Effects of sponge-derived polybrominated diphenyl ethers on human cancer cell α-N-acetylgalactosaminidase and bacterial α-D-galactosidase and their antioxidant activity†
Received
1st November 2022
, Accepted 19th December 2022
First published on 20th December 2022
Abstract
During the search for glycosidase inhibitors among marine natural products that are environmental contaminants, we evaluated for the first time the potency of polybrominated diphenyl ethers 1–7 (5: OH-BDE47, 6: OH-BDE85, 7: OH-BDE137) isolated from Dysidea sponges on the hydrolyzing activity of cancer α-N-acetylgalactosaminidase (α-NaGalase) and the potency of dihydroxylated PBDEs 1 and 2 on the hydrolyzing activity of the recombinant α-D-galactosidase (α-PsGal) from the marine bacterium Pseudoalteromonas sp. KMM 701. The antioxidant activity of the spongean metabolites was assessed to test whether the antioxidant activity affects the activity of the enzymes under study. All OH-PBDEs/MeO-PBDEs tested had no effects on human cancer cell α-N-acetylgalactosaminidase. Dihydroxylated PBDEs 1 and 2 at micromolar levels quickly and irreversibly inhibited the bacterial α-D-galactosidase. Compounds 1–7 were shown to have low antioxidant activity at millimolar levels. It is likely that antioxidant activity is not responsible for the effectiveness of α-D-galactosidase inhibition due to the large difference in the concentrations required to display antioxidant activity (millimolar) and to inhibit an enzyme (micromolar).
Environmental significance
PBDEs are under continuous research as naturally produced metabolites and synthetically produced brominated flame retardants. Both groups of PBDEs are environmental contaminants. One of the most abundant natural sources of PBDEs with various degrees of hydroxylation, methoxylation, and bromination is marine Dysidea sponges. Spongean PBDEs can be accumulated in the marine food web and transferred to humans. Enzymes glycoside hydrolases, such as α-D-galactosidase and α-N-acetylgalactosaminidase, may be one of the targets of OH-PBDEs effects on humans. Our results demonstrate that OH-PBDEs at micromolar levels irreversibly inhibit bacterial α-D-galactosidase of the GH36 family but have no effects on human cancer cell α-N-acetylgalactosaminidase.
|
Introduction
PBDEs are under continuous research as naturally produced metabolites and synthetically produced polybrominated flame retardants.1 Synthetically produced PBDEs are widespread environmental contaminants, which are transformed by biological and nonbiological ways, and are distributed in soil, groundwater, and atmosphere.2–5 PBDEs, together with their hydroxylated and methoxylated analogues, have been identified ubiquitously in humans, wildlife, and marine biota.6–8
Toxicology reports indicate that PBDEs cause thyroid dysfunction,9,10 affect ovarian granulosa cell function and follicular development,11 and interfere with the male reproductive system.12 Moreover, they have neurotoxic,13 carcinogenic14 and other toxic effects on reproductive health,15 including the effects on reproductive outcomes among women undergoing assisted reproductive technologies16 and declining semen quality.12
Marine organisms, in particular marine sponges, are an inexhaustible source of metabolites with various biological activities.17 Naturally produced PBDEs with various degrees of hydroxylation, methoxylation, and bromination have been mainly isolated with high yields from tropical marine sponges of the Dysideidae family.18–22 Spongean PBDEs possess antimicrobial,19,23 antifungal,24 and antiviral25 activities, inhibit human aldose reductase26 and a range of enzymes that are implicated in tumor development, such as inosine monophosphate dehydrogenase, guanosine monophosphate synthetase,27 15-lipoxygenase,28 and Tie2 kinase.29 They inhibit the assembly of microtubuline proteins,30 regulate interleukin-8 production,31 and exhibit anti-proliferative activity against cancer cells adapted to glucose starvation.32 Spongean PBDEs activate KCNQ potassium channels, which predominantly express in the heart and cardiomyocytes.33 Screening of 300 naturally occurring compounds showed 4,6-dibromo-2-(2′,4′-dibromophenoxy)phenol and 4,5,6-tribromo-2-(2′,4′-dibromophenoxy)phenol as substances with the potential for anticancer therapy.34 Spongean PBDEs are bioaccumulated in the marine food web with the potential transfer to humans through seafood consumption; dietary ingestion is understood to be an important uptake pathway for many OH-/MeO-PBDEs.35–38 Due to the consumption of seafood containing spongean OH-PBDEs,35 these compounds can get into humans and affect a range of enzymes, among which are glycoside hydrolases, such as α-D-galactosidase (α-Galase) and α-N-acetylgalactosaminidase (α-NaGalase).
α-D-Galactosidases catalyze the hydrolysis of the non-reducing terminal α-D-galactose from α-D-galactosides, galactooligosaccharides, and polysaccharides, such as galactomannans, galactolipids, and glycoproteins. It is known that α-galactosidase activity does not exist in the human intestine mucosa, and non-digestible α-galactosides are exclusively hydrolyzed by GH36 family α-galactosidases of the human intestinal symbiotic bacteria.39,40 So, α-Galase of the human intestinal symbiotic bacteria may be one of the targets of harmful effects of OH-PBDEs. Human α-N-acetylgalactosaminidase (α-NaGalase) is a lysosomal glycohydrolase, which hydrolyzes the O-glycosidic linkage between α-N-acetylgalactosamine and glycoproteins. α-NaGalase is produced by all cancer cells and accumulates in the blood plasma of cancer patients, leading to immunosuppression in patients with advanced cancer.41 The level of enzyme activity increases in the blood serum at the initial stage of the disease and the stage of metastasis.42 Thus, α-NaGalase, an immunosuppressive agent in cancer patients, is considered as a potential therapeutic agent in cancer treatment. One of the causes of some diseases, including cancer, viral infections, and metabolic and genetic disorders, is an imbalance in glycosidase functions;43–46 glycosidase inhibitors can regulate or block the enzyme imbalance. So, much attention is paid to identifying potential inhibitors of α-NaGalase47,48 and α-Galase.49,50
In the last years, marine organisms have received increased attention as a source of enzyme inhibitors due to their potential use in pharmacology.51,52 To date, few attempts have been made to find inhibitors of glycoside hydrolases, such as α-Galase and α-NaGalase, among low molecular marine natural products. The previously characterized low molecular marine inhibitors of α-galactosidase from the marine bacterium Pseudoalteromonas sp. (α-PsGal) are polyhydroxynaphthoquinone pigments from sea urchins and their synthetic analogues,53 polybrominated diphenyl ether and dibenzo-p-dioxins from Dysidea sponges,54 pentacyclic guanidine alkaloids from the sponge Monanchora pulchra,55 and marine alkaloids of aaptamine and makaluvamine series.56 The effect of spongean polybrominated diphenyl ethers on the activity of cancer α-NaGalase has not been previously studied.
The main goal of this study was to evaluate in vitro spongean metabolites 1–7 (Fig. 1) isolated from Dysidea sponges, some of which are environmental contaminants (5: OH-BDE47, 6: OH-BDE85, 7: OH-BDE137), on the hydrolyzing activity of α-NaGalase from cancer cells; additionally, to evaluate the effect of spongean metabolites 1 and 2 that had not been previously studied on the hydrolyzing activity of α-PsGal of the GH36 family from the marine bacterium Pseudoalteromonas sp. KMM 701.57 This enzyme can be used as a suitable model for studying the potentially harmful effects of spongean OH-PBDEs. As polybrominated diphenyl ethers can potentially be reductive inhibitors of α-PsGal and α-NaGalase due to their phenolic moieties, we evaluated their antioxidant activity to check whether the antioxidant potency influences the activity of enzymes under research.
