Biomimetic dual-responsive bioengineered nanotheranostics for intracellular cascade-synthesizing chemo-drugs and efficient oncotherapy†
Received
11th September 2022
, Accepted 29th November 2022
First published on 29th November 2022
Abstract
Intracellular-synthesized chemo-drugs based on the inherent characteristics of the tumor microenvironment (TME) have been extensively applied in oncotherapy. However, combining other therapeutic strategies to convert nontoxic small molecules into toxic small-molecule chemo-drugs in the TME is still a huge challenge. To address this issue, herein we have developed a biomimetic dual-responsive bioengineered nanotheranostics system via the supramolecular co-assembly of the nontoxic small-molecule 1,5-dihydroxynaphthalene (DHN) and small-molecule photosensitizer indocyanine green (ICG) followed by surface cloaking through red blood cell membranes (RBCs) for intracellular cascade-synthesizing chemo-drugs and efficient oncotherapy. Such nanotheranostics with a suitable diameter, core–shell structure, ultrahigh dual-drug payload rate, and excellent stability can efficiently accumulate in tumor regions and then internalize into tumor cells. Under the dual stimulations of near-infrared laser irradiation and acidic lysosomes, the nanotheranostics system exhibited exceptional instability under heat-primed membrane rupture and pH decrease, thereby achieving rapid disassembly and on-demand drug release. Furthermore, the released ICG can efficiently convert 3O2 into 1O2. After that, the generated 1O2 can efficiently oxidize the released nontoxic DHN into the highly toxic chemo-drug juglone, thereby realizing intracellular cascade-synthesizing chemo-drugs and synergistic photodynamic-chemotherapy while reducing detrimental side effects on normal cells or tissues. Overall, it is envisioned that RBC-cloaked nanotheranostics with intracellular cascade-synthesizing chemo-drugs can provide a promising strategy for intracellular chemo-drug synthesis–based oncotherapy.
1. Introduction
As is well-known, although chemotherapy is a primary therapeutic method for oncotherapy in clinics, various chemo-drugs still suffer from severe undesirable side effects owing to their rapid blood/renal clearance, low bioavailability, nonspecific biodistribution, and low tumor accumulation.1,2 In view of this, to minimize the undesirable side effects, various nanotheranostics that can optimize the therapeutic effects and pharmacokinetic indexes of various chemo-drugs have been extensively developed.3 In addition, as the physical environment, celluar metabolism, and biosynthetic intermediates of the tumor microenviroment (TME) are obviously different from those of normal cells or tissues, TME-responsive nanotheranostics have received widespread attention.4 Notably, a large number of studies have pointed out that TME-responsive nanotheranostics can effectively aviod drug leakage during in vivo blood circulation and then unload the drugs via controlled release after reaching the tumor regions, thereby further reducing undesirable side effects of the drugs.5,6 However, the direct introduction of toxic chemo-drugs still results in some non-negligible side effects.7 To address this issue, a promising therapeutic strategy is to convert nontoxic small molecules in various TME-responsive nanotheranostics into toxic small-molecule chemo-drugs in the TME.8,9 In addition, some previous reports have shown that such a strategy will not only exert a therapeutic effect on tumor cells or tissues but also have no toxic side effects on normal cells or tissues.10,11 However, such TME-responsive nanotheranostics only depend on a single chemotherapy, which can hardly exert efficent oncotherapy. Therefore, combining other therapeutic strategies to convert nontoxic small molecules into toxic small-molecule chemo-drugs in the TME is a key topic of future chemotherapy research.8
Recently, to achieve near-infrared (NIR) fluorescence imaging–guided synergistic photodynamic-chemotherapy, a representative non-invasive theranostic strategy was demonstrated involving integrating two or more different theranostic agents into a single nanotheranostics system.12,13 Among many nanotheranostics, vehicle-free nanotheranostics, which are co-assembled from small molecules, have attracted increasing attention in drug delivery and oncotherapy due to their simple construction process, high drug payload rate, and low production cost.3 However, these vehicle-free nanotheranostics co-assembled only via weak interactions still suffer from physiological instability and critical premature drug leakage.14 To address this issue, although our group found and verified that vehicle-free metal–organic nanotheranostics not only showed remarkably enhanced structural integrity and stability but also reduced drug leakage during in vivo blood circulation,15,16 nevertheless, directly exposing such vehicle-free nanotheranostics in the blood circulation system will inevitably suffer from rapid blood clearance due to immune surveillance, which will significantly reduce the delivery efficiency of vehicle-free nanotheranostics to tumor regions.17–19 To ameliorate this conflict between vehicle and vehicle-free nanotheranostics, the addition of trace amounts of various natural biological membranes cloaked on the surface of vehicle-free nanotheranostics to obtain bomimetic nanotheranostics has emerged as a promising alternative.19–21 For example, some reports have pointed out that the red blood cell membranes (RBCs), macrophagocytes, and natural killer cell membrane-cloaked nanotheranostics can effectively prolong the in vivo blood circulation time, reduce macrophage uptake, and enahance tumor accumulation.22 Moreover, once irradiated via an exogenous NIR laser, these natural biological membranes can be ruptured by the sharp thermal disturbance, which will promote rapid disassembly and on-demand drug release.
In view of the above considerations, as shown in Scheme 1, photodynamic therapy and intracellular cascade-synthesizing chemo-drugs were introduced into one theranostic system via co-assembling the nontoxic small-molecule 1,5-dihydroxynaphthalene (DHN) and small-molecule photosensitizer indocyanine green (ICG) into vehicle-free nanotheranostics (named ICG-DHN) by weak interaction-driven supramolecular co-assembly without any surfactants or other auxiliary agents. After that, ICG-DHN was cloaked with trace amounts of RBCs to construct biomimetic nanotheranostics (named RBCs@ICG-DHN). RBCs@ICG-DHN can be efficiently delivered to tumor regions and then internalized into tumor cells due to the excellent pharmacokinetics of its nanostructure and the stealth effect of the RBCs. Once stimulated with NIR laser irradiation and acidic lysosomes, RBCs@ICG-DHN can achieve rapid disassembly and on-demand drug release. Notably, the released ICG can efficiently convert 3O2 into 1O2. After that, the generated 1O2 can efficiently oxidize the released nontoxic DHN into the highly toxic chemo-drug juglone, thereby realizing intracellular cascade-synthesizing chemo-drugs and synergistic photodynamic-chemotherapy while weakening the detrimental side effects on normal cells or tissues. In contrast with traditional modalities, this study provides a reference to explore intracellular chemo-drug synthesis-based oncotherapy.
