nucleus with DAPI (blue). Scale bar of 100 μm is representative of all images. (b) Average neurite length of SH-SY5Y cells. Statistical analysis with two-away ANOVA: *p < 0.05, **p < 0.005, ****p < 0.0001; αp < 0.05 (static PVDF−), ωp < 0.0001 (static PVDF−), ρp < 0.05 (static CTR), χp < 0.005 (static CTR), σp < 0.0005 (static CTR), εp < 0.0001 (static CTR), βp < 0.0001 (T0hT3h PVDF NP), πp < 0.05 (T0hT3h CTR), υp < 0.005 (T0hT3h PVDF NP), τp < 0.0001 (T3hT48h CTR). | |
As proposed by cell proliferation measurements, all PVDF scaffolds under static conditions presented similar number of cells, uniformly distributed on all substrate and with small neurite lengths, ranging from 10 ± 1 to 14 ± 1 μm, even larger than neurites present on the control substrate (6 ± 1 μm). For the different dynamic stimuli, PVDF NP and control showed a smaller number of cells when compared to poled scaffolds, for T0hT3h and T0hT48h stimuli in PVDF NP and for T0hT48h and T3hT48h in the control surface. With T0hT3h stimulus, cells cultured on PVDF NP presented considerably smaller neurite lengths, with an average of 6 ± 1 μm. For T3hT48h, all materials presented uniformly distributed cells, with neurites ranging from 12 ± 1 to 15 ± 1 μm. Cells presented longer neurite for both static and dynamic conditions when cultured on PVDF+, ranging from 14 ± 1 to 16 ± 1 μm, confirming the relevant effect of the dynamic surface charge stimulation. Cells cultured on control surfaces shown significantly longer neurites at T0hT48h dynamic condition, which is explained by the fact that cells are clustering and developing longer processes, probably to be able to communicate.
3.3 Cell differentiation
The elongation of neuronal processes, as axons and dendrites, characterizes neuronal differentiation. The interaction of external cues and intracellular mechanisms are involved in the formation of these specialized cytoplasmic domains known as neurites. Extracellular matrix components, cell adhesion molecules and soluble factors as nerve growth factors (NGF), can modulate the direction and extension of neurite development as external cues.39 External electrical stimulation has also been shown to alter the direction and rate of neurite extension in neurons cultured within electric fields. The mechanism by which electrical stimulation influences neurite outgrowth is still unknown, but literature suggests that electrical cues can influence voltage-sensitive ion channels, modulating calcium influx, which affects growth cone motility.40
Phase-contrast microscopy was used to visualize cell morphology and quantify neurite extension under static and dynamic conditions, and images were obtained before and after RA treatment (Fig. 6a). Neurite length measurements were obtained to determine the effect of different surface charges and dynamic electric stimulation (surface charge variation) on neurite extension, an overall indicator of SH-SY5Y differentiation41 (Fig. 6b).