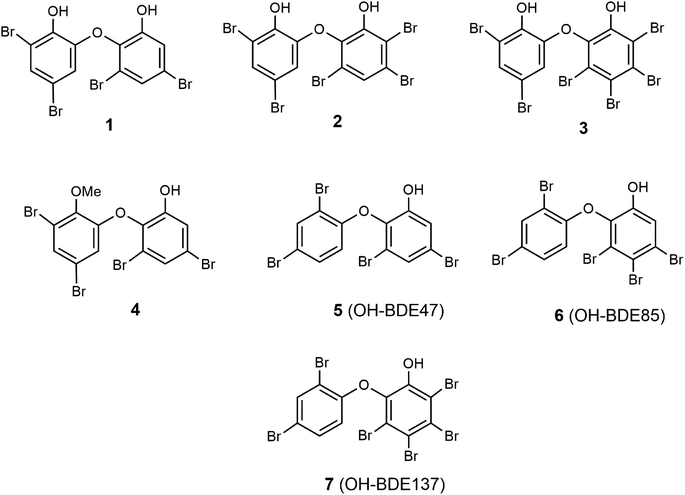 |
| Fig. 1 Structures of spongean PBDEs 1–7. | |
Materials and methods
Chemicals
Roswell Park Memorial Institute Medium (RPMI 1640), phosphate buffered saline (PBS), L-glutamine, penicillin–streptomycin solution, trypsine and fetal bovine serum (FBS), sodium hydrocarbonate (NaHCO3), and agar were purchased from BioloT (Bolshoy Sampsonievsky Ave, St. Petersburg, Russia), p-nitrophenyl-N-acetyl-α-D-galactosaminide (p-NPNA-α-Gal), p-nitrophenyl-α-D-galactopyranoside (pNP-α-Gal), bovine serum albumin (BSA), and Bradford reagent were purchased from Sigma-Aldrich (St. Louis, MO, USA); IMAC Ni2+Sepharose, Q-Sepharose, Mono-Q, and Superdex-200 PG were purchased from GE Healthcare (Uppsala, Sweden); pET 40 b(+) plasmid was purchased from Invitrogen, Carlsbad, CA, USA; recombinant protein markers SDS-PAGE-electrophoresis – from BioRad (1000 Alfred Nobel Drive, Hercules, CA, USA). Silica gel (Sorbpolymer, Krasnodar, Russia), Sephadex LH-20 (Pharmacia Fine Chemicals) were used for column chromatography, Sorbfil plates coated with silica gel F254 (Sorbpolymer, Krasnodar, Russia) were used for TLC; linoleic acid, 99% (Sigma-Aldrich) and 2,2′-azobis(2-amidinopropane) dihydrochloride (AAPH) were used for assessing antioxidant activity.
Equipment
Microplate spectrophotometer (BioTek Instruments, Highland Park, Winooski, VT, USA) was used for measuring the optical density at 400 nm (D400). Ultrasonic homogenizer Bandelin Sonopuls (Bandelin electronic GmbH & Co., KG Heinrichstraße 3–4 12, 207, Berlin, Germany) was used for homogenization. GenBAflex-tubes 6–8 kDa (Scienova GmbH, Wildenbruchstabe 15 07745 Jena, Germany) were used for dialysis. 1H NMR and 13C NMR spectra (δ in ppm, referenced to the solvent used) were recorded on a Bruker AVANCE DRX-500 NMR spectrometer at 500 and 125 MHz, respectively. High-resolution electron ionization mass spectrometry (HREI MS) was performed on an AMD-604 S mass spectrometer (Intectra, Germany). Melting points were determined on a Boetius apparatus.
Animal material
We used a freeze-dried sample of a sponge Dysidea sp. collected during scientific cruise of R/V “Academik Oparin”, which is kept in the collection of Pacific Institute of Bioorganic Chemistry.
Extraction, isolation, and identification
Reisolation of compounds 3–7 from repository samples of Dysidea sponge was described earlier.54 Data for compounds 3–7 are given in ESI (Tables S1 and S2†). A freeze-dried sponge sample Dysidea sp. (6 g) was extracted with CHCl3. The CHCl3 extract was concentrated under reduced pressure to yield a green gum. This material was partitioned into hexane solubles and hexane insolubles. The hexane-insoluble fraction contained a mixture of phenolic compounds. This mixture was chromatographed on a silica gel column in CHCl3–hexane (5
:
1) to get compound 1 and then in CHCl3 to obtain a mixture (1
:
2) of compounds 2 and 3. Compound 1 was purified by repeated column chromatography on a Sephadex LH-20 column in CHCl3–MeOH (1
:
1). Crystallization from CHCl3 gave pure 1 (1.8 mg, 0.03% on dry weight of the sponge) identified as 2-(3′,5′-dibromo-2′-hydroxyphenoxy)-3,5-dibromophenol.58 A mixture of compounds 2 and 3 was separated by repeated column chromatography on Sephadex LH-20 in CHCl3–MeOH (1
:
1) to get 2 and 3. Pure 2 (9.0 mg, 0.15% on dry weight of the sponge) was obtained by several recrystallizations from acetone and identified as 2-(3′,5′-dibromo-2′-hydroxyphenoxy)-3,4,6-tribromophenol.18–21
Compound 1.
Colourless needles (CHCl3); mp 181–183 °C; 1H NMR (acetone-d6), δH: 7.38 (1H, d, J = 2.1 Hz), 7.24 (1H, d, J = 2.1 Hz), 7.37 (1H, d, J = 2.1 Hz), 6.64 (1H, d, J = 2.1 Hz); 13C NMR, δC: 152.6 (C), 146.7 (C), 144.5 (C), 139.3 (C), 129.1 (CH), 126.8 (CH), 120.7 (CH), 119.6 (C), 118.6 (C), 116.3 (CH), 111.2 (C), 110.7 (C); HREI MS, m/z: 517.7698 (calcd for C12H6O379Br281Br2 517.7539).
Compound 2.
Colourless needles (acetone); mp 183–185 °C; 1H NMR (acetone-d6), δH: 7.47 (1H, s), 7.39 (1H, d, J = 2.1 Hz), 6.80 (1H, d, J = 2.1 Hz); 13C NMR, δC: 151.7 (C), 147.1 (C), 145.4 (C), 140.7 (C), 130.1 (CH), 128.1 (CH), 123.6 (C), 117.4 (C), 117.1 (CH), 115.4 (C), 112.2 (C), 111.2 (C); HREI MS, m/z: 595.6091, 597.6098 (calcd for C12H5O379Br381Br2 595.6110; calcd for C12H5O379Br281Br3 597.6091).
Production and purification of the recombinant α-D-galactosidase
The recombinant wild-type α-PsGal was produced as described earlier.59 The plasmid DNA pET-40b(+) containing an insertion of the gene from the marine bacterium Pseudoslteromonas sp. KMM 701 encoding α-PsGal was transformed in the Escherichia coli strain Rosetta (DE3). Heterological expression was carried out at optimal conditions as described previously.60 Purification of the recombinant α-PsGal was performed according to the procedures described previously.59,60 The purification quality was assessed by 12% Laemmli-SDS-PAGE-electrophoresis.61
Cell cultured
Cancer cells RPMI-7951 (ATCC® no. HTB-66™), MDA-MB-231 (ATCC® no. HTB-26™), DLD-1 (ATCC® no. CCL-221), HT-29 (ATCC® no. HTB-38™), HCT-116 (ATCC® no. CCL-247), SK-MEL-28 (ATCC® no. HTB-72™), and mouse healthy epidermal cells JB6 Cl 41 (ATCC® no. CRL-2010™) were obtained from the American Type Culture Collection (Manassas, VA, USA). Cells were cultured in an appropriate culture medium supplemented with 10% FBS and 1% penicillin–streptomycin solution. The cell cultures were maintained at 37 °C in a humidified atmosphere containing 5% CO2.