 |
| Scheme 1 . (A) Photooxidation mechanism of DHN. Schematic illustrations of (B) formation and (C) intracellular cascade-synthesizing drugs and efficient tumor therapy of biomimetic dual-responsive bioengineered RBCs@ICG-DHN. | |
2. Results and discussion
2.1. Construction and characterization of vehicle-free nanotheranostics
Recently, supramolecular co-assembly nanotechnology has garnered much attention in the development of new theranostic delivery nanoplatforms for precise biomedicine.23,24 In our study, the vehicle-free ICG-DHN was constructed by a weak interaction-driven supramolecular co-assembly between the nontoxic hydrophobic DHN and FDA-approved amphiphilic photosensitizer ICG (Scheme 1B). To construct ICG-DHN, the nontoxic hydrophobic DHN and amphiphilic photosensitizer ICG were dissolved in a mixed organic solvent, followed by injecting the organic solvent dropwise into an aqueous solution under stirring. After that, the resultant ICG-DHN could be facilely obtained after stirring and dialysis. As is well-known, a regular morphology, suitable diameter, and small polydispersity index (PDI) are necessary for the further in vivo application of nanotheranostics. In view of this, to obtain the excellent ICG-DHN, a large number of experiments were conducted. As shown in Fig. 1A and B, the diameter, morphology, and PDI of ICG-DHN could be adjusted by altering the mass ratio of ICG and DHN (1
:
1, 2
:
1, and 1
:
2, respectively). It was noteworthy that the transmission electron microscopy (TEM) images intuitively indicated that ICG-DHN had a little core–shell structure with an average diameter of ca. 180 nm under an optimized mass ratio of 1
:
2. In addition, the dynamic light scattering (DLS) data also showed a narrow monodispersion with both a small PDI of 0.181 and a small diameter of ca. 185 nm, which was beneficial for efficiently realizing the EPR effect.
 |
| Fig. 1 Characterization of ICG-DHN and RBCs@ICG-DHN. (A) TEM images and (B) diameter of ICG-DHN. (C) FT-IR and (D) UV-vis spectra of ICG, DHN, ICG/DHN, and ICG-DHN. (E and F) TEM images of RBCs@ICG-DHN. (G) Diameter, (H) UV-vis spectra, and (I) zeta potential of ICG-DHN and RBCs@ICG-DHN. Diameters of (J) ICG-DHN and (K) RBCs@ICG-DHN. Data are presented as mean ± SD (n = 3) and ***p < 0.001. | |
Next, the pivotal formation mechanism of ICG-DHN was simulated using the AutoDock Vina program. As depicted in Scheme 1B, the simulation result indicated that ICG and DHN could be co-assembled into ICG-DHN by weak interactions involving hydrogen bonds, π–π stacking, and hydrophobic interactions, either alone or in combination. This simulation result was further verified by the Fourier-transform infrared (FT-IR), 1H nuclear magnetic resonance (1H NMR), X-ray diffraction (XRD), and ultraviolet–visible (UV-vis) absorption results. As shown in Fig. 1C, the FT-IR spectrum of the ICG/DHN mixture exhibited an additive effect compared with that of ICG or DHN. Close observation revealed that the C–O stretching vibration from DHN at 1600 cm−1 was shifted to a lower wavenumber at 1592 cm−1 in the FT-IR spectrum of ICG-DHN compared with that of DHN.25 In addition, this experimental result was well in line with the 1H NMR results (Fig. S1A, ESI†). Notably, as shown in Fig. 1D, comparing both ICG and the physical mixture of ICG and DHN, the UV-vis peak in ICG-DHN displayed a red-shift from 390 nm and 713 nm to 398 nm and 717 nm, respectively. The above-mentioned experimental phenomenon combined with previous studies well confirmed that the sulfonic acid group of ICG could interact with the C–O group from DHN through hydrogen bonds in the structure formation of ICG-DHN.
Besides, to further explore the distribution state of DHN and ICG in ICG-DHN, X-ray diffraction (XRD) was also performed. As displayed in Fig. S1B (ESI†), the XRD pattern of ICG exhibited a broad peak, indicating that ICG was amorphous. In addition, the XRD pattern of DHN displayed some sharp crystalline peaks, suggesting that DHN had a crystalline form. Of note, the XRD pattern of the physical mixture of ICG and DHN displayed some crystalline peaks, indicating that ICG and DHN as a crystalline form were still retained in the physical mixture of ICG and DHN. By contrast, some crystalline peaks of DHN were covered in the XRD pattern of ICG-DHN, suggesting that DHN could be dispersed in the ICG-DHN matrix. Notably, such a distribution state of DHN and ICG in ICG-DHN would be conducive to enhancing the water solubility of DHN.
2.2. Construction and characterization of biomimetic nanotheranostics
Authoritative research studies have revealed that the membrane components on RBCs play a pivotal role in their functions, such as enhancing their biocompatibility, prolonging circulation lifetime, and reducing macrophage uptake.26–28 In view of these considerations, biomimetic RBCs were cloaked onto ICG-DHN surface via a multiple-step extrusion to obtain the biomimetic dual-responsive bioengineered nanotheranostics system RBCs@ICG-DHN. The TEM images in Fig. 1E and F intuitively showed that the RBCs@ICG-DHN had a classic core–shell structure and the thickness of its outer lipid shell was ca. 20 nm. In addition, Fig. 1G implied that the diameter increased by ca. 20 nm before and after RBCs modification, which was equivalent to a covering of three layers of RBCs lipid bilayers. As expected, close observation found that the characteristic peak of the RBCs could be found in the UV-vis absorption peak of RBCs@ICG-DHN (Fig. 1H), which could be due to the biomimetic cell membrane coated on the surface of ICG-DHN after a series of extrusion operations. Furthermore, the zeta potential of ICG-DHN increased to −32 mV after encapsulation with RBCs (Fig. 1I), which was conducive to improving the physiological stability and prolonging the in vivo blood circulation time. In addition, to further confirm whether there were membrane proteins from RBCs on the surface of RBCs@ICG-DHN, gel electrophoresis was also performed. As shown in Fig. S2 (ESI†), RBCs@ICG-DHN and RBCs almost possessed identical protein components, suggesting that membrane proteins from the RBCs were perfectly retained. In addition, it was found that the encapsulation efficiencies of DHN and ICG were 81.23% and 68.59%, respectively. The drug payload rates of DHN and ICG were 54.15% and 22.86%, respectively. In other words, our experimental results convincingly confirmed the successful construction of RBCs@ICG-DHN.