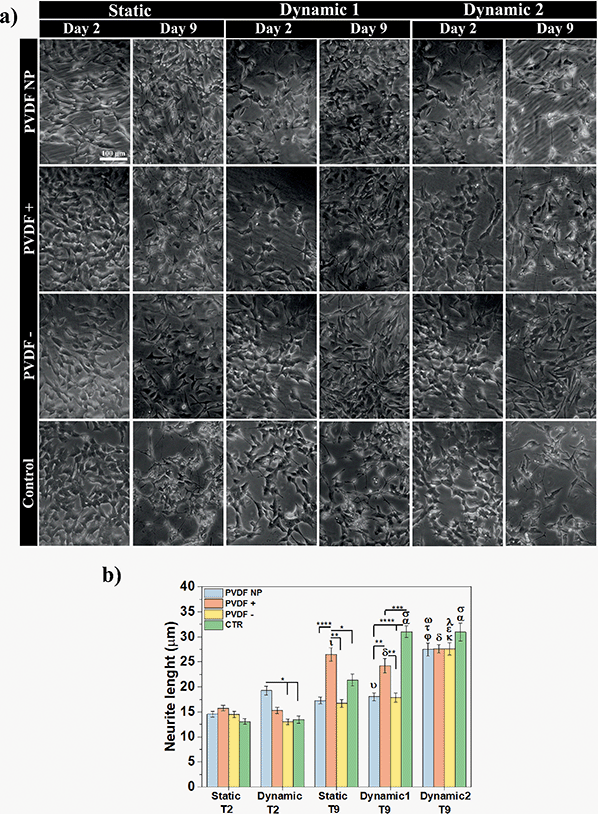 |
| Fig. 6 (a) Phase-contrast optical microscopy visualization of SH-SY5Y cells cultured on PVDF NP, PVDF+, PVDF− and control, under static, dynamic1 and dynamic2 conditions. Scale bar of 100 μm is representative for all images. (b) Neurite lengths of SH-SY5Y cells on day 2 and day 9 of differentiation assay. Statistical analysis with two-way ANOVA: *p < 0.05, **p < 0.005, ***p < 0.0005, ****p < 0.0001; αp < 0.0001 (dynamicT2 CTR), σp < 0.0001 (staticT9 CTR),υp < 0.0001 (dynamicT2 PVDF NP), φp < 0.0001 (staticT9 PVDF NP), τp < 0.0001 (dynamic1T9 PVDF NP), αp < 0.0001 (dynamic1T9 PVDF−), κp < 0.0001 (dynamicT2 PVDF−), εp < 0.0001 (staticT9 PVDF−), λp < 0.0001 (dynamic1T9 PVDF−), ιp < 0.0001 (staticT2 PVDF+), δp < 0.0001 (dynamicT2 PVDF+). | |
Phase-contrast images at day 2 showed no morphological differences in SH-SY5Y cells across all substrates and culture conditions. Cells presented an undifferentiated phenotype with small neurite lengths for all substrates (from 13 ± 1 to 16 ± 1 μm), except for PVDF NP under dynamic culture that supported longer neurites (19 ± 1 μm), compared to the samples on charged surfaces and control at the same condition. After 5 days of RA exposure (day 9 of assay), the neurites lengths significantly increased, ranging from 17 ± 1 to 31 ± 1 μm, indicating differentiated SH-SY5Y cells. Considering the effect of the surface charge, positive net charges appeared to enhance neurite length after RA treatment, independently on the culture conditions. Cells cultured under dynamic2 conditions showed the highest neurite outgrowth for all scaffolds regardless of charge, ranging between 28 ± 1 and 31 ± 1 μm. This imply that by applying a dynamic electrical stimulus through surface charge variation to the cells throughout the assay, neurite outgrowth is promoted, leading to neuronal differentiation. Under dynamic conditions, neurites on control surfaces once more are longer than those cultured on the other substrates. Instead of showing that cells are effectively differentiating more, microscope images reveal that under dynamic stimuli fewer cells are observed on the control substrate when compared to the ones cultured on PVDF. This can indicate cell detachment and may explain why cells need to stretch longer neurites to communicate. To test all these hypothesis, cells were stained with the neuronal marker Tubulin β-III and visualized through immunofluorescence imaging. Representative images are present at Fig. 7a and to determine the effect of different conditions, cells expressing tubulin were quantified (Fig. 7b).