Preparation of cell lysate
Cell lysates were prepared according to the procedure described previously.56
Isolation and purification of α-NaGalase from cell lysates
α-NaGalase was isolated from cell lysates of melanoma RPMI-7951 and SK-Mel-28, breast cancer MDA-MB-231, intestinal carcinoma DLD-1, HT-29 and HCT-116, and healthy JB6 Cl 41 mouse epidermal cells according to the procedure described previously.62 The α-NaGalase activity was found in 75% ammonium sulphate precipitate. The purification quality was assessed by 12% Laemmli-SDS-PAGE.61
Enzyme and protein assays
Enzyme and protein assays were carried out on 96-well plates. The mixture, placed in one cell, contained 50 μL of the enzyme solution and 100 μL solution of pNP-α-Gal (1 mg mL−1 (3.3 mM) 0.05 M sodium phosphate buffer (pH 7.3)) or p-NPNA-α-Gal (3 mg mL−1 (8.8 mM) 0.05 M sodium citrate buffer (pH 5.0)) as the substrates for α-PsGal or α-NaGalase, respectively. The mixture was incubated at 20 °C or 37 °C for 10 or 60 min for α-PsGal or α-NaGalase, respectively. The reaction was stopped by adding 150 μL of 1 M Na2CO3. The amount of the released pNP was determined spectrophotometrically at 400 nm (ε400 = 18
300 M−1 cm−1). One unit of the standard activity was determined as the amount of the enzyme that releases 1 μmol of pNP per 1 min at 20 °C or 1 nmol of pNP per 1 h at 37 °C for α-PsGal or α-NaGalase, respectively. The specific activity was calculated as units per mg protein. The protein concentrations were estimated by the Bradford method with BSA as a standard.63 Buffer solutions of the α-PsGal (0.01 units per mL stock solution) and α-NaGalase (0.001 units per mL stock solution) were used in further experiments.
Optical density was measured on a Microplate spectrophotometer at 400 nm. Results were read with a computer program Gen5 and treated with ExCel computer program. All calculations were based on reactions with the consumption of 10% of the chromogenic substrate.
Determination of inhibitory potency of PBDEs 1–7 for α-NaGalase and PBDEs 1, 2, and 3 for α-PsGal
To preliminary test the inhibitory potency of the compounds, 25 μL of the enzyme solutions of α-PsGal in 0.05 M sodium phosphate buffer (pH 7.0) were preincubated for 25 min at 20 °C with 2 μL of ethanol solution of a test compound at various concentrations (from 10−3 M to 10−7 M in a probe) to allow enzyme and inhibitor interaction. In the control sample, 2 μL of EtOH was added to 25 μL of enzyme solution. The enzyme reaction was initiated by adding 120 μL of the substrate pNP-α-Gal (3.3 mM) for α-PsGal in the respective buffer solutions. After 10 min, for α-PsGal, the reaction was stopped by adding 150 μL of 1 M Na2CO3. The amount of pNP was quantified by spectrophotometric detection at 400 nm. Results were evaluated as the percent of inhibition as described above.
The inhibitory activity was assessed according to the described method, and the enzyme α-PsGal was kept for 25 min in the presence of different concentrations of compounds, and after that, its standard activity was determined.54,55 Inhibition (%) was calculated with the formula: inhibition (%) = (1 − A/A0) ×100, where A is the standard activity of enzymes under the action of an inhibitor or other factors, A0 is the standard activity of enzymes in the absence of the influence of any factors. The concentration of inhibitors causing 50% inactivation of the enzyme (IC50) was determined by fitting the dependences of the inhibition (%) on the concentration of the inhibitors to the Hill equation nonlinear regression with the computer software Origin 8.1 (OriginLab, Northampton, Massachusetts, USA).
To study the effect of compounds 1–7 on α-NaGalase, 5 μL of the compound in ethanol was added to 25 μL of the enzyme in 0.05 M sodium citrate buffer (pH 5.0) and kept for 30 min. The reaction was initiated by adding 120 μL of the 8.8 mM substrate (p-NPNA-α-Gal) and incubating at 37 °C for 90 min. The reaction was stopped by adding 150 μL of 1 M Na2CO3. The concentration of compounds 1, 2, 3, 4, 5, 6, and 7 in the sample (mM) was 1.3; 1.1; 1.0; 1.3; 1.0; 1.2, and 1.5, respectively. The following mixtures served as controls:
(1) Excluding the effect of ethanol on the enzyme, 25 μL of the enzyme and 5 μL of ethanol were kept for 30 min, and then 120 μL of the substrate was added and incubated for 90 min;
(2) Mixtures controlling the absorption of the compound at 400 nm containing 25 μL of working buffer and 5 μL of the compound were kept for 30 min; then, 120 μL of buffer was added and incubated for 90 min;
(3) Mixtures controlling the absorbance of the substrate at 400 nm containing 25 μL of working buffer and 5 μL of ethanol were incubated for 30 min; then, 120 μL of the substrate was added and incubated for 90 min.
The irreversibility of enzyme inhibition by compounds 1 and 2
To determine the irreversibility of the α-PsGal activity inhibition by compounds 1 and 2, 15 μL of 0.625 mM EtOH solution of compounds 1 and 2 were added to 75 μL of α-PsGal solution in 0.05 M sodium phosphate buffer (pH 7.0). After 30 min of the mixture incubation, the aliquot of 30 μL was taken and 120 μL of 3.3 mM pNPG solution was added to initiate the reaction; the reaction was stopped by the addition of 150 μL of 1 M Na2CO3. The α-D-galactosidase activity was determined as described above. The remaining reaction mixture was dialyzed against 1 L of 0.02 M sodium phosphate buffer (pH 7.0) for 60 h at 4 °C. GenBAflex-tubes 6–8 kDa (Scienova 108 GmbH, Wildenbruchstabe 15 07745 Jena, Germany) were used for dialysis. The buffer was changed 3 times during the dialysis. The enzyme activity was determined as described above. A sample of α-PsGal treated with H2O or EtOH in the absence of the inhibitor (75 μL of the enzyme solution and 15 μL of H2O or 6 μL of EtOH, respectively) was dialyzed and used as a control for determination of the initial activity. The experiment was carried out in two replicates. The residual activity was calculated as described above.
Determination of antioxidant activity of compounds 1–7
Linoleic acid autoxidation inhibition assay.