2.3.
In vitro stability
As is well-known, ICG suffers from instability and selfaggregation in aqueous solution, which will seriously compromise its theranositic effect. To overcome these drawbacks, a promising strategy is to construct ICG-based nanotheranostics.29 In addition, a large number of reports have revealed that physiological stability is one of critical indexes for the further clinical application of nanotheranostics.18,30 As shown in Fig. S3 (ESI†), no apparent precipitation could be found in ICG-DHN after incubation with PBS for 24 h. On the contrary, a large amount of precipitation emerged in the DHN or ICG/DHN mixture. This experimental result indicated that the stability of ICG-DHN in PBS (pH 7.4) was greatly enhanced due to the hydrogen bond interactions between DHN and ICG. In addition, the introduction of both weak interactions (including hydrogen bonds, π–π stacking, salt bridges, hydrophobic interactions, π-cations/anions, and electrostatic interactions, singly or in combination) and biomimetic RBCs could improve the structural integrity and physiological stability of biomimetic nanotheranostics during in vivo blood circulation.31 In light of the aforementioned considerations, the diameter, as an estimation parameter of the physiological stability, was determined by DLS at predesigned time intervals. As shown in Fig. 1J, the diameters of ICG-DHN were almost unchanged when incubated in PBS, water, and Dulbecco's modified Eagle's medium (DMEM) for 120 h. As displayed in Fig. S4 (ESI†), the diameters of ICG-DHN sharply increased in DMEM containing 10% fetal bovine serum (FBS). In sharp contrast, the diameters of RBCs@ICG-DHN were almost unaltered when cultured in DMEM containing 10% FBS for 120 h (Fig. 1K). Hence, these experimental results demonstrated that RBCs@ICG-DHN indeed had excellent physiological stability in PBS (pH 7.4), DMEM, and DMEM containing 10% FBS, suggesting that RBCs@ICG-DHN might still retain an intact nanostructure during in vivo blood circulation, and meanwhile could provide a prerequisite for the ERR effect and tumor enrichment.15 Moreover, these results also confirmed that the hydrogen bond-driven supramolecular recognition between DHN and ICG played a critical role in ICG-DHN formation, while RBCs efficiently enhanced the dispersity, integrity, and physiological stability of the RBCs@ICG-DHN.
Next, to further evidence the photostability of RBCs@ICG-DHN, both the fluorescence intensity and UV-vis absorption were determined at different time points. As could be seen from Fig. S5 (ESI†), the fluorescence intensity of RBCs@ICG-DHN was higher than that of ICG or ICG-DHN. Close observation found that both the fluorescence intensity and UV-vis absorption of ICG remarkably decreased to 60% and 70% of the initial value (Fig. 2A and B), respectively. In sharp contrast, RBCs@ICG-DHN merely showed a slow attenuation, and meanwhile maintained the original 80% and 70%, respectively. Besides, in contrast with Fig. S6 (ESI†) and Fig. 2A and B, it could be found that the photostability of RBCs@ICG-DHN was better than that of ICG-DHN, indicating that ICG stably loaded in RBCs could efficiently improve the photostability. On the basis of the experimental analysis, it could be deduced that RBCs effectively protected the double bonds on the conjugate chain of ICG from being destroyed during in vivo blood circulation, which was well in line with the previous theories proposed by other groups or our group that wrapping ICG in biomimetic RBCs could enormously raise its photostability and physiological stability.18,29 Therefore, all the aforementioned in vitro stability results and theories convincingly confirmed that RBCs@ICG-DHN had excellent stability for intracellular cascade-synthesizing chemo-drugs and synergistic photodynamic-chemotherapy.
 |
| Fig. 2
In vitro photostability, photodynamic effect, photooxidation, and on-demand drug release of RBCs@ICG-DHN. Change in (A) fluorescence intensity and (B) UV-vis absorption of ICG and RBCs@ICG-DHN in PBS for 120 h. (C) SOSG fluorescence intensity of PBS, ICG, and RBCs@ICG-DHN after 808 nm laser irradiation (100 mW cm−2) for 5 min. (D) SOSG fluorescence spectra of RBCs@ICG-DHN after 808 nm laser irradiation (100 mW cm−2) at the designated time points. (E) UV-vis absorption spectra of DHN in PBS with RBCs@ICG-DHN after 808 nm laser irradiation (100 mW cm−2) for 30 min. (F) Cumulative release of DHN from ICG-DHN and RBCs@ICG-DHN at pH 7.4, 6.5, and 5.0. (G) Cumulative release of DHN from ICG-DHN and RBCs@ICG-DHN at pH 6.5 and 5.0 with 808 nm laser irradiation (100 mW cm−2) for 5 min. (H) TEM images of RBCs@ICG-DHN at pH 6.5 and 5.0 with 808 nm laser irradiation (100 mW cm−2) for 5 min. Data are presented as mean ± SD (n = 3), *p < 0.05, **p < 0.01, and ***p < 0.001. | |
2.4.
In vitro photodynamic effect
ICG as an FDA-approved broad photosensitizer can produce 1O2 under 808 nm laser irradiation to exert a photodynamic effect.32 In order to evaluate the photodynamic effect of RBCs@ICG-DHN, a single oxygen sensor green (SOSG) probe was utilized to detect 1O2 under 808 nm laser irradiation (100 mW cm−2) for 5 min. As shown in Fig. 2C, the amount of 1O2 produced by RBCs@ICG-DHN was greater than that by ICG under the same conditions, which could be due to the fact that both the hydrogen bond-driven supramolecular co-assembly and RBCs decoration significantly improved the photostability of ICG.30,33 In addition, after 808 nm laser irradiation (100 mW cm−2) for 5 min, the production of 1O2 from RBCs@ICG-DHN displayed an obvious time/concentration-dependent increase (Fig. 2D and Fig. S7, ESI†). Therefore, the aforementioned experimental results revealed that the RBCs@ICG-DHN possessed an excellent photodynamic effect.