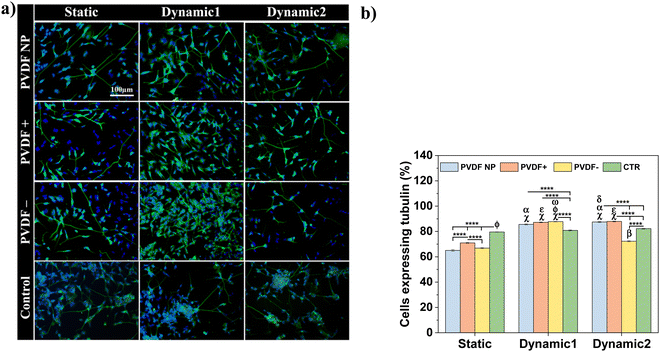 |
| Fig. 7 (a) Representative immunofluorescence micrographs of differentiated SH-SY5Y cells. Tubulin β-III is stained with anti-Tubulin β-III, clone Tu-2, Alexa Fluor®488 conjugated antibody (green) and cell nucleus with DAPI (blue). Scale bar of 100 μm is representative for all images. (b) Percentage cells expressing tubulin. Statistical analysis with two-way ANOVA: ****p < 0.0001, αp < 0.0001 (static PVDF NP), εp < 0.0001 (static PVDF+), βp < 0.0001 (static PVDF−), χp < 0.0001 (static CTR), ωp < 0.0005 (dynamic1 PVDF NP), δp < 0.05 (dynamic1 PVDF NP), ϕp < 0.0001 (dynamic2 PVDF−). | |
After RA treatment, cells cultured on the different substrates expressed tubulin β-III marker, regardless of surface charge type and dynamic stimuli, confirming neuronal identity. The quantification of cells expressing tubulin allowed the quantification and assessment of the electrical stimuli influence on cellular differentiation (Fig. 7b). Both dynamic1 and dynamic2 stimuli improved/induced cell differentiation, with more differentiated cells (72% to 87%) than in the absence of stimuli (65% to 70%), independently of the surface charge type. Although positive charges improved neurite development, when cells were activated electrically, the charge effect was masked. Also, the hypothesis that cells cultured on well-plate surface (control) under mechanical stimuli are not differentiating more comparing to the PVDF samples is corroborated by the β-III tubulin marker, since the cells cultured on the control do not present significant differences between static and dynamic conditions. In fact, cells cultured on stimulated PVDF surfaces, regardless polarization and dynamic stimuli applied (except for dynamic2 PVDF−), express significant more β-III tubulin than the ones cultured on the control, under dynamic or static conditions.
The results suggest that the dynamic2 stimulus, which was given throughout the differentiation process, was the most advantageous, as its improved cell differentiation and also improved neurite development, which is essential for neuronal function.
4. Discussion
This study proves the concept that both surface charge type and dynamic electroactive microenvironments can be used to customize cell adhesion, proliferation, and differentiation. The electrical stimuli provided by the PVDF scaffolds under mechanical stimulation, ranging from −20 μV to 100 μV,42 are found to induce vinculin expression in wider areas and to significantly induce cellular elongation. Furthermore, when the electrical stimulus was combined with positive surface charges (PVDF+), the elongation was improved, which can be related to higher adhesion strength and advanced stages of adhesion.33,36 This effect imply that electrical potentials exposed on PVDF+ generate a higher affinity for cells to adhere to substrates, accelerating the adhesion process even further. DNA content analysis and immunofluorescence assays revealed that positively charged scaffolds (PVDF+) lead to the largest influence on cellular proliferation promotion for almost all the stimuli that cells were subjected to. Furthermore, T3hT48h was the most effective stimulation time interval, with all PVDF substrates significantly improving cell proliferation regardless of charge or polarization state. This indicated that cells take most advantage of a stimulus given after the initial contact with the scaffold (after 3 h), and with this stimulus PVDF does not need to be macroscopically poled as PVDF NP lead to the same effect on cells proliferation as poled scaffolds. It is important to note that comparing PVDF+ and PVDF−, only the type of surface charge can affect the cell behavior, since their degree of crystallinity, surface roughness and wettability are similar (Fig. 1b). Comparing with the sample without surface charge (PVDF NP), only the wettability is higher, however surface chemistry and surface roughness are the same than the poled samples, and for that reason the wettability of the poled samples is determined by the surface charge.43 In this way, it is concluded that the differences found in cell adhesion, proliferation and differentiation are due to the surface charge and the provided electrical stimuli.
Regarding differentiation, neurite extension was significantly improved in dynamic2 conditions, where electrical stimulation was applied throughout the experiment, regardless of surface charge or material polarization state. Tubulin expression was increased similarly for both dynamic1 and dynamic2 stimuli (72% to 87% of the cells), compared to static conditions (65% to 70% of the cells). These results indicate that the stimulus provided throughout the differentiation process was beneficial (dynamic2), as it increased cell differentiation and also neurite extension, which is critical for neuron function.