Antioxidant activity of compounds was determined using described methods64 with a slight modification. A straight vial (15 mm diameter; 40 mm height) containing 3.58 mL of 0.1 M phosphate buffer (pH 7.4), 20 μL of either a 20 mM EtOH solution of a test compound (0.1 mM final concentration) or 20 μL of EtOH as a control, and 200 μL of 0.1 M micelle solution of linoleic acid (5.0 mM final concentration) in 0.1 M solution of Tween-40 in 0.1 M phosphate buffer was stirred vigorously and then sonicated in a bath sonicator at 50 °C for 3 min to give a clear micelle solution. Oxidation was initiated by the addition of 200 μL of 20 mM AAPH solution in 0.1 M phosphate buffer (1.0 mM final concentration). The reaction mixture (4 mL total volume) was maintained at a constant temperature (50 °C) while continuously shaking. A 400 μL aliquot was taken at 10 min intervals from the reaction mixture and the absorbance of conjugated diene hydroperoxides at 234 nm was measured for 1 h in a 1 mm pathlength cell. The rate of conjugated diene hydroperoxide formation was calculated as follows: oxidation rate (V, μM min−1) = [(Af − A0)/(Tf − T0) × ε−1b−1] × 106, where ε is the molar extinction coefficient of the conjugated dienes at 234 nm (ε = 27
000 M−l cm−1);64b = 0.1 cm, the light path for the cell; A0 and Af, the absorbance at zero (T0) and final time (Tf) of oxidation. Inhibition (%) = 100 − (Vsample × 100/Vcontrol).
Ferric reducing antioxidant power (FRAP) assay.
The FRAP assay was adapted from previous studies.65,66 The reaction mixture (2 mL total volume) consisted of EtOH solution of tested compounds (final concentration 25, 50, 100, 200, and 300 μM), 100 μL of 0.1% solution of FeSO4·7H2O in H2O, 200 μL of 0.25% EtOH solution of α,α′-dipyridyl, and 50% aqueous EtOH to 2 mL of the total value. BHT was used as a reference compound. After 60 min, the absorbance at 520 nm was measured using a Shimadzu UV 1240 spectrophotometer. The FRAP values for compounds were calculated according to the following equation: FRAP = CFe/Cx, where CFe is the concentration of Fe2+ (μM) formed in the reaction; Cx is the concentration of tested compounds in the reacting mixture. The concentration of Fe2+ (μM) formed in the reaction was calculated using the calibration curve obtained for different concentrations of FeSO4·7H2O.
Statistical analysis
Data were analyzed using Origin 8.1 software. All of the experiments were conducted in triplicate, and the data were expressed as the mean values ± standard deviation.
Results and discussion
Characterization of the spongean OH-PBDEs
For the present study, polybrominated diphenyl ethers 2-(2′,4′-dibromophenoxy)-3,5-dibromophenol (1, yield 0.03% on the dry weight of the sponge) and 2-(2′,4′-dibromophenoxy)-3,4,5-tribromophenol (2, yield 0.15%) were reisolated from repository sample of a Dysidea sponge and identified by comparison of their spectroscopic data (1H-, 13C-NMR, HREI MS) with published values.18–21,58 Reisolation of compounds 3–7 was described previously.54
α-PsGal inhibitory potency of compounds 1 and 2
Previously, we showed that polybrominated diphenyl ethers 3–7 (Fig. 1) isolated from marine sponges of the genus Dysidea are irreversible inhibitors of recombinant α-PsGal from the marine bacterium Pseudoalteromonas sp. KMM 701.54 During that investigation, the following tendency was established. It was found that in the series of polybrominated diphenyl ethers 3–7, the presence of an additional hydroxyl group in ether 3 significantly increased its activity. An increase in the number of bromine atoms in monohydroxylated diphenyl ethers from 5 to 7 also increased their activity, which is associated with the increase of the hydrophobicity of the compounds. The presence of the methoxyl group reduced the activity of 4 (Table 1).54
Table 1 Values of IC50 for compounds 1–7 in inhibition of α-D-galactosidase (α-PsGal)
Compound |
1
|
2
|
3
|
3
|
4
|
5
|
6
|
7
|
The value of IC50 was determined in this experiment.
Published data.54
Average values of three determinations.
|
IC50, μMc |
12.88 |
8.78 |
4.48 |
4.26 |
60.5 |
41.8 |
36.0 |
16.0 |
The effect of compounds 1 and 2 on the hydrolytic activity of bacterial α-PsGal has not been investigated previously. The standard activity of α-PsGal in the presence of compounds 1 and 2 was determined according to the procedure described earlier.54 Plots of dependence of inhibition on the concentration of compounds had a sigmoid form and were approximated by the Hill equation. The theoretical dependences were fitted to the experimental values using the OriginLab 8.1 software. Values of IC50 were determined from the coefficients of non-linear regression.54 We have repeated the determination of IC50 for compound 3 in this work for comparison. It was shown that the IC50 value of compound 3 determined in this study was 4.48 μM, which is similar to the reported IC50 value of 4.26 μM (ref. 54) (ESI, Fig. S1†).
Compounds 1–3 are dihydroxylated PBDEs, compounds 5–7 are monohydroxylated PBDEs, and PBDE 4 is a methoxylated analogue of compound 1 (Fig. 1). As can be seen in Table 1, values of IC50 of the compounds for inhibition of α-PsGal decrease from compound 1 to 3, which indicates an increase in their inhibitory activity. Compounds 1, 2, and 3 have four, five, and six bromine atoms in the structure, and their hydrophobicity, calculated using the ChemOffice 2008/ChemBio3D Ultra 11.0 program, increases from 1 to 3 (cLog
P 6.15, 6.77, 7.67, respectively). These data are consistent with the previously established tendency for monohydroxylated polybrominated diphenyl ethers, and an increase in hydrophobicity leads to an increase in the inhibitory activity of hydroxylated polybrominated diphenyl ethers.54 Dependence of the activity of OH-PBDEs and the number of bromine atoms in their structures was reported earlier regarding the activity of OH-PBDEs in the inhibition of lipoxygenases26 and binding to thyroid hormone receptors.10 So, data obtained for dihydroxylated diphenyl ethers 1 and 2 confirm this tendency.
It was shown that polybrominated diphenyl ethers 1 and 2 irreversibly inactivate the α-D-galactosidase. The activity of the enzyme did not recover after dialysis against the buffer solution for 60 h, but the enzyme, in the absence of the inhibitors, kept 100% activity during this dialysis process (ESI, Table S3†).
The effect of PBDEs 1–7 on α-NaGalase
According to the modern classification of carbohydrate active enzymes (CAZy), the α-NaGalase from human cancer cells belongs to the GH27 family, and α-PsGal belongs to the GH36 family. These enzymes of the GH27 and GH36 families are related, and they share a common ancestor gene and form clan D, and their active site structure is highly homologous;46 therefore, we decided to test whether spongean PBDEs 1–7 will inhibit cancerous α-NaGalase, which is closely related. We hoped that effective α-PsGal inhibitors would inhibit the highly malignant enzyme α-NaGalase in cancer cells. All PBDEs 1–7 were tested for their influence on the hydrolyzing activity of the α-NaGalase isolated from cells of human intestinal carcinoma DLD-1, HT-29, and HCT-116, breast cancer MDA-MB-231, melanoma SK-MEL-28 and RPMI-7951, and mouse healthy epidermal cells JB6 Cl 41. Our experiments showed that none of the tested compounds 1–7 had any direct inhibitory effects on the activity not only against human cancer cell α-NaGalase as well against α-NaGalase from mouse healthy epidermal cells (ESI, Fig. S2†).
The elevated level of the enzyme α-NaGalase is a well-recognized feature of cancer cells; moreover, the ability to metastasize, impaired programmed cell death, and drug resistance are the most apparent features of cancer cells. Jafari et al.67 found that after α-NaGalase silencing in cells of human gastric adenocarcinoma, no significant difference was observed for daunorubicin sensitivity, but α-NaGalase downregulation had augmentative and regressive effects on cell death and migration. Clark et al.48 showed that the iminosugar DGJNAc can inhibit, stabilize, and chaperone human α-NaGalase both in vitro and in vivo. Ayers et al.47 showed that galacto-iteamine, unnatural analogue of alkaloid iteamine (o-aminobenzyl α-D-glucopyranoside) isolated from Itea virginica L. inflorescence, was the weak direct inhibitor of chicken liver α-NaGalase. Probably, compounds 1–7 had no inhibitory effects on cancer α-NaGalase since 1–7 are not O-glycosylated.