2.5.
In vitro photooxidation
Previous reports have found that DHN has a high photooxidation selectivity and can be oxidized by 1O2 to the highly toxic chemo-drug juglone.8 In addition, our preliminary experimental results also found that ICG as an exceptional photosensitizer could efficiently catalyze DHN into juglone, thereby achieving the synergistic therapeutic purpose of photodynamic therapy and chemotherapy. In light of the aforementioned considerations, RBCs@ICG-DHN could be employed to catalyze the oxidation of DHN to generate juglone. The in vitro photooxidation of RBCs@ICG-DHN was evaluated by UV-vis absorption. As shown in Fig. 2E, after 808 nm laser irradiation (100 mW cm−2) for 30 min, the UV-vis absorption of DHN at 298 nm gradually decreased while that of juglone increased. Moreover, our calculated results showed that the photooxidation reaction rate of DHN was 30% and the juglone yield could reach 60%. Hence, when RBCs@ICG-DHN was irradiated by 808 nm laser irradiation, ICG could effectively catalyze nontoxic DHN into the highly toxic juglone. Such an in vitro photooxidation process could be expected to achieve intracellular cascade-synthesizing chemo-drugs and synergistic photodynamic-chemotherapy while weakening the detrimental side effects on normal cells or tissues.
2.6.
In vitro drug release
As is well-known, on-demand drug release can achieve a precise regulation of the release process, thereby efficently avoiding premature drug leakage or off-target release during the blood circulation system and allowing a quick unloading through a controlled release behavior once arriving at the tumor regions.12,34 Motivated by the concept of on-demand drug release mediated by stimulus responsiveness, we further verified the in vitro drug release and disassembly of RBCs@ICG-DHN under simulated physiological conditions of normal tissues and blood circulation (pH 7.4), the weakly acidic conditions of the TME (pH 6.5) including (or not) 808 nm laser irradiation, and the acidic conditions of tumor cells (pH 5.0) including (or not) 808 nm laser irradiation. As shown in Fig. 2F and Fig. S8A (ESI†), the DHN and ICG amounts released from RBCs@ICG-DHN at pH 7.4 were only ca. 22% and 26% in 48 h, respectively. This result indirectly verified that RBCs@ICG-DHN might have good stability in the physiological conditions of normal tissues and blood circulation. In addition, the cumulative releases of DHN and ICG from RBCs@ICG-DHN at pH 5.0 were ca. 50% and 49% in 48 h, respectively. By contrast, after 808 nm laser irradiation, the cumulative release of DHN and ICG rapidly increased to ca. 82% and 83% at pH 5.0 in 48 h (Fig. 2G and Fig. S8B, ESI†), respectively. This result could be due to the mature theory in previous reports that biomimetic RBCs on the surface of RBCs@ICG-DHN could be fractured under 808 nm laser irradiation and then the hydrogen bonds between DHN and ICG might be broken at pH 5.0.35,36 Therefore, these experimental phenomena revealed that RBCs@ICG-DHN could achieve disassembly (Fig. 2H) and on-demand drug release under the dual stimuli of internal acidic lysosomes and external NIR laser.18,22
2.7.
In vitro cellular uptake
According to our design idea, the efficient cellular uptake effect of RBCs@ICG-DHN in tumor cells is essential for intracellular cascade-synthesizing chemo-drugs and synergistic photodynamic-chemotherapy.37 In view of this, the celular uptake effect of RBCs@ICG-DHN was explored via visual fluorescence imaging by using its own spontaneous fluorescence of ICG. To compare the cellular uptake effect of ICG, ICG-DHN, and RBCs@ICG-DHN, 4T1 cells were cultured with them for 1 and 4 h, respectively. As shown in Fig. 3A, the fluorescence signals of ICG determined by qualitative confocal laser scanning microscopy (CLSM) indicated that ICG-DHN and RBCs@ICG-DHN were more taken up by 4T1 cells in comparison with ICG. Moreover, the enhanced cellular effects of ICG-DHN and RBCs@ICG-DHN were also verified using a quantitative flow cytometer (Fig. 3B–E). In addition, the mean fluorescence intensity was obviously higher in ICG-DHN and RBCs@ICG-DHN than in the ICG-treated cells at the same time point (p < 0.01) (Fig. 3F and G). Close observation also found that the cellular uptakes of ICG-DHN and RBCs@ICG-DHN in 4T1 cells significantly elevated in a time-dependent method. Therefore, the aforementioned quantitative and qualitative results jointly demonstrated that ICG-DHN and RBCs@ICG-DHN could be effectively taken up through 4T1 cells, which could be due to the introduction of the nanostructure with a suitable diameter.38
 |
| Fig. 3
In vitro cellular uptake and intracellular ROS generation ability. (A) CLSM images of 4T1 cells cultured with ICG, ICG-DHN, and RBCs@ICG-DHN at equivalent ICG concentrations for 1 and 4 h. Flow cytometric measurement of 4T1 cells cultured with ICG, ICG-DHN, and RBCs@ICG-DHN at equivalent ICG concentrations for (B) 1 and (C) 4 h. Flow cytometric analysis of 4T1 cells cultured with (D) ICG and (E) RBCs@ICG-DHN at equivalent ICG concentrations for 1 and 4 h. (F) Mean fluorescence intensity of 4T1 cells incubated with ICG, ICG-DHN, and RBCs@ICG-DHN at equivalent ICG concentrations for 1 and 4 h. (G) Mean fluorescence intensity of 4T1 cells incubated with ICG and RBCs@ICG-DHN at equivalent ICG concentrations for 1 and 4 h. (H) CLSM images, (I) flow cytometric analysis, and (J) mean fluorescence intensity of DCFH-DA-stained 4T1 cells after culture for 8 h with PBS (control), ICG, ICG-DHN, and RBCs@ICG-DHN under 808 nm laser irradiation (100 mW cm−2) for 5 min. Data are presented as mean ± SD (n = 3), *p < 0.05, **p < 0.01, and ***p < 0.001. | |
In addtion, as shown in Fig. 3A–G, it should be pointed out that the mean fluorescence signals of RBCs@ICG-DHN group were stronger than those of the ICG-DHN group in 4T1 cells at the same time point. This phenomenon revealed that the cellular uptake effect of the nanostructure could be enhanced due to the large proportion of bilayer lipid membrane from the RBCs on the surface of the nanostructure. Therefore, RBCs@ICG-DHN had an efficient cellular uptake effect on 4T1 cells.