Electrical stimulation has been extensively studied, but usually requires the use of electrodes, wires, and power supplies. The results of this study are noteworthy for the ability to generate electrical potentials in a non-invasive way, triggered by mechanical stimuli,32 ultrasound44 or magnetically (in magnetically activated piezoelectric materials – magnetoelectric effect17), without the use of devices that could trigger tissue inflammation when implanted, instead relying on wireless sources of stimulation.45 Furthermore, PVDF is an easily tailored polymer, that can be processed in the diverse morphologies and combined with other materials to improve performance and tailoring applications, as it is being studied for other tissues.10,17,46 Although PVDF is being investigated for neural regeneration, only a few studies have focused on the use of its piezoelectric properties.3,25
Overall, these obtained results allow to design advanced electroactive materials-based systems for neural tissue engineering.
5. Conclusions
The present work demonstrates the suitability of electroactive materials for neural tissue regeneration. It is confirmed that both surface charge and electrically active microenvironments can be used to improve and costumize SH-SY5Y adhesion, proliferation, and differentiation.
In particular, upon stimulation, PVDF+ allows to significantly improve cellular adhesion compared to PVDF− and PVDF NP. It is more favorable for proliferation if the electrical stimulus is provided after 3 hours of cells being in contact with the PVDF scaffolds (initial attachment), with the piezoelectric dynamic stimulation overcoming the surface charge effect. Independently of the PVDF surface charge type, the applied electrical stimulus throughout the assay improved neurite extension and differentiation.
However, further studies are required to understand the mechanisms that are implicated by these results. Thus, the investigated electroactive scaffolds have the potential to open up exciting opportunities of investigation and pave the way for future advances in neural regeneration research.
Conflicts of interest
There are no conflicts to declare.
Acknowledgements
This work was supported by national funds through the Fundação para a Ciência e Tecnologia (FCT) and by ERDF through COMPETE2020 – Programa Operacional Competitividade e Internacionalização (POCI) in the framework of the Strategic Programs UID/FIS/04650/2020. TMA thank FCT for the research grant: SFRH/BD/141136/2018 and CR for the contract under the Stimulus of Scientific Employment, Individual Support (CEECIND) – 3rd Edition (2020.04163.CEECIND). Finally, the authors acknowledge funding from the Basque Government Industry Departments under the ELKARTEK program.
References
- D. Kacy Cullen,
et al., Neural tissue engineering for neuroregeneration and biohybridized interface microsystems in vivo (part 2), Crit. Rev. Bioeng., 2011, 39(3), 241–259 Search PubMed.
- J. W. Fawcett and R. A. Asher, The glial scar and central nervous system repair, Brain Res. Bull., 1999, 49(6), 377–391 CrossRef CAS PubMed.
- M. Hoop,
et al., Ultrasound-mediated piezoelectric differentiation of neuron-like PC12 cells on PVDF membranes, Sci. Rep., 2017, 7(1), 4028 CrossRef PubMed.
- J. M. Stukel and R. K. Willits, Mechanotransduction of neural cells through cell-substrate interactions, Tissue Eng., Part B, 2016, 22(3), 173–182 CrossRef CAS.
- H. Yan,
et al., A micropatterned conductive electrospun nanofiber mesh combined with electrical stimulation for synergistically enhancing differentiation of rat neural stem cells, J. Mater. Chem. B, 2020, 8(13), 2673–2688 RSC.
- B. C. Heng,
et al., Signaling pathways implicated in enhanced stem/progenitor cell differentiation on electroactive scaffolds, Smart Mater. Med., 2022, 3, 4–11 CrossRef.
- J. Curie and P. Curie, Développement par compression de l'électricité polaire dans les cristaux hémièdres à faces inclinées, Bull. Mineral., 1880, 3(4), 90–93 Search PubMed.
- G. Lippmann, Principe de la conservation de l'électricité, ou second principe de la théorie des phénomènes électriques, J. Phys. Theor. Appl., 1881, 10(1), 381–394 CrossRef.
- C. Ribeiro,
et al., Piezoelectric polymers as biomaterials for tissue engineering applications, Colloids Surf., B, 2015, 136, 46–55 CrossRef CAS PubMed.
- T. Marques-Almeida,
et al., Patterned piezoelectric scaffolds for osteogenic differentiation, Int. J. Mol. Sci., 2020, 21(21), 1–8 Search PubMed.