Antioxidant activity of compounds 1–7
Polybrominated diphenyl ethers 1–7 can potentially be reductive inhibitors of α-PsGal due to their phenolic moieties. We evaluated their antioxidant activity to check if the antioxidant activity influences the activity of α-PsGal. It has been established that under the conditions of oxidation, dihydroxylated diphenyl ethers 1–3 at a concentration of 0.1 mM exhibit weak antioxidant properties, reducing the rate of oxidation of linoleic acid by a maximum of 18.8%. An increase in the concentration of compounds 1–3 to 0.2 mM reduced the oxidation rate by 34.0–34.2% (Table 2). The standard antioxidant BHT (2,6-di-tert-butyl-4-methylphenol) at a concentration of 0.1 mM and 0.2 mM reduced the oxidation rate by 88.1% and 91.4%, respectively.
Table 2 Antioxidant activity of compounds 1–7
Compound |
AAPH-initiated oxidation of linoleic acid |
Reduction of Fe(III), μM Fe(II) per μM antioxidanta |
Rate, μM min−1 |
Inhibition, % |
0.1 mM |
0.2 mM |
0.1 mM |
0.2 mM |
Average value of three determinations.
Oxidation of linoleic acid without antioxidants.
|
1
|
7.95 |
6.45 |
18.8 |
34.2 |
0.19 |
2
|
8.01 |
6.47 |
18.2 |
34.0 |
0.18 |
3
|
7.97 |
6.46 |
18.6 |
34.1 |
0.19 |
4
|
9.78 |
9.49 |
n/a |
3.2 |
0.03 |
5
|
9.80 |
9.48 |
n/a |
3.3 |
0.03 |
6
|
9.80 |
9.47 |
n/a |
3.4 |
0.03 |
7
|
9.79 |
9.50 |
n/a |
3.1 |
0.03 |
BHT |
1.17 |
0.84 |
88.1 |
91.4 |
0.45 |
Controlb |
9.80 |
9.80 |
|
|
— |
Monohydroxylated diphenyl ethers 4–7 at a concentration of 0.1 mM did not show a noticeable inhibitory effect on the rate of linoleic acid oxidation. At a concentration of 0.2 mM, diphenyl ethers 4–7 showed a very weak antioxidant activity, reducing the oxidation rate by a maximum of 3.4% (Table 2).
In addition to the ability of compounds 1–7 to scavenge peroxyl radicals during the oxidation of linoleic acid, the iron-reducing activity of diphenyl ethers 1–7 was tested. The reduction process of Fe(III) ions was followed by an increase in the concentration of Fe(II) ions, which form a colored complex with α,α′-dipyridyl.66 The iron-reducing activity of diphenyl ethers 1–3 containing two hydroxyl groups was lower than the activity of BHT by more than 2 times. The iron-reducing activity of the monohydroxylated diphenyl ethers 4–7 was an order of magnitude lower than that of the dihydroxylated diphenyl ethers 1–3 (Table 2).
As can be seen from the data shown in Table 2, the number of bromine atoms in the molecules of brominated diphenyl ethers did not affect the rate of oxidation of linoleic acid and the iron-reducing activity. A comparison of compounds 1 and 4 shows that the replacement of one hydroxyl group in ether 1 with a methoxyl group leads to a decrease in the antioxidant activity of ether 4 (Table 2). Thus, the observed antioxidant activity of dihydroxylated diphenyl ethers 1–3 is associated with the presence of an additional hydroxyl group in their structure. As can be seen in Table 1, dihydroxylated PBDEs are more active and their α-PsGal inhibitory activity increases in the following order: 3 > 2 > 1 and the activity of less active monohydroxylated PBDEs increases in the order: 7 > 6 > 5. Although the antioxidant activity of PBDEs is low, the influence of hydroxyl groups is observed; the activity of compounds 3, 2, 1 is higher than that of 7, 6, 5. So, there is a direct correlation between the α-PsGal inhibitory and antioxidant activity of tested PBDEs.
Conclusion
Here, we characterized the properties of OH-PBDEs 1 and 2 as irreversible inhibitors of the bacterial α-D-galactosidase of GH36 family (α-PsGal) from the marine bacterium used as a suitable model. Bacterial α-D-galactosidase of GH36 family of the human intestine may be one of the targets of the harmful effects of OH-PBDEs. The study showed that OH-PBDEs from marine sponges of the genus Dysidea inhibit α-PsGal but do not act on cancer α-NaGalase.
Spongean OH-PBDEs at high concentrations exhibit weak antioxidant activity. Comparison of the results of inhibition of α-PsGal activity by compounds 1–7 and antioxidant activity allows us to draw the following conclusions: the presence of a hydroxyl group is a necessary condition for the inhibition of α-PsGal and antioxidant activity; dihydroxylated compounds are more active than monohydroxylated ones; the introduction of a methoxyl group into the molecule of PBDE reduces its inhibitory action on α-PsGal and reduces the antioxidant activity of PBDE; an increase in the degree of bromination increases the inhibitory activity of PBDEs on the enzyme, while the antioxidant activity does not depend on the number of bromine atoms in the molecule. Antioxidant activity is not responsible for the effectiveness of α-PsGal inhibition due to the large difference in the concentrations required for enzyme inhibition (μM) and antioxidant activity (mM).
Our findings add to the knowledge of OH-PBDEs as environmental toxins and their potential action on human health.
Conflicts of interest
There are no conflicts of interest to declare.
Acknowledgements
This work was supported by the Russian Foundation for Basic Research, RFBR grant number 20-04-00591. We thank Slepchenko L. for technical support in the isolation of α-D-galactosidase.
References
- L. Schmitt, I. Hinxlage, P. A. Cea, H. Gohlke and S. Wesselborg, 40 Years of Research on Polybrominated Diphenyl Ethers (PBDEs)—A Historical Overview and Newest Data of a Promising Anticancer Drug, Molecules, 2021, 26, 995, DOI:10.3390/molecules26040995.
- Y. Zhang, B. Xi and W. Tan, Release, transformation, and risk factors of polybrominated diphenyl ethers from landfills to the surrounding environments: A review, Environ. Int., 2021, 157, 106780, DOI:10.1016/j.envint.2021.106780.
- C. R. Ohoro, A. O. Adeniji, A. I. Okoh and O. O. Okoh, Polybrominated diphenyl ethers in the environmental systems: a review, J. Environ. Health Sci. Eng., 2021, 19(1), 1229–1247, DOI:10.1007/s40201-021-00656-3.
- T. Wu, Y. Li, H. Xiao and M. Fu, Molecular Modifications and Control of Processes to Facilitate the Synergistic Degradation of Polybrominated Diphenyl Ethers in Soil by Plants and Microorganisms Based on Queuing Scoring Method, Molecules, 2021, 26(13), 3911, DOI:10.3390/molecules26133911.
- D. Lindqvist and J. Gustafsson, Degradation of naturally produced hydroxylated polybrominated diphenyl ethers in Baltic Sea sediment via reductive debromination, Environ. Sci. Pollut. Res. Int., 2021, 28(20), 25878–25885, DOI:10.1007/s11356-021-12462-3.