2.8. Intracellular ROS evaluation
For RBCs@ICG-DHN, after 808 nm laser irradiation, the generation profile of the intracellular 1O2 (a kind of ROS) not only reflected its photodynamic effect but also provided a prerequisite for catalyzing nontoxic DHN into the highly toxic chemo-drug juglone. For this purpose, the 2′,7′-dichlorodihydrofluorescein diacetate (DCFH-DA) probe was employed to evaluate the ability of RBCs@ICG-DHN to generate the intracellular 1O2. As shown in Fig. 3H–J, no significant DCFH fluorescence signals could be found in the control group due to the lack of 1O2 in cytoplasm. Notably, after 808 nm laser irradiation for 5 min, the 4T1 cells cultured with ICG showed strong fluorescence signals, verifing that ICG as a kind of photosensitizer could exert a photodynamic effect.20 By contrast, 4T1 cells incubated with both ICG-DHN and RBCs@ICG-DHN showed an enhanced oxidative stress effect. In addition, it was also found that the DCFH fluorescence signals of 4T1 cells were much stronger in RBCs@ICG-DHN than those in ICG-DHN (p < 0.01). Therefore, the aforementioned experimental results revealed that RBCs@ICG-DHN with 808 nm laser irradiation could generate a high abundance of 1O2 in 4T1 cells due to its efficient cellular uptake effect. Therefore, the efficient cellular uptake and photodynamic effect of RBCs@ICG-DHN could be expected to provide a prerequisite for intracellular cascade-synthesizing chemo-drugs and synergistic photodynamic-chemotherapy.
2.9.
In vitro therapeutic effect by synergistic photodynamic-chemotherapy
Motivated by the efficient photooxidation and photodynamic effect of RBCs@ICG-DHN, the in vitro antitumor effect of RBCs@ICG-DHN was further estimated. In the present study, the cytotoxicity of RBCs@ICG-DHN was determined via a 3-[4,5-dimethylth-iazol-2-yl]-2,5-diphenyltetrazolium bromide (MTT) assay. 4T1 cells were cultured with ICG, DHN, ICG-DHN, and RBCs@ICG-DHN for 24 h. Meanwhile, the cytotoxicity of RBCs@ICG-DHN in 4T1 cells was further measured with/without 808 nm laser irradiation. As shown in Fig. 4A and B, in the case with no 808 nm laser irradiation, except for juglone (a highly toxic chemo-drug), all the formulations showed no an obvious killing effect (the cell viability was higher than 90%) with the concentration increase, indicating that ICG-DHN and RBCs@ICG-DHN could be expected to greatly minimize the toxic side effects on normal tissue during in vivo circulation due to ICG and DHN not having a toxic effect.8,39 As displayed in Fig. 4C, in the case with 808 nm laser irradiation, the cytotoxicity of all the formulations showed a significant concentration-dependent increase. Notably, the cytotoxicity of RBCs@ICG-DHN with 808 nm laser irradiation was higher than that of ICG-DHN with 808 nm laser irradiation.
 |
| Fig. 4
In vitro antitumor evaluation. (A) Cell viability of 4T1 cells incubated with ICG, DHN, ICG-DHN, and RBCs@ICG-DHN at equivalent DHN concentrations (2, 5, 10, 20, and 40 μg mL−1) for 24 h. (B) Cell viability of 4T1 cells incubated with juglone at different concentrations (1, 2, 5, 10, and 20 μg mL−1) for 24 h. (C) Cell viability of 4T1 cells incubated with ICG, ICG-DHN, and RBCs@ICG-DHN at equivalent DHN concentrations (2, 5, 10, 20, and 40 μg mL−1) with 808 nm laser irradiation (100 mW cm−2 and 5 min) for 24 h. (D) Calcein-AM (green, live cells) and PI (red, dead cells) costained fluorescence images and (E) flow cytometric analysis with early and late apoptotic cells of 4T1 cell apoptosis treated with ICG, DHN, ICG-DHN, and RBCs@ICG-DHN with or without 808 nm laser irradiation (100 mW cm−2 and 5 min) for 24 h. Data are presented as mean ± SD (n = 3), *p < 0.05, and **p < 0.01. | |
Next, to further verify the in vitro antitumor effect of RBCs@ICG-DHN, both live/dead staining and the Annexin V-FITC/PI assay were also conducted. As shown in Fig. 4D and E, RBCs@ICG-DHN with 808 nm laser irradiation could kill the majority of 4T1 cells compared with the other groups, which was well in line with the aforementioned cytotoxicity experiment. Therefore, these experimental results jointly evidenced that RBCs@ICG-DHN with 808 nm laser irradiation had an outstanding in vitro antitumor effect due to the efficient intracellular drug enrichment, the controllable on-demand drug release behavior, the efficient photooxidation, and the synergistic photodynamic-chemotherapy.
2.10.
In vivo NIR fluorescence self-imaging
As is well-known, accurate drug delivery, efficient tumor accumulation, and reasonable laser irradiation measures play a crucial role in tumor photodynamic therapy.40 In addition, NIR fluorescence self-imaging technology has attracted widespread attention due to low absorption and auto-fluorescence of biological tissues in the NIR range.29 Moreover, NIR fluorescence self-imaging can provide precise information for investigating the in vivo behaviors of nanotheranostics and for guiding the therapeutic effect to optimize therapy.41 Based on the above considerations, to further explore the tumor accumulation situation and timing of RBCs@ICG-DHN, in vivo NIR fluorescence self-imaging was performed on 4T1 tumor-bearing nude mice at predesigned time intervals after intravenous injection. As shown in Fig. 5A and B, the fluorescence signals in the ICG group show it was quickly metabolized and then eliminated from the blood circulation system after intravenous injection for 6 h due to the small molecular ICG binding to plasma proteins. In addition, close observation also found that the fluorescence signals in the ICG-DHN group or RBCs@ICG-DHN group showed their obvious accumulation at tumor regions. In contrast, the fluorescence signals of the tumor regions were much stronger in the RBCs@ICG-DHN group compared with those in the ICG-DHN group. Meanwhile, the fluorescence signals in the RBCs@ICG-DHN group reached the maximum peak at 12 h and were maintained in vivo for a long time, which might be associated with the introduction of RBCs (RBCs on the surface of the nanostructure are conducive to escaping macrophage uptake and prolonging the in vivo circulation time).35 Therefore, the aforementioned experimental results also evidenced that RBCs@ICG-DHN could be efficiently enriched in tumor regions through a passive tumor-targeting effect.