- R. Sobreiro-Almeida,
et al., Human mesenchymal stem cells growth and osteogenic differentiation on piezoelectric poly(Vinylidene fluoride) microsphere substrates, Int. J. Mol. Sci., 2017, 18(11), 2391 CrossRef PubMed.
- T. Zheng,
et al., Mimicking the electrophysiological microenvironment of bone tissue using electroactive materials to promote its regeneration, J. Mater. Chem. B, 2020, 8(45), 10221–10256 RSC.
- M. A. Fernandez-Yague,
et al., A Self-Powered Piezo-Bioelectric Device Regulates Tendon Repair-Associated Signaling Pathways through Modulation of Mechanosensitive Ion Channels, Adv. Mater., 2021, 33(40), 2008788 CrossRef CAS PubMed.
- H. F. Guo,
et al., Piezoelectric PU/PVDF electrospun scaffolds for wound healing applications, Colloids Surf., B, 2012, 96, 29–36 CrossRef CAS PubMed.
- A. Sultana,
et al., Human skin interactive self-powered wearable piezoelectric bio-e-skin by electrospun poly-l-lactic acid nanofibers for non-invasive physiological signal monitoring, J. Mater. Chem. B, 2017, 5(35), 7352–7359 RSC.
- S. Ribeiro,
et al., Electroactive biomaterial surface engineering effects on muscle cells differentiation, Mater. Sci. Eng., C, 2018, 92, 868–874 CrossRef CAS PubMed.
- S. Ribeiro,
et al., Magnetically Activated Electroactive Microenvironments for Skeletal Muscle Tissue Regeneration, ACS Appl. Bio Mater., 2020, 3(7), 4239–4252 CrossRef CAS PubMed.
- S. Wu,
et al., Aligned fibrous PVDF-TrFE scaffolds with Schwann cells support neurite extension and myelination in vitro, J. Neural Eng., 2018, 15(5), 056010 CrossRef PubMed.
- X. Yao, Y. Qian and C. Fan, Electroactive nanomaterials in the peripheral nerve regeneration, J. Mater. Chem. B, 2021, 9(35), 6958–6972 RSC.
- D. M. Thompson,
et al., Electrical stimuli in the central nervous system microenvironment, Annu. Rev. Biomed. Eng., 2014, 397–430 CrossRef CAS.
- F. M. Weaver,
et al., Bilateral deep brain stimulation vs best medical therapy for patients with advanced parkinson disease: A randomized controlled trial, JAMA, J. Am. Med. Assoc., 2009, 301(1), 63–73 CrossRef CAS PubMed.
- P. Martins, A. C. Lopes and S. Lanceros-Mendez, Electroactive phases of poly(vinylidene fluoride): Determination, processing and applications, Prog. Polym. Sci., 2014, 39(4), 683–706 CrossRef CAS.
- Y. S. Lee, G. Collins and T. Livingston Arinzeh, Neurite extension of primary neurons on electrospun piezoelectric scaffolds., Acta Biomater., 2011, 7(11), 3877–3886 CrossRef CAS PubMed.
- Y. S. Lee and T. L. Arinzeh, The influence of piezoelectric scaffolds on neural differentiation of human neural stem/progenitor cells, Tissue Eng., Part A, 2012, 18(19–20), 2063–2072 CrossRef CAS PubMed.
- G. G. Genchi,
et al., P(VDF-TrFE)/BaTiO3 Nanoparticle Composite Films Mediate Piezoelectric Stimulation and Promote Differentiation of SH-SY5Y Neuroblastoma Cells, Adv. Healthcare Mater., 2016, 5(14), 1808–1820 CrossRef CAS PubMed.
- J. Gomes,
et al., Influence of the β-phase content and degree of crystallinity on the piezo-and ferroelectric properties of poly(vinylidene fluoride), Smart Mater. Struct., 2010, 19(6), 065010 CrossRef.
- V. Sencadas, S. Lanceros-Méndez and J. F. Mano, Characterization of poled and non-poled β-PVDF films using thermal analysis techniques, Thermochim. Acta, 2004, 424(1–2), 201–207 CrossRef CAS.
- C. Ribeiro,
et al., Fibronectin adsorption and cell response on electroactive poly(vinylidene fluoride) films, Biomed. Mater., 2012, 7(3), 035004 CrossRef CAS PubMed.