- L. Belova, Y. Fujii, P. Cleys, M. Śmiełowska, K. Haraguchi and A. Covaci, Identification of novel halogenated naturally occurring compounds in marine biota by high-resolution mass spectrometry and combined screening approaches, Environ. Pollut., 2021, 289, 117933, DOI:10.1016/j.envpol.2021.117933.
- Q. T. Ho, M. S. Bank, A. M. Azad, B. M. Nilsen, S. Frantzen, S. Boitsov, A. Maage, T. Kögel, M. Sanden, L. Frøyland, R. Hannisdal, H. Hove, A. K. Lundebye, O. J. Nøstbakken and L. Madsen, Co-occurrence of contaminants in marine fish from the North East Atlantic Ocean: Implications for human risk assessment, Environ. Int., 2021, 157, 106858, DOI:10.1016/j.envint.2021.106858.
- B. Xu, M. Wu, M. Wang, C. Pan, W. Qiu, L. Tang and X. Gang, Polybrominated diphenyl ethers (PBDEs) and hydroxylated PBDEs in human serum from Shanghai, China: a study on their presence and correlations, Environ. Sci. Pollut. Res., 2018, 25(4), 3518–3526, DOI:10.1007/s11356-017-0709-4.
- Q. He, X. Chen, J. Liu, C. Li, H. Xing, Y. Shi and Q. Tang, Combining Network Pharmacology with Molecular Docking for Mechanistic Research on Thyroid Dysfunction Caused by Polybrominated Diphenyl Ethers and their Metabolites, BioMed Res. Int., 2021, 2961747, DOI:10.1155/2021/2961747.
- X. M. Ren, L. H. Guo, Y. Gao, B. T. Zhang and B. Wan, Hydroxylated polybrominated diphenyl ethers exhibit different activities on thyroid hormone receptors depending on their degree of bromination, Toxicol. Appl. Pharmacol., 2013, 268(3), 256–263, DOI:10.1016/j.taap.2013.01.026.
- P. L. C. Lefèvre, T. C. Nardelli, W. Y. Son, A. R. Sadler, D. F. K. Rawn, C. Goodyer, B. Robaire and B. F. Hales, Polybrominated Diphenyl Ethers in Human Follicular Fluid Dysregulate Mural and Cumulus Granulosa Cell Gene Expression, Endocrinology, 2021, 162(3), bqab003, DOI:10.1210/endocr/bqab003.
- S. Ermler and A. Kortenkamp, Declining semen quality and polybrominated diphenyl ethers (PBDEs): Review of the literature to support the derivation of a reference dose for a mixture risk assessment, Int. J. Hyg. Environ. Health, 2022, 242, 113953, DOI:10.1016/j.ijheh.
- B. Qian, Z. Zen, Z. Zheng, C. Wang and J. Song, A preliminary study on the mechanism of the neurosteroid-mediated ionotropic receptor dysfunction in neurodevelopmental toxicity induced by decabromodiphenyl ether, Ecotoxicol. Environ. Saf., 2021, 217, 112198, DOI:10.1016/j.ecoenv.2021.112198.
- B. L. Qu, W. Yu, Y. R. Huang, B. N. Cai, L. H. Du and F. Liu, 6-OH-BDE-47 promotes human lung cancer cells epithelial mesenchymal transition via the AKT/Snail signal pathway, Environ. Toxicol. Pharmacol., 2015, 39(1), 271–279, DOI:10.1016/j.etap.2014.11.022.
- A. D. Mutic, D. B. Barr, V. S. Hertzberg, P. A. Brennan, A. L. Dunlop and L. A. McCauley, Polybrominated Diphenyl Ether Serum Concentrations and Depressive Symptomatology in Pregnant African American Women, Int. J. Environ. Res. Public Health, 2021, 18, 3614, DOI:10.3390/ijerph18073614.
- R. D. Björvang, I. Hallberg, A. Pikki, L. Berglund, M. Pedrelli, H. Kiviranta, P. Rantakokko, P. Ruokojärvi, C. H. Lindh, M. Olovsson, S. Persson, J. Holte, Y. Sjunnesson and P. Damdimopoulou, Follicular fluid and blood levels of persistent organic pollutants and reproductive outcomes among women undergoing assisted reproductive technologies, Environ. Res., 2022, 208, 112626, DOI:10.1016/j.envres.2021.112626.
- A. R. Carroll, B. R. Copp, R. A. Davis, R. A. Keyzers and M. R. Prinsep, Marine natural products, Nat. Prod. Rep., 2021, 38(2), 362–413, 10.1039/D0NP00089B.
- N. Hanif, T. A. Tyas, L. Hidayati, F. F. Dinelsa, D. Provita, N. R. Kinnary, F. M. Prasetiawan, G. A. Khalik, Z. Mubarok, D. Tohir, A. Setiawan, M. Farid, V. Kurnianda, A. Murni, N. J. de Voogd and J. Tanaka, Oxy-Polybrominated Diphenyl Ethers from the Indonesian Marine Sponge, Lamellodysidea herbacea: X-ray, SAR, and Computational Studies, Molecules, 2021, 26(21), 6328, DOI:10.3390/molecules26216328.
- G. M. Sharma and B. Vig, Studies on the antimicrobial substances of sponges. VI. Structures of two antibacterial substances isolated from the marine sponge Dysidea herbacea, Tetrahedron Lett., 1972, 13(17), 1715–1718, DOI:10.1016/S0040-4039(01)84729-8.
- B. Carté and D. J. Faulkner, Polybrominated diphenyl ethers from Dysidea herbacea, Dysidea chlorea and, Phyllospongia foliascens, Tetrahedron, 1981, 37(13), 2335–23339, DOI:10.1016/S0040-4020(01)88886-4.
- R. S. Norton, K. D. Croft and R. J. Wells, Polybrominated oxydiphenol derivatives from the sponge Dysidea herbacea: structure determination by analysis of 13C spin-lattice relaxation data for quaternary carbons and 13C–1H coupling constants, Tetrahedron, 1981, 37(13), 2341–2349, DOI:10.1016/S0040-4020(01)88887-6.
- J. Salvá and D. J. Faulkner, A new brominated diphenyl ether from a Philippine Dysidea species, J. Nat. Prod., 1990, 53(3), 757–760, DOI:10.1021/np50069a043.
- S. Sun, C. B. Canning, K. Bhargava, X. Sun, W. Zhu, N. Zhou, Y. Zhang and K. Zhou, Polybrominated diphenyl ethers with potent and broad spectrum antimicrobial activity from the marine sponge Dysidea, Bioorg. Med. Chem. Lett., 2015, 25(10), 2181–2183, DOI:10.1016/j.bmcl.2015.03.057.
- E. Sionov, D. Roth, H. Sandovsky-Losica, Y. Kashman, A. Rudi, L. Chill, I. Berdicevsky and E. Segal, Antifungal effect and possible mode of activity of a compound from the marine sponge Dysidea herbacea, J. Infect., 2005, 50(5), 453–460, DOI:10.1016/j.jinf.2004.07.014.
- A. Yamashita, Y. Fujimoto, M. Tamaki, A. Setiawan, T. Tanaka, K. Okuyama-Dobashi, H. Kasai, K. Watashi, T. Wakita, M. Toyama, M. Baba, N. J. de Voogd, S. Maekawa, N. Enomoto, J. Tanaka and K. Moriishi, Identification of antiviral agents targeting hepatitis B virus promoter from extracts of Indonesian marine organisms by a novel cell-based screening assay, Mar. Drugs, 2015, 13(11), 6759–6773, DOI:10.3390/md13116759.
- J. A. de la Fuente, S. Manzanaro, M.