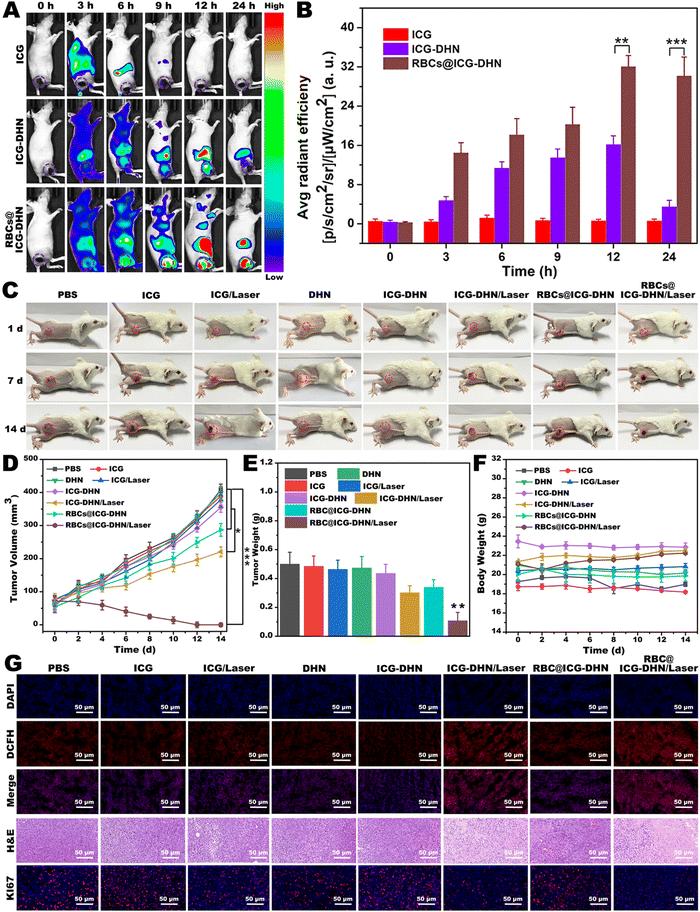 |
| Fig. 5
In vivo NIR fluorescence self-imaging-guided intracellular cascade-synthesizing chemo-drugs and efficient oncotherapy. (A) In vivo fluorescence self-imaging and (B) corresponding semi-quantitative analysis of 4T1 tumor-bearing nude mice after intravenous injection of ICG, ICG-DHN, and RBCs@ICG-DHN at the equivalent ICG concentration of 100 μg mL−1. Every test group had three nude mice. Data are presented as mean ± SD (n = 3), **p < 0.01 and ***p < 0.001. (C) Photographs of representative 4T1 tumor-bearing mice after different therapies during 14 d. Changes in (D) tumor volume, (E) tumor weight, and (F) body weight of 4T1 tumor-bearing mice treated with PBS, ICG, DHN, ICG-DHN, and RBCs@ICG-DHN with or without 808 nm laser irradiation (100 mW cm−2 and 5 min) during 14 d. (G) Representative DCFH-DA, H&E, and Ki67 staining histological images obtained from tumor tissues after different therapies. Every test group had six mice. Data are presented as mean ± SD (n = 6), *p < 0.05, **p < 0.01, and ***p < 0.001. | |
2.11.
In vivo therapeutic effect by synergistic photodynamic-chemotherapy
Motivated by the outstanding synergistic photodynamic-chemotherapy of RBCs@ICG-DHN at the cellular level, the in vivo therapeutic effect of RBCs@ICG-DHN was further evaluated. After the tumor enrichment of RBCs@ICG-DHN at the maximum peak value at 12 h post-injection, 808 nm laser irradiation was applied at the tumor regions. In addition, the tumor volume of 4T1 tumor-bearing mice was investigated to evaluate the therapeutic effect every 2 days. As shown in Fig. 5C–E, during the whole therapy, the tumor tissues of the mice in PBS, ICG, ICG with 808 nm laser irradiation, DHN, ICG-DHN, and RBCs@ICG-DHN groups continuously grew for 14 d. In contrast, the tumor tissues of the mice in the ICG-DHN with 808 nm laser irradiation group were slightly inhibited. It was also found that the tumor tissues of the mice in the RBCs@ICG-DHN with 808 nm laser irradiation group were eliminated. Meanwhile, as depicted in Fig. S9 (ESI†), the associated excised tumor images after therapy exhibited a similar antitumor tendency. Further, to evaluate the histopathological changes, DCFH-DA, hematoxylin and eosin (H&E), and Ki67 staining of the tumor tissues were also conducted. The highest intratumoral ROS abundance, most serious necrosis, and lowest Ki 67 antigen expression were shown in the tumor tissues of mice in the RBCs@ICG-DHN with 808 nm laser irradiation group (Fig. 5F).18 These findings evidenced the outstanding therapeutic effect of RBCs@ICG-DHN with 808 nm laser irradiation.
Next, the body weight was also evaluated every 2 days, and no significant weight loss could be found in all the groups during therapy (Fig. 5G). In addition, no obvious histopathological abnormalities could be found in the main organs (including the heart, liver, spleen, lung, and kidney) of the mice after therapy (Fig. S10, ESI†). It is worth mentioning that the hemolysis rate of RBCs@ICG-DHN was only 1.59%, lower than the international standard value of 5% (Fig. S11, ESI†). Overall, the aforementioned experimental phenomena jointly revealed that RBCs@ICG-DHN had an outstanding therapeutic effect, which was due to the fact that RBCs@ICG-DHN could achieve intracellular cascade-synthesizing chemo-drugs by a photooxidation process and a synergistic therapeutic effect via photodynamic-chemotherapy, thereby weakening the detrimental side effects on normal cells or tissues.