- J. Serrado Nunes,
et al., Relationship between the microstructure and the microscopic piezoelectric response of the α- And β-phases of poly(vinylidene fluoride), Appl. Phys. A: Mater. Sci. Process., 2009, 95(3), 875–880 CrossRef CAS.
- P. M. Martins,
et al., Effect of poling state and morphology of piezoelectric poly(vinylidene fluoride) membranes for skeletal muscle tissue engineering, RSC Adv., 2013, 3(39), 17938–17944 RSC.
- C. Ribeiro,
et al., Electroactive poly(vinylidene fluoride)-based structures for advanced applications, Nat. Protoc., 2018, 13(4), 681–704 CrossRef CAS PubMed.
- C. Ribeiro,
et al., Dynamic piezoelectric stimulation enhances osteogenic differentiation of human adipose stem cells, J. Biomed. Mater. Res., Part A, 2015, 103(6), 2172–2175 CrossRef CAS PubMed.
- S. Hong,
et al., Real-time analysis of cell-surface adhesive interactions using thickness shear mode resonator, Biomaterials, 2006, 27(34), 5813–5820 CrossRef CAS PubMed.
- C. Zhou,
et al., Compliant substratum modulates vinculin expression in focal adhesion plaques in skeletal cells, Int. J. Oral Sci., 2019, 11(2), 18 CrossRef PubMed.
- C. A. Mullen,
et al., Cell morphology and focal adhesion location alters internal cell stress, J. R. Soc., Interface, 2014, 11(101), 20140885 CrossRef CAS PubMed.
- A. A. Khalili and M. R. Ahmad, A Review of cell adhesion studies for biomedical and biological applications, Int. J. Mol. Sci., 2015, 16(8), 18149–18184 CrossRef CAS PubMed.
- S. Cai,
et al., Recent advance in surface modification for regulating cell adhesion and behaviors, Nanotechnol. Rev., 2020, 9(1), 971–989 CrossRef CAS.
- G. I. Bell, M. Dembo and P. Bongrand, Cell adhesion. Competition between nonspecific repulsion and specific bonding, Biophys. J., 1984, 45(6), 1051–1064 CrossRef CAS PubMed.
- S. Paganoni and A. Ferreira, Neurite extension in central neurons: A novel role for the receptor tyrosine kinases Ror1 and Ror2, J. Cell Sci., 2005, 118(2), 433–446 CrossRef CAS PubMed.
-
I. P. Clements, et al., Chapter II.6.14 - Neuronal Tissue Engineering, in Biomaterials Science, Academic Press, 3rd edn, 2013, pp. 1291–1306 Search PubMed.
- S. Dwane, E. Durack and P. A. Kiely, Optimising parameters for the differentiation of SH-SY5Y cells to study cell adhesion and cell migration, BMC Res. Notes, 2013, 6(1), 366 CrossRef PubMed.
- S. Ribeiro,
et al., Electroactive functional microenvironments from bioactive polymers: A new strategy to address cancer, Biomater. Adv., 2022, 212849 CrossRef CAS PubMed.
- S. Ribeiro,
et al., Surface Charge-Mediated Cell-Surface Interaction on Piezoelectric Materials, ACS Appl. Mater. Interfaces, 2020, 12(1), 191–199 CrossRef CAS PubMed.
- K. Cai,
et al., Improved activity of MC3T3-E1 cells by the exciting piezoelectric BaTiO3/TC4 using low-intensity pulsed ultrasound, Bioact. Mater., 2021, 6(11), 4073–4082 CrossRef CAS PubMed.
- A. Zaszczyńska, A. Gradys and P. Sajkiewicz, Progress in the applications of smart piezoelectric materials for medical devices, Polymers, 2020, 12(11), 1–19 CrossRef PubMed.
- M. M. Fernandes,
et al., Bioinspired Three-Dimensional Magnetoactive Scaffolds for Bone Tissue Engineering, ACS Appl. Mater. Interfaces, 2019, 11(48), 45265–45275 CrossRef CAS PubMed.
|
This journal is © The Royal Society of Chemistry 2023 |
Click here to see how this site uses Cookies. View our privacy policy here.