J. Martín, T. G. de Quesada, I. Reymundo, S. M. Luengo and F. Gago, Synthesis, activity, and molecular modeling studies of novel human aldose reductase inhibitors based on a marine natural product, J. Med. Chem., 2003, 46, 5208–5221, DOI:10.1021/jm030957n.
- X. Fu, F. J. Schmitz, M. Govindan, S. A. Abbas, K. M. Hanson, P. A. Horton, P. Crews, M. Laney and R. C. Schatzman, Enzyme inhibitors: new and known polybrominated phenols and diphenyl ethers from four Indo-Pacific Dysidea sponges, J. Nat. Prod., 1995, 58, 1384–1391, DOI:10.1021/np50123a008.
- E. N. Segraves, R. R. Shah, N. L. Segraves, T. A. Johnson, S. Whitman, J. K. Sui, V. A. Kenyon, R. H. Cichewicz, P. Crews and T. R. Holman, Probing the activity differences of simple and complex brominated aryl compounds against 15-soybean, 15-human, and 12-human lipoxygenase, J. Med. Chem., 2004, 47(16), 4060–4065, DOI:10.1021/jm049872s.
- Y. M. Xu, R. K. Johnson and S. M. Hecht, Polybrominated diphenyl ethers from a sponge of the Dysidea genus that inhibit Tie2 kinase, Bioorg. Med. Chem., 2005, 13(3), 657–659, DOI:10.1016/j.bmc.2004.10.061.
- H. Liu, M. Namikoshi, S. Meguro, H. Nagai, H. Kobayashi and X. Yao, Isolation and characterization of polybrominated diphenyl ethers as inhibitors of microtubule assembly from the marine sponge Phyllospongia dendyi collected at Palau, J. Nat. Prod., 2004, 67, 472–474, DOI:10.1021/np0304621.
- T. Oda, H. Liu and M. Namikoshi, Effects of polybrominated diphenol ethers from a marine sponge Phyllospongia dendyi on IL-8 production in a PMA-stimulated promyelocytic leukemia cell line, Mar. Drugs, 2005, 3, 119–125, DOI:10.3390/md304119.
- M. Arai, D. Shin, K. Kamiya, R. Ishida, A. Setiawan, N. Kotoku and M. Kobayashi, Marine spongean polybrominated diphenyl ethers, selective growth inhibitors against the cancer cells adapted to glucose starvation, inhibits mitochondrial complex II, J. Nat. Med., 2017, 71, 44–49, DOI:10.1007/s11418-016-1025-x.
- L.-X. Liu, R.-R. Gu, Y. Jin, X.-Q. Chen, X.-W. Li, Y.-M. Zheng, Z.-B. Gao and Y.-W. Guo, Diversity-oriented synthesis of marine polybrominated diphenyl ethers as potential KCNQ potassium channel activators, Bioorg. Chem., 2022, 126, 105909, DOI:10.1016/j.bioorg.2022.105909.
- S. Mayer, M. Prechtl, P. Liebfried, R.-P. Cadeddu, F. Stuhldreier, M. Kohl, F. Wenzel, B. Stork, S. Wesselborg, P. Proksch, U. Germing, R. Haas and P. Jäger, First results from a screening of 300 naturally occurring compounds: 4,6-dibromo-2-(2′,4′-dibromophenoxy)phenol, 4,5,6-tribromo-2-(2′,4′-dibromophenoxy)phenol, and 5-epi-nakijinone Q as substances with the potential for anticancer therapy, Mar. Drugs, 2019, 17, 521, DOI:10.3390/md17090521.
- Y. Liu, J. Liu, M. Yu, Q. Zhou and G. Jiang, Hydroxylated and methoxylated polybrominated diphenyl ethers in a marine food web of Chinese Bohai Sea and their human dietary exposure, Environ. Pollut., 2018, 233, 604–611, DOI:10.1016/j.envpol.2017.10.105.
- A. S. Madgett, K. Yates, L. Webster, C. McKenzie, A. Brownlow and C. F. Moffat, The concentration and biomagnification of PCBs and PBDEs across four trophic levels in a marine food web, Environ. Pollut., 2022, 309, 119752, DOI:10.1016/j.envpol.2022.119752.
- A. Li, Q. Tang, K. Kearney, K. Nagy, J. Zhang, S. Buchanan and M. E. Turyk, Persistent and toxic chemical pollutants in fish consumed by Asians in Chicago, United States, Sci. Total Environ., 2022, 811, 152214, DOI:10.1016/j.scitotenv.2021.152214.
- X. Li, M. Wang, Y. Yang, B. Lei, S. Ma and Y. Yu, Influence of nutrients on the bioaccessibility and transepithelial transport of polybrominated diphenyl ethers measured using an in vitro method and Caco-2 cell monolayers, Ecotoxicol. Environ. Saf., 2021, 208, 111569, DOI:10.1016/j.ecoenv.2020.111569.
- M. Lafond, A. S. Tauzin, L. Bruel, E. Laville, V. Lombard, J. Esque, I. André, N. Vidal, F. Pompeo, N. Quinson, J. Perrier, M. Fons, G. Potocki-Veronese and T. Giardina, α-Galactosidase and Sucrose-Kinase Relationships in a Bi-functional AgaSK Enzyme Produced by the Human Gut Symbiont Ruminococcus gnavus E1, Front. Microbiol., 2020, 11, 579521, DOI:10.3389/fmicb.2020.579521.
- Y. Sasaki, Y. Uchimura, K. Kitahara and K. Fujita, Characterization of a GH36 α-D-galactosidase associated with assimilation of gum Arabic in Bifidobacterium longum subsp. longum JCM7052, J. Appl. Glycosci, 2021, 68(2), 47–52, DOI:10.5458/jag.jag.JAG-2021_0004.
- N. Yamamoto, V. R. Naraparaju and S. O. Asbell, Deglycosylation of serum vitamin D3-binding protein leads to immunosuppression in cancer patients, Cancer Res., 1996, 56, 2827–2831, DOI:10.1006/clin.1996.4320.
- S. P. J. Albracht and J. van Pelt, Multiple exo-glycosidases in human serum as detected with the substrate DNP-α-GalNAc. II. Three α-N-acetylgalactosaminidase-like activities in the pH 5 to 8 region, BBA Clin., 2017, 8, 90–96, DOI:10.1016/j.bbacli.2017.09.002.
- N. Yamamoto and M. Urade, Pathogenic significance of α-N-acetylgalactosaminidase activity found in the hemagglutinin of influenza virus, Microbes Infect., 2005, 7, 674–681, DOI:10.1016/j.micinf.2005.01.015.
- N. Yamamoto, Pathogenic significance of α-N-acetylgalactosaminidase activity found in the envelope glycoprotein gp160 of human immunodeficiency virus Type I, AIDS Res. Hum. Retroviruses, 2006, 22, 262–271, DOI:10.1089/aid.2006.22.262.
- O. P. Van Diggelen, D. Schindler, W. J. Kleijer, J. M. G. Huijmans, H. Galjaard, H.-U. Linden, J. Peter-Katalinic, H. Egge and M. Cantz, Lysosomal alpha-NaGalase deficiency: A new inherited metabolic disease, Lancet, 1987, 2, 804, DOI:10.1016/S0140-6736(87)92542-6.
- I. Y. Bakunina, L. A. Balabanova, A. Pennacchio and A. Trincone, Hooked on α-D-galactosidases: From biomedicine to enzymatic synthesis, Crit. Rev. Biotechnol., 2016, 36, 233–245, DOI:10.3109/07388551.2014.949618.