3. Conclusions
We have successfully constructed the RBC-cloaked biomimetic NIR laser/pH-responsive bioengineered nanotheranostics (RBCs@ICG-DHN) were obtained by wrapping RBCs on the surface of vehicle-free nanotheranostics (ICG-DHN), which were constructed by the hydrogen bond-driven supramolecular self-assembly between ICG and DHN. The in vitro and in vivo NIR fluorescence self-imaging results jointly evidenced that RBCs@ICG-DHN could be efficiently delivered to tumor regions and then internalized into tumor cells due to the excellent pharmacokinetics of the nanostructure and the stealth effect of the RBCs. Once stimulated with NIR laser irradiation and acidic lysosomes, RBCs@ICG-DHN could achieve a rapid disassembly and on-demand drug release. Moreover, the in vitro and in vivo results also revealed that the released ICG could efficiently convert 3O2 into 1O2. Afterward, the generated 1O2 could efficiently oxidize the released nontoxic DHN into the highly toxic chemo-drug juglone, thereby achieving intracellular cascade-synthesizing chemo-drugs and synergistic photodynamic-chemotherapy while weakening the detrimental side effects on normal cells or tissues. In summary, our vehicle-free dual-responsive nanotheranostics self-assembled via small molecules followed by a biomimetic bioengineered strategy can provide a unique idea for intracellular chemo-drug synthesis–based oncotherapy.
Ethical statement
All animals were treated according to the guidelines and policies approved by the Institutional Animal Care and Use Committee of Xiamen University.
Author contributions
Xin Zhang: methodology, writing – original draft, data curation. Xinglin Zhu: methodology, data curation, software. Yuan He: methodology, data curation, software; Ying Zhan: formal analysis, visualization, software. Shan Huang: data curation, software; Xue Yi: formal analysis; Ying Li: software, funding acquisition; Zhenqing Hou: resources, supervision, funding acquisition; Zhongxiong Fan: conceptualization, supervision, writing – review & editing.
Conflicts of interest
The authors declare that they have no known competing financial interests or personal relationships that could have appeared to influence the work reported in this paper.
Acknowledgements
We acknowledge the financial supports from the National Natural Science Foundation of China (No. 82001941), Natural Science Foundation of Fujian, China (No. 2021J01344), Provincial Department of Education of Fujian, China (No. JAT200711), Science and Technology Project of Xiamen, China (No. 3502Z02214ZD1326), the Project of Institute of Respiratory Diseases, Xiamen Medical College (No. HXJB-03), and Science Foundation of Xiamen Medical College (No. K2021-03).
References
- H. Xiang, C. You, W. Liu, D. Wang, Y. Chen and C. Dong, Biomaterials, 2021, 277, 121071 CrossRef CAS PubMed.
- D. V. Hingorani, M. M. Allevato, M. F. Camargo, J. Lesperance, M. A. Quraishi, J. Aguilera, I. Franiak-Pietryga, D. J. Scanderbeg, Z. Wang, A. A. Molinolo, D. Alvarado, A. B. Sharabi, J. D. Bui, E. E. W. Cohen, S. R. Adams, J. S. Gutkind and S. J. Advani, Nat. Commun., 2022, 13, 3869 CrossRef CAS.
- L.-H. Liu and X.-Z. Zhang, Prog. Mater. Sci., 2022, 125, 100919 CrossRef CAS.
- Y. Yi, M. Yu, C. Feng, H. Hao, W. Zeng, C. Lin, H. Chen, F. Lv, D. Zhu, X. Ji, L. Mei, M. Wu and W. Tao, Matter, 2022, 5, 2285–2305 CrossRef CAS.
- M. Shu, J. Tang, L. Chen, Q. Zeng, C. Li, S. Xiao, Z. Jiang and J. Liu, Biomaterials, 2021, 268, 120574 CrossRef CAS.
- T. Luan, L. Cheng, J. Cheng, X. Zhang, Y. Cao, X. Zhang, H. Cui and G. Zhao, ACS Appl. Mater. Interfaces, 2019, 11, 25654–25663 CrossRef CAS PubMed.
- H. He, L. Du, H. Xue, J. Wu and X. Shuai, Acta Biomater., 2022, 149, 297–306 CrossRef CAS PubMed.
- S. He, S. Lu, S. Liu, T. Li, J. Li, S. Sun, M. Liu, K. Liang, X. Fu, F. Chen, G. Meng, L. Zhang, J. Hai and B. Wang, Chem. Sci., 2020, 11, 8817–8827 RSC.
- L. Yang, Y. Zhu, L. Liang, C. Wang, X. Ning and X. Feng, Nano Lett., 2022, 22, 4207–4214 CrossRef CAS.
- S. Shen, Y. Wu, K. Li, Y. Wang, J. Wu, Y. Zeng and D. Wu, Biomaterials, 2018, 154, 197–212 CrossRef CAS PubMed.
- C. Cao, N. Yang, Y. Su, Z. Zhang, C. Wang, X. Song, P. Chen, W. Wang and X. Dong, Adv. Mater., 2022, 34, 2203236 CrossRef CAS.
- B. Chu, Y. Qu, X. He, Y. Hao, C. Yang, Y. Yang, D. Hu, F. Wang and Z. Qian, Adv. Funct. Mater., 2020, 30, 2005918 CrossRef CAS.
- Y. Zhou, W. Wu, P. Yang, D. Mao and B. Liu, Biomaterials, 2022, 288, 121693 CrossRef CAS PubMed.
- Y. Chen, Y. Li, J. Liu, Q. Zhu, J. Ma and X. Zhu, J. Controlled Release, 2021, 335, 345–358 CrossRef CAS PubMed.
- Z. Fan, D. Shi, W. Zuo, J. Feng, D. Ge, G. Su, L. Yang and Z. Hou, ACS Appl. Mater. Interfaces, 2022, 14, 5033–5052 CrossRef CAS.
- Y. Li, J. Lin, P. Wang, Q. Luo, H. Lin, Y. Zhang, Z. Hou, J. Liu and X. Liu, ACS Nano, 2019, 13, 12912–12928 CrossRef CAS.
- Y. Cheng, Y. Ji and J. Tong, J. Controlled Release, 2020, 327, 35–49 CrossRef CAS.
- J. Lin, Y. Li, P. Wang, M. Wu, F. Zhu, Y. Zhang, Z. Hou, J. Liu and X. Liu, Adv. Sci., 2022, 9, 2103498 CrossRef CAS PubMed.
- L. Huang, S. Zhao, F. Fang, T. Xu, M. Lan and J. Zhang, Biomaterials, 2021, 268, 120557 CrossRef CAS.
- L. Yang, B. Huang, S. Hu, Y. An, J. Sheng, Y. Li, Y. Wang and N. Gu, Nano Res., 2022, 15, 4285–4293 CrossRef CAS.
- H. Mei, S. Cai, D. Huang, H. Gao, J. Cao and B. He, Bioact. Mater., 2022, 8, 220–240 CrossRef CAS PubMed.
- J. Shao, L. Feng, Q. Zhao, C. Chen, J. Li, Q. Ma, X. Jiang, Y. Sun, Y. Yang, H. Gu, Y. Hu and D. Xia, J. Controlled Release, 2022, 341, 261–271 CrossRef CAS.
- H.-T. Feng, Y. Li, X. Duan, X. Wang, C. Qi, J. W. Y. Lam, D. Ding and B. Z. Tang, J. Am. Chem. Soc., 2020, 142, 15966–15974 CrossRef CAS PubMed.
- Z. Fan, D. Shi, W. Zuo, J. Feng, D. Ge, G. Su, L. Yang and Z. Hou, ACS Appl. Mater. Interfaces, 2022, 14, 5033–5052 CrossRef CAS.
- Z. M. Zhou, J. Xu, X. Q. Liu, X. M. Li, S. Y. Li, K. Yang, X. F. Wang, M. Liu and Q. Q. Zhang, Polymer, 2009, 50, 3841–3850 CrossRef CAS.
- M. Gao, C. Liang, X. Song, Q. Chen, Q. Jin, C. Wang and Z. Liu, Adv. Mater., 2017, 29, 1701429 CrossRef PubMed.
- Q. Xia, Y. Zhang, Z. Li, X. Hou and N. Feng, Acta Pharm. Sin. B, 2019, 9, 675–689 CrossRef PubMed.
- Q. Jiang, Y. Liu, R. Guo, X. Yao, S. Sung, Z. Pang and W. Yang, Biomaterials, 2019, 192, 292–308 CrossRef CAS PubMed.
- W. Shan, R. Chen, Q. Zhang, J. Zhao, B. Chen, X. Zhou, S. Ye, S. Bi, L. Nie and L. Ren, Adv. Mater., 2018, 30, 1707567 CrossRef.
- S. Ye, F. Wang, Z. Fan, Q. Zhu, H. Tian, Y. Zhang, B. Jiang, Z. Hou, Y. Li and G. Su, ACS Appl. Mater. Interfaces, 2019, 11, 15262–15275 CrossRef CAS PubMed.
- P. Zhang, J. Wang, H. Chen, L. Zhao, B. Chen, C. Chu, H. Liu, Z. Qin, J. Liu, Y. Tan, X. Chen and G. Liu, J. Am. Chem. Soc., 2018, 140, 14980–14989 CrossRef PubMed.
- Y. Zhang, H. Cheng, H. Chen, P. Xu, E. Ren, Y. Jiang, D. Li, X. Gao, Y. Zheng, P. He, H. Lin, B. Chen, G. Lin, A. Chen, C. Chu, J. Mao and G. Liu, Eur. J. Nucl. Med. Mol. Imaging, 2022, 49, 2605–2617 CrossRef CAS PubMed.
- C. Fu, R. Wei, P. Xu, S. Luo, C. Zhang, R. K. Kankala, S. Wang, X. Jiang, X. Wei, L. Zhang, A. Chen and R. Yang, Chem. Eng. J., 2021, 407, 127108 CrossRef CAS.
- J. J. Xiang, J. Liu, X. Liu, Q. Zhou, Z. H. Zhao, Y. Piao, S. Q. Shao, Z. X. Zhou, J. B. Tang and Y. Q. Shen, J. Controlled Release, 2022, 348, 444–455 CrossRef CAS.
- J. Xiong, M. Wu, J. Chen, Y. Liu, Y. Chen, G. Fan, Y. Liu, J. Cheng, Z. Wang, S. Wang, Y. Liu and W. Zhang, ACS Nano, 2021, 15, 19756–19770 CrossRef CAS.
- J. Shi, W. Nie, X. Zhao, X. Yang, H. Cheng, T. Zhou, Y. Zhang, K. Zhang and J. Liu, Adv. Mater., 2022, 34, 2201049 CrossRef CAS.
- H. Heng, G. Song, X. Cai, J. Sun, K. Du, X. Zhang, X. Wang, F. Feng and S. Wang, Angew. Chem., Int. Ed., 2022, 61, e202203444 CrossRef CAS PubMed.
- Z. Fan, T. Ren, Y. Wang, H. Jin, D. Shi, X. Tan, D. Ge, Z. Hou, X. Jin and L. Yang, Biomaterials, 2022, 283, 121452 CrossRef CAS PubMed.
- H. Lin, Y. Zhou, J. Wang, H. Wang, T. Yao, H. Chen, H. Zheng, Y. Zhang, E. Ren, L. Jiang, C. Chu, X. Chen, J. Mao, F. Wang and G. Liu, Sci. Adv., 2021, 7, eabl5862 CrossRef CAS PubMed.
- W.-L. Pan, Y. Tan, W. Meng, N.-H. Huang, Y.-B. Zhao, Z.-Q. Yu, Z. Huang, W.-H. Zhang, B. Sun and J.-X. Chen, Biomaterials, 2022, 283, 121449 CrossRef CAS.
- J. Chen, L. Chen, F. Zeng and S. Wu, Anal. Chem., 2022, 94, 8449–8457 CrossRef CAS PubMed.
Footnotes |
† Electronic supplementary information (ESI) available: Experimental section of construction of RBCs@ICG-DHN and additional data for RBCs@ICG-DHN including figure S1–S11. See DOI: https://doi.org/10.1039/d2tb01943d |
‡ X. Zhang, X. Zhu, and Y. He contributed equally to this work. |
|
This journal is © The Royal Society of Chemistry 2023 |
Click here to see how this site uses Cookies. View our privacy policy here.