- B. J. Ayers, J. Hollinshead, A. W. Saville, S. Nakagawa, I. Adachi, A. Kato, K. Izumori, B. Bartholomew, G. W. J. Fleet and R. J. Nash, Iteamine, the first alkaloid isolated from Itea virginica L. inflorescence, Phytochemistry, 2014, 100, 126–131, DOI:10.1016/j.phytochem.2014.01.012.
- N. E. Clark, M. C. Metcalf, D. Best, G. W. J. Fleet and S. C. Garman, Pharmacological chaperones for human α-N-acetylgalactosaminidase, Proc. Natl. Acad. Sci. U. S. A., 2012, 109, 17400–17405, DOI:10.1073/pnas.1203924109.
- P. Weber, R. Fischer, S. A. Nasseri, A. E. Stütz, M. Thonhofer, S. G. Withers, A. Wolfsgruber and T. M. Wrodnigg, New α-galactosidase-inhibiting aminohydroxycyclopentanes, RSC Adv., 2021, 11(26), 15943–15951, 10.1039/d1ra02507d.
- P. Besada, M. Gallardo-Gómez, T. Pérez-Márquez, L. Patiño-Álvarez, S. Pantano, C. Silva-López, C. Terán, A. Arévalo-Gómez, A. Ruz-Zafra, J. Fernández-Martín and S. Ortolano, The new pharmacological chaperones PBXs increase α-Galactosidase A activity in Fabry disease cellular models, Biomolecules, 2021, 11(12), 1856, DOI:10.3390/biom11121856.
- D. A. Tischler, Perspective on enzyme inhibitors from marine organisms, Mar. Drugs, 2020, 18, 431, DOI:10.3390/md18090431.
- N. Ruocco, S. Costantini, F. Palumbo and M. Costantini, Marine sponges and bacteria as challenging sources of enzyme inhibitors for pharmacological applications, Mar. Drugs, 2017, 15, 173, DOI:10.3390/md15060173.
- I. Y. Bakunina, E. A. Kol'tsova, N. D. Pokhilo, O. P. Shestak, A. Y. Yakubovskaya, T. N. Zvyagintseva and V. F. Anufriev, Effect of 5-hydroxy- and 5,8-dihydroxy-1,4-naphthoquinones on the hydrolytic activity of alpha-galactosidase, Chem. Nat. Compd., 2009, 45, 69–73, DOI:10.1007/s10600-009-9252-y.
- N. K. Utkina, G. N. Likhatskaya, L. A. Balabanova and I. Y. Bakunina, Sponge-derived polybrominated diphenyl ethers and dibenzo-p-dioxins, irreversible inhibitors of the bacterial α-D-galactosidase, Environ. Sci.: Processes Impacts, 2019, 21, 1754–1763, 10.1039/C9EM00301K.
- I. Bakunina, G. Likhatskaya, L. Slepchenko, L. Balabanova, L. Tekutyeva, O. Son, L. Shubina and T. Makarieva, Effect of pentacyclic guanidine alkaloids from the sponge Monanchora pulchra on activity of α-glycosidases from marine bacteria, Mar. Drugs, 2019, 17, 22, DOI:10.3390/md17010022.
- N. Utkina, G. Likhatskaya, O. Malyarenko, S. Ermakova, L. Balabanova, L. Slepchenko and I. Bakunina, Effects of sponge-derived alkaloids on activities of the bacterial α-D-galactosidase and human cancer cell α-N-Acetylgalactosaminidase, Biomedicines, 2021, 9, 510, DOI:10.3390/biomedicines9050510.
- L. A. Balabanova, I. Y. Bakunina, O. I. Nedashkovskaya, I. D. Makarenkova, T. S. Zaporozhets, N. N. Besednova, T. N. Zvyagintseva and V. A. Rasskazov, Molecular characterization and therapeutic potential of a marine bacterium Pseudoalteromonas sp. KMM 701 α-D-galactosidase, Mar. Biotechnol., 2010, 12, 111–120, DOI:10.1007/s10126-009-9205-2.
- N. K. Utkina and V. A. Denisenko, New polybrominated diphenyl ether from the marine sponge Dysidea herbacea, Chem. Nat. Compd., 2006, 42, 606–607, DOI:10.1007/s10600-006-0226-z.
- I. Y. Bakunina, L. A. Balabanova, V. A. Golotin, L. A. Slepchenko, V. V. Isakov and V. V. Rasskazov, Stereochemical course of hydrolytic reaction catalyzed by alpha-galactosidase from cold adaptable marine bacterium of genus Pseudoalteromonas, Front. Chem., 2014, 2, 1–6, DOI:10.3389/fchem.2014.00089.
- V. A. Golotin, L. A. Balabanova, Y. A. Noskova, L. A. Slepchenko, I. Y. Bakunina, N. S. Vorobieva, N. A. Terentieva and V. A. Rasskazov, Optimization of cold-adapted α-D-galactosidase expression in Escherichia coli, Protein Expression Purif., 2016, 123, 14–18, DOI:10.1016/j.pep.2016.03.006.
- V. K. Laemmli, Cleavage of structural proteins during of the head of bacteriophage T4, Nature, 1970, 227, 680–685, DOI:10.1038/227680a0.
- I. Y. Bakunina, O. A. Chadova, O. S. Malyarenko and S. P. Ermakova, The Effect of fucoidan from the brown alga Fucus evanescence on the activity of α-N-acetylgalactosaminidase of human colon carcinoma cells, Mar. Drugs, 2018, 16, 155, DOI:10.3390/md16050155.
- M. M. Bradford, A rapid and sensitive method for the quantitation of microgram quantities of protein utilizing the principle of protein-dye binding, Anal. Biochem., 1976, 72, 248–254, DOI:10.1016/0003-2697(76)90527-3.
- W. A. Pryor, J. A. Cornicelli, J. L. Devall, B. Tait, B. K. Trivedi, D. T. Witiak and M. Wut, A rapid screening test to determine the antioxidant potencies of natural and synthetic antioxidants, J. Org. Chem., 1993, 58, 3521–3532, DOI:10.1021/jo00065a013.
- J. F. Berkowitz, C. M. VanZomeren, S. J. Currie and L. Vasilas, Application of α,α'-dipyridyl dye for hydric soil identification, Soil Sci. Soc. Am. J., 2017, 81, 654–658, DOI:10.2136/sssaj2016.12.0431.
- P. Vassileva-Alexandrova, Détermination spectrophotométrique de l'analgène au moyen de chlorure de fer(III) et d'α,α'-dipyridyle, Mikrochim. Acta, 1970, 58, 1039–1044, DOI:10.1007/BF01225740.
- M. Jafari, N. Rahimi, M. S. Jami, M. Hashemzadeh Chaleshtori, F. Elahian and S. A. Mirzaei, Silencing of α-N-acetylgalactosaminidase in the gastric cancer cells amplified cell death and attenuated migration, while the multidrug resistance remained unchanged, Cell Biol. Int., 2022, 46(2), 255–264, DOI:10.1002/cbin.11727.
Footnote |
† Electronic supplementary information (ESI) available: Physical properties for compounds 3–7; dose–response curves of α-PsGal inhibition with compounds 1–3; the α-PsGal activity after PBDEs 1 and 2 treatment; effects of compounds 1–7 on cancer cell α-NaGalase. See DOI: https://doi.org/10.1039/d2va00269h |
|
This journal is © The Royal Society of Chemistry 2023 |
Click here to see how this site uses Cookies. View our privacy policy here.